Collagen-based materials combined with microRNA for repairing cornea wounds and inhibiting scar formation†
Received
31st August 2018
, Accepted 30th October 2018
First published on 1st November 2018
Abstract
The cornea repair materials amniotic membrane, acellular corneal stroma, and natural polymer-based materials have excellent biocompatibility and support epithelization. However, few researchers have focused on corneal stromal wound healing repair with regard to scar formation and transparency improvements. Herein, we introduced nanocomplexes of gold nanoparticles (AuNPs) and microRNA-133b (miR-133b) into collagen-based material to achieve corneal repair and scar inhibition. We evaluated the cytocompatibility of AuNPs and the ability of miRNA-133b to inhibit myofibroblast transformation in vitro. The AuNPs had no cytotoxicity at working concentrations, and AuNP/miR-133b complexes down-regulated the expression of myofibroblast transformation genes (alpha-smooth muscle actin and type I collagen) in corneal stromal cells. We then loaded AuNP/miR-133b complexes into collagen using two methods: loading on the surface (Col-AMS) and loading inside (Col-AMI) the collagen membrane. The results showed that AuNP/miR-133b introduction did not affect water content, light transmittance, or the mechanical properties of the collagen membrane. MiR-133b can maintain its integrity during the preparation of materials. In vivo lamellar keratoplasty results showed that the cornea epithelized completely and rapidly. Corneas without transplantation and with the transplantation of a non-modified collagen membrane became cloudy after trauma to different degrees after approximately 14 days. Interestingly, cornea transparency was retained after transplantation with Col-AMS and Col-AMI. Hematoxylin–eosin-stained histologic sections revealed that the corneas transplanted with Col-AMS and Col-AMI were similar to healthy corneas. Immunohistochemical staining revealed lower a-SM and Col-1 expression in corneal stroma after transplantation with collagen combined with AuNP/miR-133b. Our results thus suggest that collagen membranes combined with AuNP/miR-133b complexes can rapidly repair corneas and effectively inhibit scar formation.
1. Introduction
Transparency is one of the most important properties of the cornea, which is located on the outermost layer of the eye and is easily injured. Corneal injury or opacification can lead to visual loss and affect many people.1,2 The only widely accepted therapeutic method is treatment with conventional donor corneal grafts.3 To solve the donor insufficiency problem, researchers have developed various artificial cornea repair materials, including amniotic membrane, acellular corneal stroma, and natural polymer-based materials, such as chitosan, fibrin, and collagen.4–10 These materials have appropriate physicochemical properties and biocompatibility in vitro and in vivo. Corneal transparency can be restored within 4 weeks, and the materials cause no stimulation at the ocular surface. Epithelialization can also be completed within 4 weeks, and no obvious postoperative complications have been observed.11,12
However, corneal wound healing requires not only epithelium healing but also stromal healing. The healing of the corneal stroma is a complex physiological process that affects corneal scar formation.13,14 After corneal damage or graft transplantation, varying degrees of corneal scarring can clearly be seen in the anterior segment in optical coherence tomography images and in hematoxylin–eosin-stained sections.15,16 Unlike skin fibrotic scar formation after wound healing, corneal scarring significantly decreases transparency and affects function.17 After a scar is formed, it cannot recover through self-repair. However, even though scar formation cannot be prevented after transplantation with these materials, few researchers have focused on corneal scar inhibition. Finding a means to reduce scar formation during the corneal healing process in the presence of repair materials is a problem that needs to be solved.
MicroRNAs are short RNA sequences that regulate gene expression.18,19 Functional miRNA can combine with an Argonaute protein to form an miRNA–ribonucleoprotein complex. This can cause block translation in the case of partial complementarity.20,21 Several researchers have introduced microRNA into scaffolds for scar inhibition in skin regeneration and osteogenesis, but no previous reports have combined collagen with microRNA for corneal applications.22–24 Researchers have found that the scar formation process can be reduced through the down-regulation of alpha-smooth muscle actin (a-SM) and type I collagen (Col-1), which are up-regulated during corneal wound healing.25 MiR-133b can mediate the expression of a-SM and Col-1 in cornea stromal cells by combining with target mRNA and inhibiting the translation of a-SM and Col-1.25 However, reliable nanodelivery vectors are needed to allow microRNA to cross the cell membrane and subsequently facilitate sequence-specific mRNA degradation or translational inhibition.26,27 Gold nanoparticles (AuNPs) are promising candidates for the delivery of small molecule drugs or biomacromolecules, such as DNA, siRNA and miRNA.28,29 In other words, without using AuNPs, miR-133b will be degraded in the physiological environment and be unable to enter into cells.
In this study, we used collagen as a scaffold material and combined AuNP/miR-133b to repair corneas and inhibit the scar formation process. The physicochemical properties of the composite membrane were characterized. The effects of AuNPs on the collagen membrane and the effects of collagen on microRNA were analyzed. The inhibitory capacities of miR-133b and the composite membrane in vitro/in vivo were assayed in cell experiments and in a lamellar keratoplasty (LKP) model in New Zealand white rabbits.
2. Materials and methods
2.1 Materials
PEI-capped gold nanoparticles were prepared thorough a one-pot procedure, as previously described.30 The sense strand sequence of miRNA-133b was 5′-UUUGGUCCCCUUCAACCAGCUA-3′, and the miRNA negative control (miRNA-NC) sequence was 5′-UUCUCCGAACGUGUCACGUTT-3′ (GenePharma Co. Ltd, Guangzhou, China). Freeze-dried type I collagen (HM Biotech Ltd, Guangzhou, China) was extracted from bovine tendons. Deionized water was obtained from a water purification system (Millipore S. A. S., Molsheim, France). All cell culture related reagents were purchased from Sigma-Aldrich (St Louis, MO, USA). Male and female New Zealand white rabbits (8–10 weeks old and 1.8–2.3 kg body weight) (Guangdong Medical Laboratory Animal Center, Foshan City, Guangdong Province, China) were used as animal transplant recipients. Their use was approved by the Medicine Ethics Committee at Sun Yat-sen University, Guangzhou, China.
2.2
In vitro cell culture and AuNP/miRNA complexes
The rabbit corneal stromal cells (Rochen Pharma Co., Ltd) were extracted from fresh New Zealand rabbit cornea and cultured in high glucose Dulbecco's modified Eagle's medium (Gibco BRL, Waltham, MA, USA) with 10% fetal bovine serum (Gibco), 100 U mL−1 penicillin and 100 μg mL−1 streptomycin (Gibco). Cells were grown to confluency in 25 cm2 polystyrene tissue culture flasks at 37 °C in 5% CO2 and 95% air, and confluent cells were subcultured every 2–3 days via trypsinization with trypsin/EDTA solution. Cells from early passages (≤6) were used in all experiments.
MiR-133b (1 μL of 20 μM, to obtain 1 pmol) was mixed with poly(ethyleneimine) (PEI)-capped AuNP solution at different volume ratios. The ability of the PEI-capped AuNPs to bind miR-133b was assessed through 1% agarose gel electrophoresis after incubation at room temperature for 15 min.
The integrity and resistance to degradation of naked miR-133b, compared with its integrity in AuNP/miR-133b complexes, were tested using RNase and 15% polyacrylamide gel electrophoresis. Naked miR-133b in phosphate-buffered saline (PBS) or in a complex with AuNPs were mixed with RNase solution (100 μg mL−1) at a volume ratio of 1
:
1. Then the mixtures were incubated at 37 °C. After incubation for a determined time, the integrities of naked miR-133b as well as miRNA in AuNP/miR-133b complexes were assessed via gel electrophoresis. Then the gel was stained with Gelred (Beijing Solarbio Science & Technology Co., Ltd, China) to visualize the integrity of the miRNA. Complexes of miR-133b with AuNPs were added to cells to a final miRNA concentration of 50 nM. The w/w ratio between AuNP and miR-133b was 3. The morphology of the PEI-capped AuNPs was examined using transmission electron microscopy (TEM; JEM-2100HR, Japan).
2.3
In vitro biocompatibility and wound healing assays
Rabbit corneal stromal cells were seeded in 96-well tissue culture plates (BD Biosciences) at 5000 cells per cm2. The culture medium contained different concentrations of AuNPs (experimental group, n = 5). The concentration used for transfection was defined as 1 × 2.1375 μg mL−1. The proliferation of cells was quantitatively determined with CCK-8 (Dojindo, Kumamoto, Japan) assays, and the results were detected at an optical density of 450 nm with a microplate reader. The optical density (OD) values were recorded 1 day and 3 days after the addition of AuNPs to the culture medium.
Rabbit corneal stromal cells were seeded into 48-well tissue culture plates (BD Biosciences). Cells cultured without AuNPs were used as a control. Cells cultured in medium with 1× AuNPs were used as the experimental group. After the rabbit corneal stromal cells were grown to confluency, a scratch was made through the confluent cells with a 200 μL pipette tip. Micrographs were taken at determined times with an inverted fluorescence microscope (Zeiss Observer A1). Crystal violet staining was used to visualize the cells.
2.4 Cellular uptakes
Free Cy3-labeled miR-133b, miR-NC or its complex with AuNPs were added to cells at a final miR-133b concentration of 50 nM. After incubation for 4 h, cells were extensively washed with cold PBS and fixed with 4% formaldehyde for 15 min. After rinsing twice with PBS, cells were immersed first in 0.1% Triton X-100 for 10 min to increase permeability, then stained with Cell Navigator F-Actin Labeling Kit (AAT Bioquest Inc.) to highlight the cell skeleton (green fluorescence), and finally they were stained with Cell Navigator (10 μg mL−1) to highlight the cell nucleus (blue fluorescence ). Samples were observed using confocal laser scanning microscopy (CLSM, Leica TCS SP8, Germany).
For flow cytometry analysis, cells (2 × 105) were seeded in 6-well plates and pulsed with complexes containing 50 nM miRNA. After 4 h of incubation, cells were rinsed with cold PBS and detached from the wells using trypsin-EDTA. The fluorescence signal within cells indicating miRNA uptake was measured via Guava easyCyte flow cytometry (Merck Millipore).
2.5 Immunofluorescence analysis
Cells were fixed in 4% paraformaldehyde for 15 min. For a-SM and Col-1 indirect immunofluorescence, cells were incubated with mouse monoclonal antibodies (Abcam) overnight after permeabilization with 0.3% Triton X-100. Secondary detection was performed with goat anti-mouse secondary antibodies (Invitrogen). Nuclei were localized by staining with 4,6-diamidino-2-phenylindole (DAPI, 10 μg mL−1). Images of sections were captured on an inverted fluorescence microscope (Zeiss Observer A1).
2.6 RNA isolation and quantitative real-time PCR (qRT-PCR)
We used qRT-PCR to detect myofibroblast transformation marker genes (a-SM and Col-1) in corneal stromal cells. The total RNA was extracted from cell cultures with TRIzol (Invitrogen). The quantity and quality of the isolated RNA were determined with a NanoDrop2000 spectrophotometer (Thermo Scientific). RNA was reverse-transcribed into cDNA with a PrimeScript RT reagent kit with gDNA Eraser (TaKaRa Biotechnology, Japan), according to the manufacturer's protocol. Finally, RT-PCR analysis was performed with a SYBR Green System (GeneCopoeia) on an RT-PCR instrument (QuantStudio 6 Flex, Life Technologies). cDNA samples were amplified at 50 °C for 2 min and 95 °C for 10 min; with 40 cycles at 95 °C for 15 s, 60 °C for 30 s; and 72 °C for 30 s. The relative quantification of target genes was performed through normalization to glyceraldehyde-3-phosphate dehydrogenase (GAPDH), and the 2−ΔCt method was used to calculate the gene expression. The PCR primer sequences were as follows. For GAPDH: sense: 5′-ACTTCGGCATTGTGGAGG-3′; antisense: 5′-GGAGGCAGGGATGATGTTCT-3′; for a-SM: sense: 5′-CCATGCCATCATGCGTCT-3′; antisense: 5′-GCCATCTCGTTTTCAAAGTCC-3′; for Col-1: sense: 5′-GCCTGAGCCAGCAGATTGA-3′; antisense: 5′-AGGTTGCCCCAGTGTCCAT-3′.
2.7 Preparation of collagen-based samples with AuNP/miRNA complexes
Type I collagen was dissolved in 0.1 mol L−1 HCl solution at 4 °C (6.5 mg mL−1). For the preparation of samples with AuNP/miR-133b complexes on the surface (Col-AMS), collagen solution was dispensed into a round mold and air dried. AuNP/miR-133b complexes were added to the surface of the collagen membrane and air dried. For the preparation of samples with AuNP/miR-133b complexes inside the collagen membrane (Col-AMI), AuNP/miR-133b complexes were added into collagen solution. The AuNP dosage in Col-AMS and Col-AMI was 11.34 μg cm−2. The mixed solution was stirred for 4 h at 4 °C to allow for the complete mixing of the solution. Then the mixture was dispensed into a round mold and air dried. Non-modified collagen membrane was used as a control.
2.8 Water content characterization
The equilibrated water content of the hydrogels was measured as previously described.31 The samples were immersed in PBS solution (pH = 7.4) for a specific time period. After being quickly blotted with filter paper to remove superficial water, the weights of the wet samples (Mt) were measured with electronic scales. Then, the samples were vacuum dried to a constant weight (M0). The water content of the samples was calculated according to the following equation: Wt = (Mt − M0)/Mt × 100%. Every reported value was an average of at least five measurements.
2.9 Evaluation of optical properties
Light transmission was measured with a UV3802 ultraviolet-visible spectrophotometer (Shanghai UNICO, Shanghai, China) at 37 °C over a range from 400 to 800 nm. Before testing, samples were immersed in PBS solution (pH = 7.4) for 1 h to absorb water completely, and the samples were then cut into rectangles.
2.10 Evaluation of mechanical properties
The mechanical properties of the samples were measured as previously described.32 The tensile strength, elastic modulus, and elongation at breaking (elasticity) were determined with a uniaxial load testing instrument (Model #5567; Instron Corporation, Issaquah, WA, USA), equipped with a load cell of 10 N capacity at a crosshead speed of 10 mm min−1 and an initial grip separation of 10 mm. The PBS equilibrated samples were cut into dumbbell shaped specimens of identical rectangular gauge areas (width: 5 mm; gauge length: 10 mm; thickness: 0.2 mm) with two 8 mm end tabs. To avoid the breakage and slippage of samples in the jaws, there were 8 mm wide tabs on the end of each dumbbell. Samples were not stress preconditioned before testing to failure. During the tests, the samples were hydrated. Every reported value is an average of at least five measurements.
2.11 LKP model in rabbits
Adult male and female New Zealand white rabbits, 10 weeks of age, and weighing 1.8–2.3 kg, were used as animal transplant models. All animals were treated in accordance with the Association of Vision and Ophthalmology Statement on the Use of Animals in Ophthalmic and Vision Research. Col-AMS samples, 5 mm in diameter, were trephined, then washed in sterile PBS. After the implants were dried, they were sealed with a sealing machine in an aseptic environment. Before surgery, the samples were immersed in sterile water for more than 1 h to absorb water until saturation. Samples were implanted into the right corneas of the rabbits through LKP. Operations were performed on only one eye of each animal. Briefly, in LKP, a 4.5 mm diameter circular incision was made with a trephine in rabbits under general anesthesia. The depth of the lamellar incision was 200–250 mm. A lamellar dissection was then made with a microkeratome along a natural uniform stratum in the corneal stroma to remove the host epithelium and anterior stroma. The sample graft was sutured into the recipient bed with eight interrupted 10–0 nylon sutures. Corneas without repair material after the removal of the anterior stroma were used as a control group. Dexamethasone eye ointment was applied three times daily for 7 days after LKP. Follow-up clinical examinations were performed, including sodium fluorescein staining to assess epithelial integrity, slit lamp microscopy to assess corneal optical clarity, neovascularization determinations, corneal deformation assessments and rejection reaction determinations. The repair statuses of the implants and regenerated neo-corneas were assessed via the examination of changes in thicknesses and shapes. Anterior segment optical coherence tomography (OCT) was used to monitor changes in corneal repair. Hematoxylin–eosin sections were made to examine the recovery of corneal epithelium and stroma. Immunohistochemical analysis was used for the detection of a-SM and Col-1 in the corneal stroma. All animal experiments were performed with permission from the Medicine Ethics Committee at Sun Yat-sen University, China.
2.12 Histology
On the 60th day after transplantation, the rabbits were sacrificed with an overdose intravenous injection of pentobarbital sodium under deep anesthesia. The transplanted and normal corneas were excised from the rabbits and fixed for 24 h in 10% neutral buffered formalin solution at room temperature. They were then cut and stained with hematoxylin–eosin. On the 60th day after transplantation, immunohistochemical staining was performed to observe a-SM and Col-1. Antibodies were purchased from Abcam. The sections were observed with a digital pathology system (Aperio CS2, Leica, Germany).
2.13 Statistical analysis
All data were expressed as means ± standard deviation. Experiments were analyzed using analysis of variance to determine significant differences among groups. Statistical significance was defined as p < 0.05.
3. Results and discussion
3.1 Binding ability of AuNP to miR-133b, and miRNA stability
In this study, we chose AuNPs as a carrier for miRNA delivery. AuNPs delivered with miRNA were able to cross the cell membrane and exert their functions. We analyzed the ability of AuNPs to bind miR-133b by using 1% agarose gel electrophoresis (Fig. 1A). Complete binding of miR-133b was achieved at a volume ratio of 0.2 or higher. This result showed that AuNPs bind miR-133b well at a low dosage. MiR-133b integrity must be preserved during the preparation of materials and before entry into cells. As shown in Fig. 1B, we observed the microstructure of the complex of AuNP/miR-133b via TEM; it was about 15 nm in size. We also compared the integrities of naked miR-133b and miR-133b in AuNP/miR-133b complexes in the presence of RNase at different time points (Fig. 1C). When miR-133b was exposed to RNase, an intact band was observed before 3 hours, but degradation was observed after further incubation, because no band was present in the gel after 4 hours. When miR-133b was combined with AuNP to form complexes, miR-133b integrity persisted until 24 hours after exposure to RNase. These results indicated that the AuNPs protect miR-133b from degradation in the physiological environment before its entry into cells. Next, we tested the integrity of naked miR-133b in collagen solution (Fig. 1S†). MiR-133b integrity in acidic collagen solution persisted after 48 hours at room temperature. Importantly, AuNP/miR-133b complexes remain stable and retain their integrity in collagen solution after several days. These results showed that miR-133b can maintain its integrity during the preparation of collagen-based samples in the form of AuNP/miR-133b complexes.
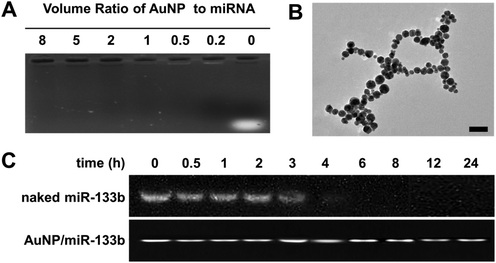 |
| Fig. 1 The characterization of a combination of PEI-capped AuNPs and miR-133b: (A) electrophoretic retardation analysis of miRNA binding; (B) a TEM image for particle size analysis of AuNPs/miR-133b (scale bar = 50 nm); and (C) miRNA stability tests in the presence of RNase. | |
3.2
In vitro biocompatibility and wound healing assays of AuNPs
Before we used AuNP/miR-133b for further study, assessing the cytocompatibility of AuNPs in vitro was the first thing considered. CCK-8 and wound healing assays were performed. The CCK-8 assay results for rabbit corneal stromal cells cultured in media with different AuNP concentrations are shown in Fig. 2A. The AuNPs did not show obvious cytotoxicity at working concentrations; cytotoxicity was apparent only at high concentrations of AuNPs. The wound healing assay results are shown in Fig. 2B. Compared with the control group, the experimental group had a similar healing speed. AuNPs did not have a negative effect on the proliferation and migration of rabbit corneal stromal cells and thus can be used to prepare materials for biomedical applications.
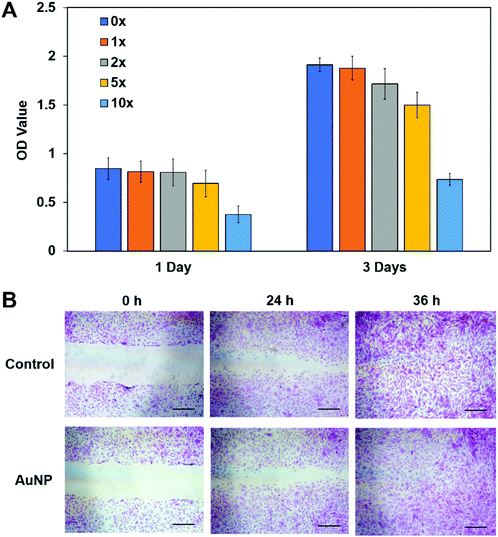 |
| Fig. 2
In vitro biocompatibility of AuNPs. (A) CCK-8 assay of AuNPs cultured with rabbit corneal stromal cells (n = 5) after 1 day and 3 days. (B) Wound healing assays of rabbit corneal stromal cells on tissue culture plates without AuNPs (control) and with 1× AuNPs (scale bar = 200 μm). Photographs were taken 0 h, 24 h and 36 h after the wound was created. | |
3.3 Cellular delivery of the AuNP/miR-133b complex
MiR-133b can work only after it enter into cells. To examine the cellular delivery of miR-133b and AuNP/miR-133b, we chose miR-133b with red fluorescence to experiment with. The final concentration of fluorescently labeled miRNA in complexes with AuNPs was 50 nM. 4 h post transfection, the uptake of AuNP/miR-133b by corneal stromal cells was analyzed using CLSM and fluorescence-activated cell sorting (Fig. 3). Without complexing with AuNPs, Cy3-labeled free miRNA-133b could not enter into cells and showed no detectable fluorescence. Dispersed red fluorescence signals could be observed clearly in cells treated with Cy3-labeled miRNA-133b complexed with AuNPs. The red fluorescence signals were primarily located in the cytoplasm close to the nucleus in these cells. These results indicate that AuNP/miR-133b can enter into the cytoplasm of corneal stromal cells with relatively high efficiency.
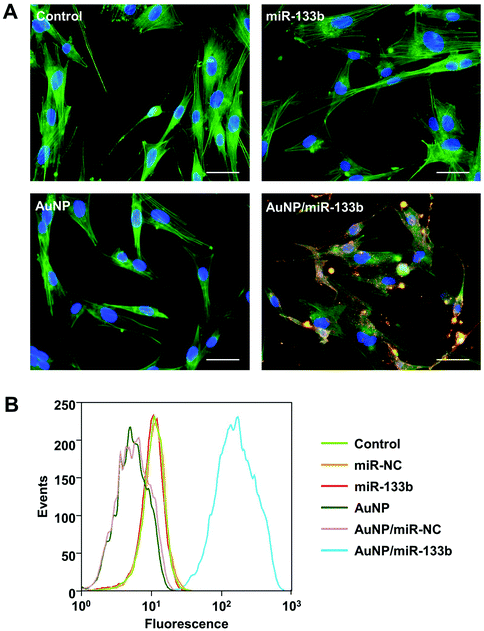 |
| Fig. 3 The cellular uptake ability of AuNPs/miR-133b complexes in corneal stromal cells: (A) confocal microscopy images of corneal stromal cells after treatment with 50 nM Cy3-miR-133b and AuNPs/Cy3-miR-133b (scale bar = 20 μm); and (B) flow cytometry histograms of corneal stromal cells. | |
After confirming the cellular delivery of the AuNP/miR-133b complex, we further tested the cellular delivery of the complex after Col-AMS and Col-AMI preparation. As shown in Fig. 2S,† after incubation with Col-AMS and Col-AMI for 8 h, red fluorescence signals could be observed clearly in cells treated with Col-AMS and Col-AMI. These results indicated that AuNP/miR-133b can be released from the samples and enter into cells with relatively high efficiency. AuNP/miR-133b could maintain its ability to enter into cells after the material preparation.
3.4 Expression of proteins and genes related to myofibroblast transformation
According to previous reports, TGF-β1 secreted from epithelium cells can stimulate corneal stroma cells to transform into myofibroblasts.33,34 In addition, it promotes excessive extracellular matrix deposition in the wound bed and leads to scar formation during the wound healing process.35–37 TGF-β1 can pass through damaged Bowman's membranes and stimulate corneal stroma cells to transform into myofibroblasts.38,39 Myofibroblasts secrete collagen with no orderly pattern, forming scars.13,40 This irregular secretion is the main reason for corneal opacification after trauma. A-SM and Col-1 are marker genes for myofibroblasts, which corneal stroma cells hardly express.41 The overexpression of a-SM and Col-1 could be a signal of scar formation.
We then evaluated the ability of miRNA-133b to inhibit the myofibroblast transformation of corneal stroma in the presence of TGF-β1, which simulated the corneal wound healing process. Two related proteins, a-SM and Col-1, were examined through immunofluorescence analysis after 2 days (Fig. 4). There was little staining from a-SM and Col-1 when corneal stromal cells were cultured without TGF-β1; in contrast, obvious staining was observed in the presence of TGF-β1. However, a-SM and Col-1 expression was lower when AuNP/miR-133b complexes were added into the medium. In contrast, AuNPs and AuNP/miR-NC complexes did not have the same effect. To further quantify the inhibition ability, a-SM and Col-1, two key markers for myofibroblast transformation, were also measured with qRT-PCR (Fig. 5). The mRNA levels of a-SM and Col-1 2 days after cultivation were clearly enhanced in the presence of TGF-β1. Together with the immunofluorescence analysis, these results indicated that TGF-β1 effectively stimulates myofibroblast transformation. The levels of a-SM and Col-1 were lower when corneal stromal cells were cultured with AuNP/miR-133b complexes. Moreover, this expression was comparable to the control group with TGF-β1 after culturing with only AuNPs and AuNP/miR-NC complexes. These findings were consistent with those from the immunofluorescence analysis. Our results thus indicate that miR-133b inhibits the myofibroblast transformation of corneal stromal cells.
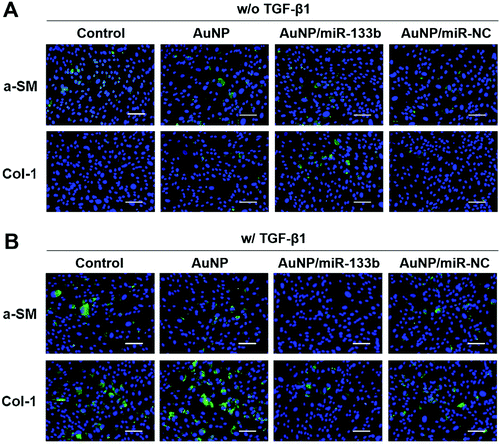 |
| Fig. 4 Micrographs showing immunofluorescence staining of a-SM and Col-1 (green) in corneal stromal cells under different conditions, (A) without and (B) with TGF-β1. Nuclei were visualized by staining with DAPI (blue). Scale bar = 100 μm. | |
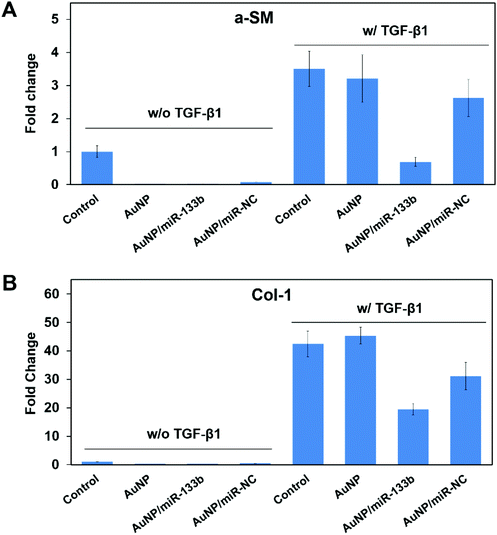 |
| Fig. 5 qRT-PCR analysis of mRNA levels of myofibroblast transformation genes after culturing for 2 days, with or without TGF-β1 (2.5 ng mL−1). Gene expression of a-SM (A) and Col-1 (B) (133b = miRNA-133b; NC = miRNA negative control). | |
3.5 Characterization of collagen-based samples with AuNP/miRNA complexes
Our previous study has shown that collagen-based membranes have appropriate water content, excellent optical transparency and sufficient mechanical strength for biological applications.42 These properties are important to ensure that cornea repair materials are non-stimulating, transparent and intact after operations and postoperative care. Whether the addition of AuNPs into collagen might significantly affect the properties of collagen requires further study. We loaded AuNP/miR-133b complexes through two methods: loading on the surface of (Col-AMS) and inside (Col-AMI) the collagen membrane. The physical and chemical properties of the membranes are shown in Fig. 6. The water content values of both Col-AMS (84.1 ± 0.8%) and Col-AMI (87.872 ± 2.156%) were similar to that of Col (83.4 ± 1.7%). The transmittances of Col-AMS, Col-AMI and Col showed no significant difference under visible light. The tensile strength, elongation at break and Young's modulus values of Col, Col-AMS and Col-AMI also showed no significant differences. From these results, we conclude that the addition of AuNPs did not substantially affect the properties of collagen samples.
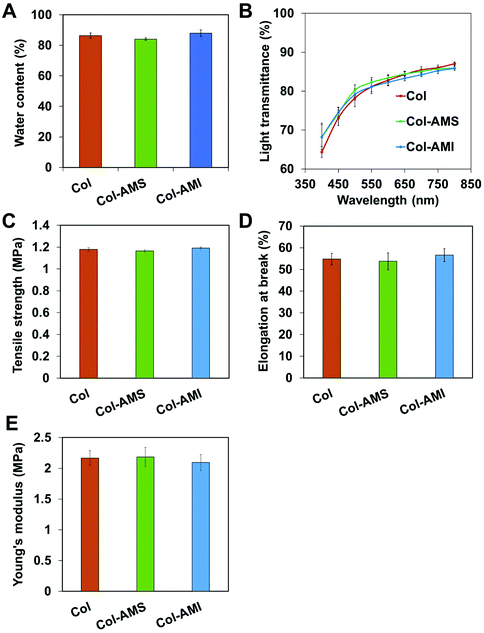 |
| Fig. 6 The characterization of the physical and chemical properties of Col, Col-AMS and Col-AMI membranes: (A) water content; (B) optical properties over the range from near ultraviolet to visible light; and mechanical properties, including (C) tensile strength, (D) elongation at break and (E) modulus. | |
3.6 Evaluation of collagen-based membranes in an LKP model
AuNP/miR-133b complexes were found to be effective in the inhibition of myofibroblast transformation and were able to be combined with collagen to form membranes suitable for cornea repair. AuNP/miR-133b has been proven to show excellent cellular delivery in vitro. We further confirmed the release of AuNP/miR-133b by the samples in vivo, and the results are shown in Fig. 3S.† Green fluorescence signals could be observed clearly in the rabbit corneal stroma layer, which was close to the field of operation. AuNP/miR-133b could be released from Col-AMS and Col-AMI and not only enter into cells in vitro but also enter into corneal stromal tissue in vivo. This can make sure that AuNP/miR-133b can be released from Col-AMS and Col-AMI and enter into cells to work.
Col-AMS, Col-AMI and Col were transplanted into rabbit corneas to characterize the repair effects and scar inhibition. The surgical sutures were removed after 1 week. The healing progress is shown in Fig. 7. Epithelization was completed in approximately 7 days in all groups. No keratoconus was observed within 8 weeks. Obvious leukoma was observed in the control group after 14 days. This result indicated that the cornea exhibited cicatricial repair after trauma without treatment. The leukoma persisted until 56 days after operation. After a scar is formed, the cornea cannot restore transparency through self-repair. However, haze was scarcely observed in the Col-AMS and Col-AMS groups. Slight leukoma was seen in the Col group after 21 days, but this was not visible to the naked eye at other time points.
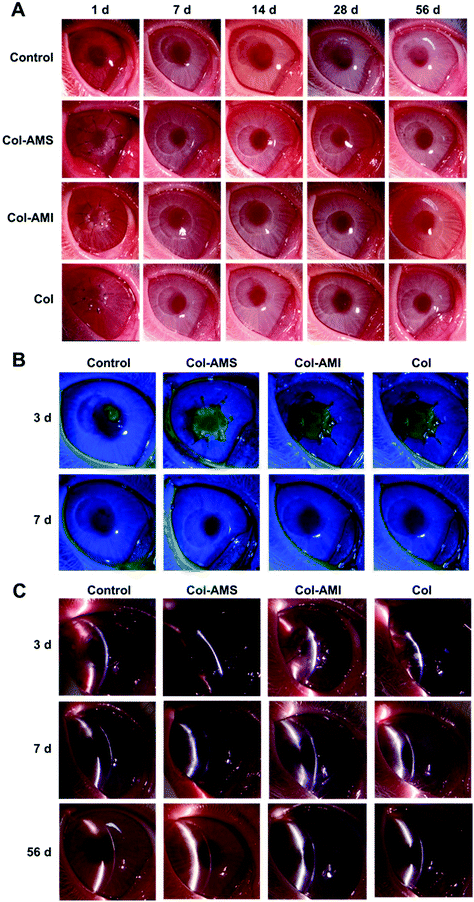 |
| Fig. 7 (A) Post-operative observation of LKP, using Col-AMS, Col-AMI and Col. Corneas without repair material after the removal of the anterior stroma were used as a control group. The depth of the lamellar incision was approximately 200 μm. Photographs were taken 1 d, 7 d, 14 d, 28 d and 56 d after operation. (B) The process of re-epithelialization. (C) Slit lamp biomicroscopy photographs. | |
To further examine the scar tissue, we used anterior segment OCT to examine the cornea after operation. Although granulation tissue, corneal edema and scar tissue have similar images in OCT, we could confirm permanent scars after 1 month using OCT, on account of corneal edema and granulation tissue, which was able to regress after 2–3 weeks, and scar tissue, which could not be repaired once formed.42,43 The OCT photographs are shown in Fig. 8A and OCT imaging of a healthy cornea is shown in Fig. 4S.† Wide white stripes were seen after 14 days in the control group, thus indicating that disordered collagen had formed in the neo-stroma during the repair process. This led to leukoma and was consistent with the results in Fig. 7. The white stripes were less pronounced in the Col group than in the control group, but were nonetheless observed at 14, 21, 28, 42, and 56 days. This result may have occurred because the collagen membrane can partly obstruct contact between epithelial cells and stromal cells. Because TGF-β1 cannot enter into the stroma directly, it was difficult to observe haze with the naked eye. These results were consistent with findings from previous research.44 Unlike the control and Col groups, the Col-AMS and Col-AMI groups had much less scar tissue, and the corneal stroma was more homogeneous in OCT images. Histological sections through the operative region of each group (Fig. 8B) indicated that corneas transplanted with samples exhibited normal and healthy corneal architecture. Epithelial proliferation was observed in the control and collagen groups, possibly because the stroma had not regenerated well. To make the cornea surface smooth, the epithelial cells may have proliferated and formed a thicker epithelial layer than in a healthy cornea. A large number of myofibroblasts can be observed in the stroma of the control group, indicating that cornea damage without treatment could lead to fibrotic healing. These cells are non-transparent and secrete collagen with no orderly pattern, finally leading to scar formation.39,45 This result is consistent with the results in Fig. 7 and 8A. To observe a-SM and Col-1 expression in vivo after the operation, immunohistochemical staining was performed (Fig. 8C). The expression of these markers was higher in the control group than the collagen group and the experimental group treated with AuNP/miR-133b complexes. There was no obvious difference between the collagen group and experimental group. These results were explained because the materials with AuNP/miR-133b complexes probably inhibit the protein expression of the myofibroblast transformation markers (a-SM and Col-1) only in the early postoperative stage.
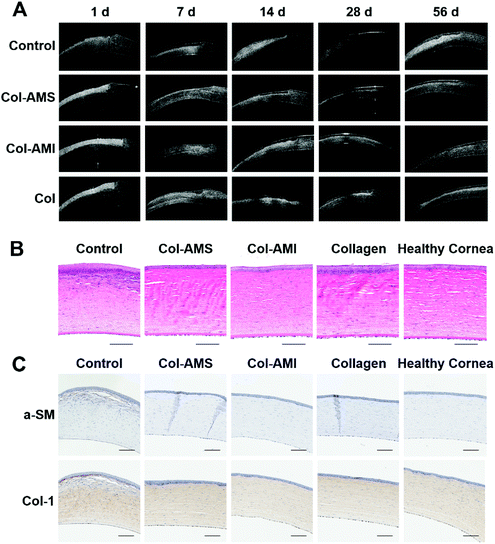 |
| Fig. 8 (A) Anterior segment optical coherence tomography images of corneas repaired with different membranes, over the course of 2 months. Corneas without repair material after the removal of the anterior stroma were used as a control group. (B) Hematoxylin–eosin-stained sections of different membranes transplanted into the ocular surface in rabbits, and a healthy cornea, over the course of 2 months. (C) Immunohistochemical staining section of a-SM and Col-1 in different membranes transplanted into the ocular surface in rabbits, and a healthy cornea, over the course of 2 months. Scale bar = 100 μm. | |
According to other research, nanoparticles with larger diameters accumulate in the spleen and liver in vivo, due to a binding or filtration mechanism in biological systems,46 and small sized nanoparticles accumulate a lot in the kidneys.47,48 The nanoparticles used in the cornea were first introduced into the cornea stroma to work. Some of them may pass through the anterior chamber and get into circulation, and some of them may be excreted out of the body in tears over time.49,50 Moderate AuNP concentrations will not induce toxicity. In further material design, Col-AMS may be adapted to the case of only affecting one side of samples or being quick-release, while Col-AMI may be adapted to the case of acting on both sides and having slow release.
4. Conclusions
We developed collagen-based materials containing AuNP/miR-133b complexes as cornea repair materials with the ability to provide scarless healing. The addition of AuNPs did not affect the collagen properties related to cornea regeneration. Moreover, the microRNA retained its function in collagen-based materials. We demonstrated the applicability of these materials in vitro/in vivo. After transplantation, AuNP/miR-133b was released from Col-AMS and Col-AMI and entered into stromal cells. AuNP/miR-133b can down-regulate the expression of a-SM and Col-1 to inhibit scar formation. Col-AMS and Col-AMI have excellent biocompatibility and the ability to inhibit scar formation in corneal stroma.
Conflicts of interest
There are no conflicts to declare.
Acknowledgements
This work was financially supported by the National Key R&D Program of China (2017YFC1105000), the National Natural Science Foundation of China (51232002, 51603073), the Guangdong Scientific and Technological Project (2014B090907004, 2017A030313294), the Pearl River S&T Nova Program of Guangzhou (201710010195), and the Fundamental Research Funds for the Central Universities.
References
- B. Rra, S. R. Flaxman, T. Braithwaite, M. V. Cicinelli, A. Das, J. B. Jonas, J. Keeffe, J. H. Kempen, J. Leasher and H. Limburg, Lancet Global Health, 2017, 5, 1–10 Search PubMed.
- D. Bremond-Gignac, H. Copin and M. Benkhalifa, Expert. Opin. Biol. Ther., 2018, 1–7, DOI:10.1080/14712598.2018.1508443.
- J. C. Dayoub, F. Cortese, A. Anzic, T. Grum and J. P. de Magalhaes, Exp. Gerontol., 2018, 110, 230–240 CrossRef PubMed.
- A. Tidu, D. Ghoubay-Benallaoua, C. Teulon, S. Asnacios, K. Grieve, F. Portier, M.-C. Schanne-Klein, V. Borderie and G. Mosser, Biomater. Sci., 2018, 6, 1492–1502 RSC.
- C. Kilic, A. Girotti, C. Rodriguez-Cabello and V. Hasirci, Biomater. Sci., 2014, 2, 318–329 RSC.
- F. E. Kruse, K. Rohrschneider and H. E. Völcker, Ophthalmology, 1999, 106, 1504–1511 CrossRef CAS PubMed.
- B. Seitz, Ophthalmologe, 2007, 104, 1075–1079 CrossRef CAS PubMed.
- B. Seitz, M. D. Resch, U. Schlötzer-Schrehardt, C. Hofmann-Rummelt, R. Sauer and F. E. Kruse, Arch. Ophthalmol., 2006, 124, 1487–1490 CrossRef PubMed.
- H. Y. Wang, R. H. Wei and S. Z. Zhao, Int. J. Ophthalmol., 2013, 6, 873–878 CAS.
- X. Zhao, W. J. Song, S. Liu and L. Ren, Sci. China: Chem., 2016, 59, 1548–1553 CrossRef CAS.
- P. Fagerholm, N. S. Lagali, K. Merrett, W. B. Jackson, R. Munger, Y. W. Liu, J. W. Polarek, M. Soderqvist and M. Griffith, Sci. Transl. Med., 2010, 2, 46–61 Search PubMed.
- P. Fagerholm, N. S. Lagali, J. A. Ong, K. Merrett, W. B. Jackson, J. W. Polarek, E. J. Suuronen, Y. Liu, I. Brunette and M. Griffith, Biomaterials, 2014, 35, 2420–2427 CrossRef CAS PubMed.
- K. E. Myrna, S. A. Pot and C. J. Murphy, Vet. Ophthalmol., 2009, 12(Suppl 1), 25–27 CrossRef PubMed.
- A. Stejskalová and B. D. Almquist, Biomater. Sci., 2017, 5, 1421–1434 RSC.
- J. V. Jester, A. S. Budge and J. Huang, Invest. Ophth. Vis. Sci., 2005, 46, 2369–2378 CrossRef PubMed.
- X. Y. Ma, Y. Zhang, D. Zhu, Y. Lu, G. D. Zhou, W. Liu, Y. L. Cao and W. J. Zhang, PLoS One, 2015, 10, 1–13 CAS.
- A. V. Ljubimov and M. Saghizadeh, Prog. Retinal Eye Res., 2015, 49, 17–45 CrossRef CAS PubMed.
- K. R. Beavers, C. E. Nelson and C. L. Duvall, Adv. Drug Delivery Rev., 2015, 88, 123–137 CrossRef CAS PubMed.
- W. P. Kloosterman and R. H. Plasterk, Dev. Cell, 2006, 11, 441–450 CrossRef CAS PubMed.
- D. P. Bartel, Cell, 2009, 136, 215–233 CrossRef CAS PubMed.
- W. Filipowicz, S. N. Bhattacharyya and N. Sonenberg, Nat. Rev. Genet., 2008, 9, 102–114 CrossRef CAS PubMed.
- X. Liu, L. Ma and C. Y. Gao, Organogenesis, 2013, 9, 76–78 CrossRef PubMed.
- X. Liu, L. Ma, J. Liang, B. Zhang, J. Y. Teng and C. Y. Gao, Biomaterials, 2013, 34, 2038–2048 CrossRef CAS PubMed.
- K. M. Blum, T. Novak, L. Watkins, C. P. Neu, J. M. Wallace, Z. R. Bart and S. L. Voytik-Harbin, Biomater. Sci., 2016, 4, 711–723 RSC.
- P. M. Robinson, T. D. Chuang, S. Sriram, L. Pi, X. P. Luo, B. E. Petersen and G. S. Schultz, Invest. Ophthalmol. Visual Sci., 2013, 54, 6944–6951 CrossRef CAS PubMed.
- C. V. Pecot, G. A. Calin, R. L. Coleman, G. Lopez-Berestein and A. K. Sood, Nat. Rev. Cancer, 2011, 11, 59–67 CrossRef CAS PubMed.
- W. Liao, W. Li, T. Zhang, M. Kirberger, J. Liu, P. Wang, W. Chen and Y. Wang, Biomater. Sci., 2016, 4, 1051–1061 RSC.
- C. Zhang, X. Li, C. Tian, G. Yu, Y. Li, W. Jiang and C. Mao, ACS Nano, 2014, 8, 1130–1135 CrossRef CAS PubMed.
- J. Elbaz, A. Cecconello, Z. Fan, A. O. Govorov and I. Willner, Nat. Commun., 2013, 4, 1–7 Search PubMed.
- W. J. Song, J. Z. Du, T. M. Sun, P. Z. Zhang and J. Wang, Small, 2010, 6, 239–246 CrossRef CAS PubMed.
- Y. Liu, L. Ren, K. Long, L. Wang and Y. Wang, Acta Biomater., 2014, 10, 289–299 CrossRef CAS PubMed.
- Y. Liu, L. Ren and Y. J. Wang, Mat. Sci. Eng., C, 2013, 33, 196–201 CrossRef CAS PubMed.
- H. C. Baldwin and J. Marshall, Acta Ophthalmol. Scand., 2002, 80, 238–247 CrossRef CAS PubMed.
- F. Malecaze, D. Massoudi, P. Fournie, C. Tricoire, M. Cassagne, M. Malbouyres, D. J. S. Hulmes, C. Moali and S. D. Galiacy, Invest. Ophthalmol. Visual Sci., 2014, 55, 6712–6721 CrossRef CAS PubMed.
- L. Shi, Y. Chang, Y. M. Yang, Y. Zhang, F. S. X. Yu and X. Y. Wu, PLoS One, 2012, 7, 1–9 Search PubMed.
- D. Karamichos, A. E. K. Hutcheon and J. D. Zieske, Exp. Eye Res., 2014, 124, 31–36 CrossRef CAS PubMed.
- A. A. M. Torricelli and S. E. Wilson, Exp. Eye Res., 2014, 129, 151–160 CrossRef CAS PubMed.
- B. M. Stramer, J. D. Zieske, J. C. Jung, J. S. Austin and M. E. Fini, Invest. Ophthalmol. Visual Sci., 2003, 44, 4237–4246 CrossRef.
- T. Kawakita, E. M. Espana, H. He, R. Smiddy, J. M. Parel, C. Y. Liu and S. C. G. Tseng, Invest. Ophthalmol. Visual Sci., 2006, 47, 1918–1927 CrossRef PubMed.
- M. E. Fini and B. M. Stramer, Cornea, 2005, 24, S2–S11 CrossRef PubMed.
- A. Bukowiecki, D. Hos, C. Cursiefen and S. A. Eming, Int. J. Mol. Sci., 2017, 18, 1–24 Search PubMed.
- T. Sumioka, A. Kitano, K. C. Flanders, Y. Okada, O. Yamanaka, N. Fujita, H. Iwanishi, W. W. Y. Kao and S. Saika, Lab. Invest., 2013, 93, 207–217 CrossRef CAS PubMed.
- I. S. Zagon, M. S. Klocek, J. W. Griffith, J. W. Sassani, A. M. Komaromy and P. McLaughlin, Arch. Ophthalmol., 2008, 126, 501–506 CrossRef PubMed.
- X. Zhao, W. Song, W. Li, S. Liu, L. Wang and L. Ren, RSC Adv., 2017, 7, 28865–28875 RSC.
- T. Møllerpedersen, Exp. Eye Res., 2004, 78, 553–560 CrossRef.
- S. Ravindran, J. K. Suthar, R. Rokade, P. Deshpande, P. Singh, A. Pratinidhi, R. Khambadkhar and S. Utekar, Curr. Drug Metab., 2018, 19, 327–334 CrossRef CAS PubMed.
- C. Perez-Campana, V. Gomez-Vallejo, M. Puigivila, A. Martin, T. Calvo-Fernandez, S. E. Moya, R. F. Ziolo, T. Reese and J. Llop, ACS Nano, 2013, 7, 3498–3505 CrossRef CAS PubMed.
- G. Sonavane, K. Tomoda and K. Makino, Colloids Surf., B, 2008, 66, 274–280 CrossRef CAS PubMed.
- A. Sharma, A. Tandon, J. C. K. Tovey, R. Gupta, J. D. Robertson, J. A. Fortune, A. M. Klibanov, J. W. Cowden, F. G. Rieger and R. R. Mohan, Nanomed. Nanotechnol., 2011, 7, 505–513 CrossRef CAS PubMed.
- R. R. Mohan, S. Sinha, K. V. Katti, R. Kannan, W. M. Stapleton and G. S. Schultz, J. Neurosurg., 2007, 76, 1029–1031 Search PubMed.
Footnote |
† Electronic supplementary information (ESI) available. See DOI: 10.1039/c8bm01054d |
|
This journal is © The Royal Society of Chemistry 2019 |