DOI:
10.1039/C8BM01042K
(Paper)
Biomater. Sci., 2019,
7, 429-441
Hypoxia- and singlet oxygen-responsive chemo-photodynamic Micelles featured with glutathione depletion and aldehyde production†
Received
28th August 2018
, Accepted 15th November 2018
First published on 16th November 2018
Abstract
Triggered drug release from anti-tumor nanomedicine is an efficient approach to address the dilemma of systemic nanocarrier stability and on-demand drug liberation in tumor sites. Combinational drug delivery has been a useful means to enhance antitumor efficacy and reduce adverse effects. We report a multifunctional micelle for dually hypoxia- and singlet oxygen-responsive integration of chemotherapy and photodynamic therapy. The micelles were made of a nitroimidazole (NI)-bearing polymer; doxorubicin (Dox) and chlorin e6 (Ce6) were selected as the model chemodrug and photosensitizer, respectively. The co-delivery micelles displayed a hydrodynamic size of 138.5 ± 3.6 nm with the cargo loading at 2.5 ± 0.2% w/w (Dox) and 1.8 ± 0.3% w/w (Ce6), respectively. Under hypoxia (e.g. tumor microenvironment), the NI moiety was bio-reduced to aminoimidazole, resulting in micelle disassembly, rapid cargo release, and glutathione (GSH) depletion. Upon laser irradiation, the singlet oxygen produced by Ce6 caused the oxidation of NI, leading to micelle collapse, facilitated payload release, and the production of aldehyde end-products. Rapid drug release enabled the fast onset of the therapeutic action. GSH depletion and aldehyde production would provide a supplementary effect to enhance the anti-tumor efficacy of co-delivery micelles. This proof-of-concept was demonstrated in a murine mammary carcinoma cell line (4T1) in vitro as well as in a 4T1 tumor-bearing mouse model in vivo. This study expanded the function of traditional stimuli-responsive nanomedicines by utilizing the multifunctional NI moiety that could realize both triggered release and the amplified anti-tumor effect via the auxiliary action of GSH depletion and aldehyde production.
1. Introduction
Combinational drug delivery has been an efficient approach for tumor management.1–3 The benefits of co-delivery of different active pharmaceutical ingredients (APIs) include simultaneous targeting of multiple targets for enhanced anti-tumor efficacy, reduced adverse effects via lowering the drug dose, and reversal of multidrug resistance.4–6 The combinational delivery has not been limited to chemodrugs; it also involves the synergism between chemotherapy and other treatment modalities, among which the integration between chemotherapy and photodynamic therapy (PDT) has been prevalent.7–10 PDT is a unique anti-tumor technique, for which a photosensitizer is activated upon light irradiation to produce highly active singlet oxygen (1O2) that acts as the active species to induce cytotoxicity.11 However, the simple cocktail formulation is not ideal for the co-delivery of multiple APIs that usually display different physiochemical properties, resulting in un-identical pharmacokinetic and biodistribution behavior, and hence compromised in vivo anti-tumor efficacy.12 The employment of nanocarriers as the co-delivery vehicle for combinational anti-tumor therapy can address the aforementioned issues.13–15
Polymeric micelles are robust pharmaceutical vehicles that can be engineered with good stability and passive and active targeting ability to aid combinational drug delivery.16–18 Moreover, the micelles can be tailored to be stimuli-responsive for preventing premature drug release during systemic circulation, and enabling triggered drug liberation in tumor sites.19–21 Various internal and external triggers are available for precise engineering micelle performance; among these, hypoxia is a distinct one as the solid tumor is characterized by a very low oxygen content.22–24 Azobenzene and nitroimidazole (NI) are two regular functional moieties to build hypoxia-responsive micelles.25–27 Regarding the NI moiety, it is often attached to the hydrophobic segment of the amphiphilic copolymer and is converted to hydrophilic aminoimidazole under hypoxia, leading to micelle disassembly and cargo release. Our recent work further demonstrated that NI-bearing micelles were also sensitive to singlet oxygen with the hydrophilic oxamic aldehyde as the end-product.28 Such discovery rendered the NI-bearing micelles dually responsive to both hypoxia and singlet oxygen, which would be valuable in PDT-based anti-tumor therapy.
Because NI-bearing micelles could produce aldehyde that was cytotoxic, it was hypothesized that such micelles would have an amplified anti-tumor effect in delivering photosensitizers. In addition, the conversion of NI to aminoimidazole consumes nicotinamide adenine dinucleotide phosphate (NADPH) that is a key component to maintain the redox cycle between glutathione (GSH) and glutathione disulfide (GSSG).29 It was further postulated that the hypoxia-induced NI-aminoimidazole conversion would reduce intracellular NADPH concentration, which could in turn kinetically decrease the GSH content in the cytosol. As the high GSH level is one major defense mechanism against the oxidative damage of singlet oxygen in PDT, the NI-bearing micelles would offer the additional efficacy amplification effect for anti-tumor PDT. Moreover, the GSH is reported able to confer resistance to a number of chemotherapeutic drugs and detoxify xenobiotics.30,31 Hence, GSH depletion holds promise for enhancing the potency of these agents.
Therefore, the aim of this study was to employ multifunctional NI-bearing polymeric micelles as the nanoscale platform to co-deliver a cytotoxic agent and a photosensitizer for enhanced anti-tumor performance in vivo. We believe that such a system could display a “four birds with one stone” effect to boost the therapeutic outcome, i.e. hypoxia-responsiveness, singlet oxygen-sensitivity, aldehyde production, and GSH diminishment (Scheme 1). NI was covalently linked to the side chains of methoxy poly(ethylene glycol)-co-poly(aspartic acid) (i.e. mPEG-P(Asp-NI)) (Fig. S1, ESI†), producing self-assembling polymer conjugate micelles. Doxorubicin (DOX) and chlorin e6 (Ce6) were selected as the model cytotoxic drug and photosensitizer, respectively, and both agents were co-encapsulated in the hydrophobic micellar core.
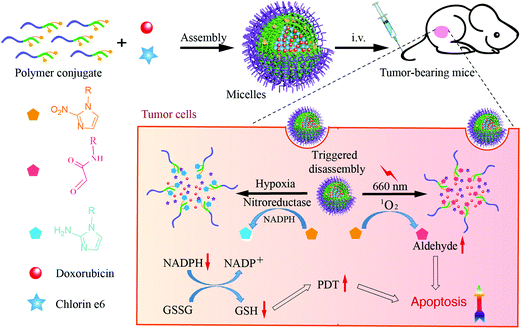 |
| Scheme 1 Dually responsive polymer conjugate micelles for enhanced combinational delivery of doxorubicin and chlorin e6 (Ce6) in a tumor-bearing mice model. Both drugs were co-loaded in the mPEG-PAsp-NI conjugate micelles. The NI moiety was converted to hydrophilic aminoimidazole under hypoxia, accompanied by the reduction of glutathione (GSH). Upon laser irradiation, NI could be oxidized to aldehyde, resulting in micelle disassembly and cargo liberation. | |
2. Results and discussion
2.1 Singlet oxygen responsiveness and aldehyde production
The synthesis of mPEG-PAsp-NI conjugate employed our recently published method; the obtained conjugate displayed a molecular weight of 7800 Da and the degree of aspartic acid polymerization and NI conjugation both were ca. 10, i.e. all the side chains of the polypeptide were occupied by the NI moiety.28 The NI-bearing amphiphilic polymer conjugate could self-assemble into micelles and the drug loading was determined at 2.5 ± 0.2% w/w (Dox) and 1.8 ± 0.3% w/w (Ce6), respectively. The absorption and emission spectra proved the successful cargo loading and hypoxia-triggered drug release (Fig. S2–S5†). The hydrodynamic diameter of placebo and co-loaded micellar nanocarriers (NCs/Dox + Ce6) was 109.7 ± 3.7 nm and 138.5 ± 3.6 nm, respectively. The cargo encapsulation-induced micelle size increase was a typical phenomenon because the payload occupies some space in the micelle core and induces core expansion.16,32 Under the same laser irradiation conditions (660 nm), the hydrodynamic size of placebo NCs remained unchanged (Fig. 1A), whereas the drug-loaded NCs/Dox + Ce6 experienced a dramatic size expansion (Fig. 1B). This was demonstrated due to that the singlet oxygen produced by Ce6 under laser treatment oxidized the NI moiety to aldehyde, resulting in the change of conjugate amphiphilicity and micelle disassembly (Fig. 1C and Fig. S6†).28 The formation of the oxamic aldehyde end-product was detected by a fluorescence-based method (Fig. S7†). The singlet oxygen-induced micelle disassembly significantly speeded up the release of both Dox and Ce6, which was proven by the distinctive release curve in the presence and absence of laser irradiation (Fig. 1D and E). A similar phenomenon was also observed previously in imidazole-bearing micelles.21
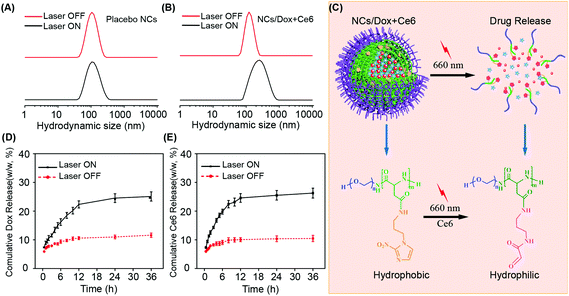 |
| Fig. 1 Hydrodynamic size of placebo micellar nanocarriers (NCs) (A) and drug-loaded micelles (NCs/Dox + Ce6) (B) with or without laser treatment (660 nm, 30 min, 100 mW cm−2). (C) Illustration of micelle disassembly induced by nitroimidazole oxidation upon the production of singlet oxygen. Cumulative Dox (D) and Ce6 (E) release in vitro in the presence or absence of laser irradiation (660 nm, 10 min, 100 mW cm−2) (n = 3). | |
2.2 Hypoxia responsiveness and GSH depletion
The current work employed a murine mammary carcinoma cell line (4T1) to determine the ability of NI micelles to deplete intracellular GSH. The obtained results agreed well with our expectation; both the small molecule (NI) and the NI-bearing conjugate micelles successfully induced GSH depletion in a kinetic manner (Fig. 2A and B). The conversion of hydrophobic NI to hydrophilic aminoimidazole under hypoxia has been a well-known phenomenon; the process is catalyzed by various nitroreductases with the aid of NADPH.33 NADPH involves many chemical systems to reduce the activity of intracellular reactive oxygen species (ROS) and hence control the redox potential with GSH as the major player.29 NADPH works together with glutathione reductase (GR) to reduce GSSG to GSH and maintain an appropriate redox potential. However, when sufficient amounts of intracellular NADPH were consumed by the nitroreductases for NI bioreduction under a hypoxic tumor microenvironment, the redox cycle between GSH and GSSG could be kinetically affected, leading to GSH depletion (Fig. 2C). The reduction of the intracellular GSH level could compromise the ability of cells to fight against ROS, which would be beneficial for PDT and related combination therapy. The hypoxia-facilitated payload release in vitro was not performed due to the difficulty in constant sampling under hypoxic conditions. Nevertheless, this is an eminent phenomenon and a plenty of previous studies have utilized such a mechanism to enhance drug release.27,34,35
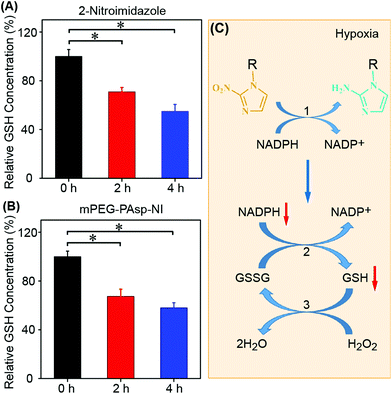 |
| Fig. 2 The relative GSH concentration in the 4T1 cells upon incubation with 2-nitroimidazole (A) or mPEG-PAsp-NI (B) under hypoxia. (C) The proposed mechanism of NI-induced GSH depletion (1: nitroreductase; 2: glutathione reductase; 3: glutathione peroxidase). *p < 0.05. | |
2.3 Cell viability
In the absence of laser irradiation, the free drug combination (Dox + Ce6) displayed similar cytotoxicity between hypoxic and normoxic conditions (Fig. 3A). As no laser was applied, no singlet oxygen should be produced and the cytotoxicity was presumed primarily due to the contribution of Dox. For the same formulation (Dox + Ce6), the presence of laser treatment produced a significant difference between the two conditions (hypoxia versus normoxia) (Fig. 3B, Table S1†) (p < 0.05). This was thought as a consequence of more singlet oxygen production under normoxia. Regarding the micellar formulation (NCs/Dox + Ce6), the cells maintained good viability under the laser-free conditions (Fig. 3C). Two reasons contributed to this result: (1) no singlet oxygen was generated without irradiation and (2) Dox release from micelles was very slow. Interestingly, when switching to the hypoxic environment, the micelles induced significant cytotoxicity (IC50/Dox: 7.3 ± 0.9 μM) because of hypoxia-responsive payload release and the released free Dox could exert the toxic action. Irrespective of the oxygen level, the co-loaded micelles displayed enhanced cytotoxicity in the presence of irradiation compared to that in the laser OFF situation (p < 0.05), which was because of the action of singlet oxygen generated by Ce6 (Fig. 3D and Table S1†). Despite the low oxygen-induced discount of PDT efficacy under hypoxia, the NI-aminoimidazole conversion could induce micelle disassembly and aid cargo release, which was accompanied by GSH depletion that could amplify the PDT effect. Under normoxia, the NI oxidation-induced micelle collapse and payload release plus the production of toxic aldehyde contributed to the efficacy enhancement of micellar formulations. Considering all these factors together, the co-delivery micelles showed slightly higher cytotoxicity under normoxia. The drug-free micelles displayed no cytotoxicity regardless of the irradiation and oxygen level (Fig. S8 and S9†).
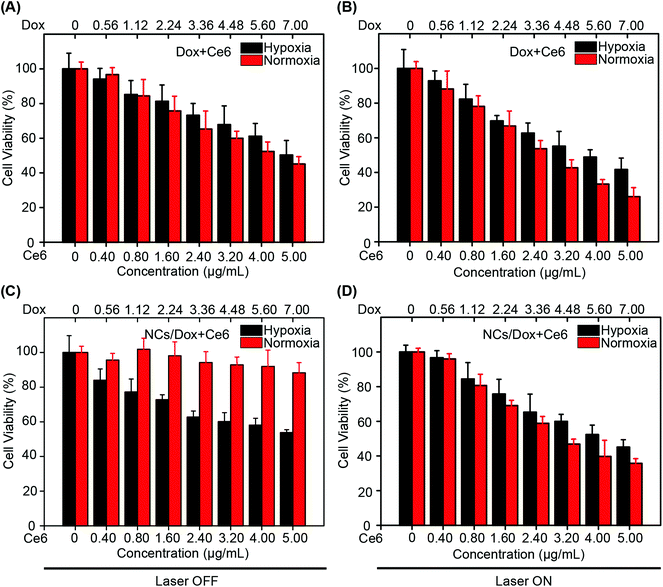 |
| Fig. 3 Viability of 4T1 cells in response to the free drug and micellar formulations under hypoxia or normoxia (n = 4). The cells were treated with free Dox + Ce6 without (A) or with (B) laser treatment; the cells were treated with drug-loaded micelles (NCs/Dox + Ce6) without (C) or with (D) laser irradiation. The irradiation conditions were fixed at 660 nm, 100 mW cm−2, 10 min. | |
2.4 Intracellular triggered drug release
Because both Dox and Ce6 show inherent fluorescence, it is convenient to examine drug release in situ using a confocal laser scanning microscope (CLSM) (Fig. 4A). The drug release mechanisms in response to irradiation and hypoxia trigger were also shown (Fig. 4B). We employed the Pearson's correlation coefficient (PCC) between Dox and DAPI to quantitatively assess the drug release.36,37 DAPI was used to indicate the location of nuclei. The reason to select the Dox/DAPI pair was because the nucleus is the site of action of Dox. PCC indicates the linear correlation between two variables and has a value ranging from −1 to +1, where −1 is total negative linear correlation, +1 is the total positive linear correlation, and 0 means no linear correlation.37 Under hypoxia, it was clear that Dox/DAPI showed a positive correlation that increased with time irrespective of laser irradiation (Fig. 4C). However, the value of PCC increased in the presence of laser treatment, which was because the irradiation induced aldehyde production and enhanced micelle disassembly that was triggered by both hypoxia and irradiation. In contrast, PCC was relatively low (<0.3) under normoxia without laser irradiation (Fig. S10†), which was a consequence of simple drug release by diffusion without the contribution of triggered release mechanisms. When the laser was applied at the normal oxygen level, PCC dramatically increased because of the machinery of singlet oxygen-mediated micelle disassembly and rapid cargo release.
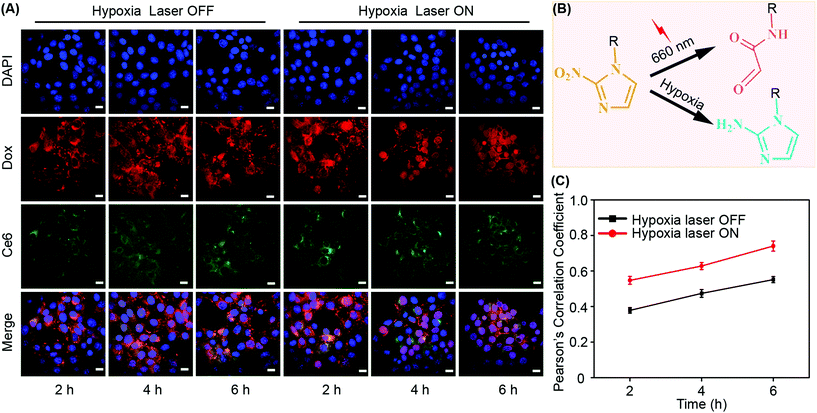 |
| Fig. 4 (A) Kinetic analysis of Dox and Ce6 fluorescence in 4T1 cells under hypoxia after incubation with micelles (NCs/Dox + Ce6) in the presence or absence of laser irradiation; (B) the mechanisms of triggered payload release from micelles; (C) the Pearson's correlation coefficient between Dox and DAPI under hypoxia with or without irradiation (n = 3). Scale bar: 20 μm (DAPI: Ex = 405 nm, Em = 415–475 nm; Dox: Ex = 488 nm, Em = 500–550 nm; Ce6: Ex = 640 nm, Em = 660–745 nm). | |
2.5 Enhanced apoptosis via triggered release
To assess the ability of co-delivery micelles (NCs/Dox + Ce6) in terms of singlet oxygen production, the commercial probe SOSG was employed. Irrespective of the oxygen level, the drug-loaded micelles could produce singlet oxygen upon laser treatment as evidenced by the green fluorescence of SOSG (Fig. 5A). Under normoxia, the Ce6 release was presumed via the NI/aldehyde-induced micelle disassembly. Under hypoxia with irradiation, both NI/aldehyde and NI/aminoimidazole mechanisms were responsive for payload release. The rapid release of Ce6 was essential for efficient singlet oxygen production due to the limited diffusion radius and short half-life of singlet oxygen.38–40 The discrepancy between the SOSG fluorescence intensity under hypoxia and that under normoxia was presumed mainly due to the difference in oxygen concentration. Under normoxia, the percentage of apoptosis (early plus late) induced by micelles (NCs/Dox + Ce6) was only ca. 19% in the absence of laser irradiation (Fig. 5B and C). Under the same laser-free conditions, this value doubled at 43% under hypoxia, which was believed as the hypoxia-induced rapid cargo release; in such a situation, Dox was the major active agent to induce cytotoxicity. In the presence of laser treatment under hypoxia, the extent of apoptosis increased to ca. 50%; in this situation, both release mechanisms should work to aid cargo release and both agents should exert cytotoxicity. In addition, the depletion of GSH would enhance the PDT effect of Ce6 and the formed aldehydes by NI oxidation would further decrease the cell viability as an auxiliary anti-tumor agent (Fig. 5D). As a positive control, the micelles under normoxia with laser irradiation could induce an apoptosis degree over 70%, for which both NI/aldehyde conversion-mediated payload release and the aldehyde cytotoxicity made a contribution. However, the PDT effect caused by Ce6 was the primary reason for high apoptosis percentage since oxygen is a key element of PDT.
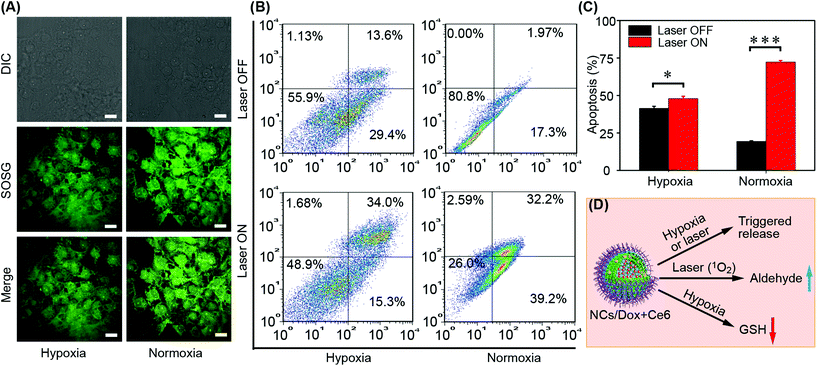 |
| Fig. 5 (A) Intracellular production of singlet oxygen in 4T1 cells by NCs/Dox + Ce6 micelles under hypoxia or normoxia with the aid of commercial probe SOSG. (B) Apoptotic cell populations determined by flow cytometric analysis of 4T1 cells incubated with NCs/Dox + Ce6 micelles under hypoxia or normoxia in the presence or absence of laser irradiation; (C) summary of the apoptosis extent. (D) Proposed mechanisms of micelle-induced apoptosis enhancement. Scale bar: 20 μm, *p < 0.05, ***p < 0.001. | |
2.6 Biodistribution
The drug-loaded micelles displayed good stability in different media including water, PBS, DMEM and serum (Fig. S11 and S12†). Although both Dox and Ce6 display intrinsic fluorescence, the latter Ce6 was selected as the probe to investigate the in vivo biodistribution of NCs/Dox + Ce6 micelles post-intravenous administration. This was mainly because of the longer excitation and emission wavelength of Ce6 (>600 nm) that made Ce6 an ideal candidate to image the location of micelles in mice. The formulation of the free drug (Dox + Ce6) with the same dose as those in micelles was used as the control to demonstrate the passive targeting ability of micelles. Both the micellar formulation and free drug combination showed a kinetic tumor deposition profile (Fig. 6A). At all four time points (4 h, 6 h, 8 h, and 24 h), the micellar nanocarrier could deliver more cargo to the tumor site in contrast to the control formulation, which was mainly an outcome of the enhanced permeability and retention (EPR) effect.41,42 Regarding the micelles, the EPR effect reached the peak 8 h post-dose administration, which was consistent with previous investigations (Fig. 6D).43 At the end of the biodistribution study, i.e. 24 h post-sample dosing, the fluorescence of both Ce6 and Dox in tumor as well as major healthy organs was obtained ex vivo (Fig. 6B and C). The content of both Ce6 and Dox in tumor was much higher for micellar formulations compared to the control (Fig. 6E and F) (p < 0.01). Despite the fact that micellar nanocarriers could aid the tumor targeting of encapsulated payloads, they could not avoid the distribution of formulations in healthy organs. This has been the origin of adverse effects of chemotherapy and related combination therapy. The complete eradication of side-effects of these therapies has been very challenging, but some recent advances in nanomedicine with triggered API activation open the door for efficient reduction of adverse effects.44,45
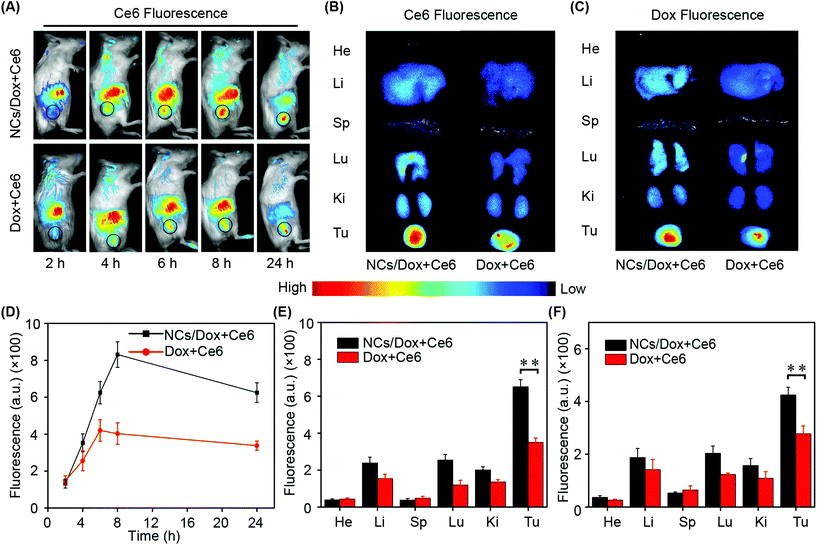 |
| Fig. 6 Biodistribution of micellar formulations. (A) In vivo kinetic fluorescence of Ce6 in 4T1 tumor-bearing mice upon intravenous administration of NCs/Dox + Ce6 micelles or free drug combination control (Dox + Ce6). Ex vivo fluorescent analysis of Ce6 (B) and Dox (C) in tumor (Tu) and major healthy organs (He: heart; Li: liver; Sp: spleen; Lu: lung; Ki: kidney) 24 h post-dose administration. (D) Kinetically semi-quantitative analysis of Ce6 fluorescence in tumors. Semi-quantitative fluorescence summary of Ce6 (E) and Dox (F) in tumors and other healthy organs 24 h post-dose administration (n = 3). Ce6: Ex = 660 nm, Em = 700–950 nm; Dox: Ex = 532 nm, Em = 560–750 nm. **p < 0.01. | |
2.7
In vivo antitumor efficacy
Continuous monitoring of the tumor volume has been a robust method for assessing the anti-tumor performance of various therapies (Fig. 7A). In the current study, phosphate buffered saline (PBS) was employed as the negative control. Regardless of the laser irradiation, both treatments by PBS resulted in almost identical tumor growth inhibition curves. In terms of the free drug combination (Dox + Ce6), the sample displayed a better anti-tumor behavior in the presence of irradiation in contrast to that without laser treatment (p < 0.001). This was because both Dox and Ce6 exerted toxicity upon the application of laser. However, when the laser is not present, only Dox worked for tumor elimination. Likewise, the micellar formulation (NCs/Dox + Ce6) also showed superior anti-tumor capability when the irradiation was turned on in contrast to that in the dark (p < 0.001). In the presence of laser treatment, the ability of micelles in inhibiting tumor growth was much better than the free drug (p < 0.001), which was a consequence of the EPR effect and enhanced tumor targeting by nanocarriers.41 This result was in good agreement with previous investigations of anti-tumor nanomedicine.21,24,46 The body weight of mice remained close to 20 gram for all groups during the course of treatment, indicating no significant adverse effects (Fig. 7B). In the end of the efficacy study, quantitative analysis of tumor mass ex vivo concurred well with the tumor inhibition curves (Fig. 7C). The excellent anti-tumor capability of NCs/Dox + Ce6 micelles was a collection of multiple factors, including passive tumor targeting, dually hypoxia- and singlet oxygen-triggered drug release, GSH depletion caused by NI bioreduction under hypoxia, and aldehyde production upon NI oxidation. Because the light trigger was only applied in the tumor site, there would be no aldehyde production in the normal organs.
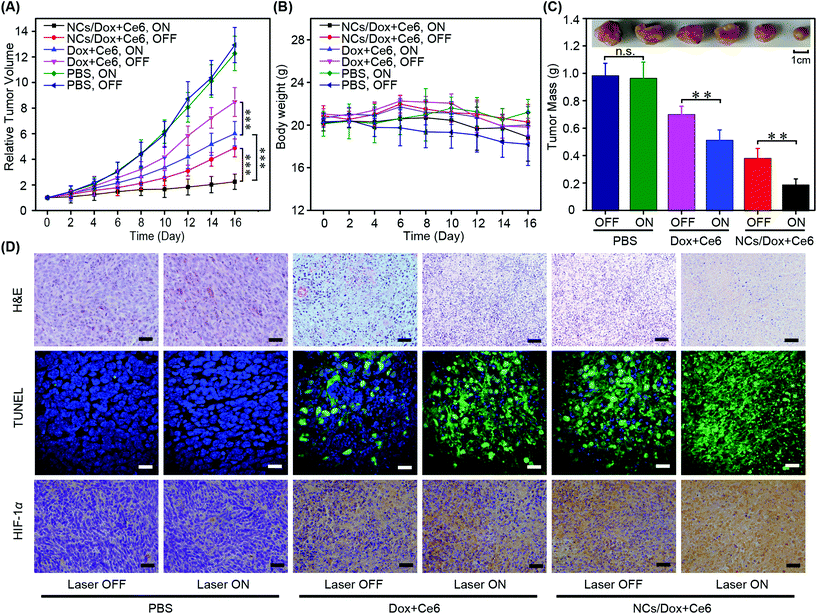 |
| Fig. 7
In vivo antitumor efficacy assessment of micelle and control formulations including NCs/Dox + Ce6, free drug (Dox + Ce6) and PBS in 4T1 tumor-bearing mice. (A) The tumor growth curves without (i.e. OFF) or with (i.e. ON) laser irradiation (8 h and 24 h post-intravenous dosing); (B) the body weight variation of 4T1 tumor-bearing mice during the period of treatment; (C) representative images of tumors collected from the mice in the end of treatment and quantitative analysis of tumor weight. (D) Analysis of tumor tissues via H&E staining, TUNEL staining, and HIF-1α staining in the end of therapy. All data were presented as mean ± standard deviation (n = 6). “n.s.” means no significant difference, **p < 0.01, ***p < 0.001, scale bar: 100 μm. | |
Histological analysis of tumor tissue by hematoxylin and eosin (H&E) staining revealed that the anti-tumor performance of formulations with laser irradiation ranked as follows: micelles > free drug > PBS (Fig. 7D). For each formulation, the presence of laser treatment resulted in increased toxicity. This trend was consistent in terms of the extent of apoptosis that was revealed by the terminal deoxynucleotidyl transferase mediated dUTP Nick end labeling (TUNEL) assay (Fig. 7D). In the end of the efficacy study, the intra-tumor level of hypoxia inducible factor-1α (HIF-1α) was demonstrated as the following ranking: micelles (laser ON) > micelles (laser OFF) > free drug (laser ON) > free drug (laser OFF) > PBS (laser ON) = PBS (laser OFF). The HIF-1α content was a good indicator of oxygen utilization, singlet oxygen generation, and hence tumor inhibition capability.24 All three types of staining agreed well with the tumor inhibitor curves, demonstrating the anti-tumor potency of multifunctional co-delivery NI-bearing conjugate micelles. The histological analysis of the major healthy organs in the end of the efficacy experiment demonstrated the absence of the adverse effect of conjugate micelles (Fig. S13†).
3. Conclusion
Taking advantage of the hypoxic microenvironment of solid tumor and the dual responsiveness of the NI moiety, we utilized the multifunctional NI-bearing polymer conjugate micelles for combinational delivery of chemotherapeutics and photosensitizers. The tailored micelles could deliver more cargos to the tumor site due to the passive targeting effect of nanocarriers. On-demand micelle disassembly and rapid cargo release were achieved in response to either hypoxia or laser irradiation. The hypoxia-induced drug release also caused GSH depletion that would enhance PDT efficacy. In addition, the singlet oxygen-triggered NI oxidation produced aldehydes that could induce supplementary anti-tumor effects. Because the hypoxia and laser irradiation were limited to the tumor site, the drug release in healthy organs was presumed slow and hence the side-effects caused by non-specific biodistribution could be minimized. The robust NI-bearing micelles offer a novel nanoplatform for the efficient co-delivery of photosensitizers and chemodrugs. Due to the limited tissue penetration of light, such micellar vehicles can also be used for the combination of chemotherapy and sonodynamic therapy for which ultrasound is employed for singlet oxygen production.
4. Experimental section
4.1 Materials
Chlorin e6 (Ce6) was purchased from Beijing JL Technology Co., Ltd (Beijing, China). Doxorubicin was purchased from Huateng Pharmaceutical Co., Ltd (Changsha, China). Methanol and acetonitrile were provided by Tianjin Concord Technology Co., Ltd. Sodium phosphate dibasic, dimethyl sulfoxide (DMSO) and citric acid were obtained from Tianjin Guangfu Fine Chemical Research Institute (Tianjin, China). 2,2′-Furil and ammonium acetate were purchased from Beijing J&K Scientific Co., Ltd (Beijing, China). 3-(4,5-Dimethyl-thiazol-2-yl)-2,5-diphenyl tetrazolium bromide (MTT, Sigma), Dulbecco's modified eagle's medium (DMEM) and fetal bovine serum (FBS) were sourced from Biological Industries (Israel). HIF-1α monoclonal antibody was sourced from Beyotime Biotechnology Co., Ltd (Shanghai, China). All other chemicals were purchased from Sigma Aldrich (Beijing, China).
4.2 Preparation of drug co-loaded micellar nanocarriers
The amphiphilic polymer (mPEG-PAsp-NI) was synthesized according to our recently published method.28 The preparation of placebo micelles used a dialysis method.28 The encapsulation of both drugs (Dox and Ce6) employed a similar approach. In brief, the polymer (200 mg), Dox (20 mg), and Ce6 (20 mg) were dissolved in 5 mL DMSO that was dialyzed against deionized water for 12 hours using the regenerated cellulose membrane (molecular weight cut-off/MWCO: 1000 Da), which was followed by centrifugation (2000g, 10 min) and then ultrafiltration (MWCO: 3000 Da). The retentate was collected and lyophilized to get the micellar nanocarrier (NCs/Dox + Ce6). The drug loading was determined using high performance liquid choreography (HPLC) using the previously published methods (n = 3).21,46
4.3 Characterization of micelles
The hydrodynamic sizes of micelles were determined using a Zetasizer Nano ZS (Malvern Instrument Ltd, Malvern, UK) at 25 °C with or without laser treatment (660 nm, 30 min, 100 mW cm−2) (n = 3). The drug release under sink conditions was carried out using static Franz cells with details described in our earlier publications.21,46 The effect of laser treatment on drug release was investigated and the irradiation details were 660 nm and 100 mW cm−2 for 10 min. Laser-free conditions were used as the control. The verification of oxamic aldehyde formation upon irradiation used the published protocol.28 The micelles (NCs/Dox + Ce6) were treated by the laser irradiation (660 nm, 100 mW cm−2, 30 min) at ambient temperature. Then 300 μL ammonium acetate (3.0 M) solution in glacial acetic acid and 800 μL 2,2′-furil (8.0 mM) solution in methanol were added. The mixture was kept at 90 °C for 30 min and then the emission spectrum was recorded by using a microplate reader at ambient temperature with an excitation wavelength of 250 nm. Methanol was used as the control for aldehyde detection. The micelle (1 mg mL−1) stability in three different media (water, PBS and DMEM) was assessed by constant monitoring of the hydrodynamic size (37 °C) over time (n = 3). The serum stability of NCs/Dox + Ce6 micelles was detected using a fluorescence approach. In brief, the micelles (NCs/DOX + Ce6) were dissolved in PBS containing 10% (v/v) FBS solution and the concentration was fixed at 1 mg mL−1 with the temperature being maintained at 37 °C. At the predetermined time points up to 48 h, the fluorescence intensity of Dox and Ce6 was recorded (Ex = 490 nm (Dox), Em = 585 nm (Dox); Ce6, Ex = 406 nm (Ce6), and Em = 650 nm (Ce6)). The UV-vis absorption and fluorescence emission spectra of NCs/Dox + Ce6 micelles in PBS (pH 7.4, 0.18 M) were recorded with placebo NCs, free Ce6 and free Dox as the control. The drug concentration was set at 1.0 μg mL−1 (Dox) and 0.7 μg mL−1 (Ce6). The excitation wavelength was 406 nm (Dox) and 490 nm (Ce6); the emission wavelength was 450–800 nm (Dox) and 520–800 nm (Ce6), respectively. Regarding the in vitro drug release under hypoxia, the drug-loaded micelles in PBS were incubated with a chemical mimic of nitroreductase, sodium dithionite (Na2S2O4) (0.4 mM). At predetermined time points, the absorption and emission spectra of both drugs were determined accordingly.
4.4 Glutathione assay
4T1 cells were obtained from the State Key Laboratory of Medicinal Chemical Biology, Nankai University. The cells were cultured in DMEM supplemented with 10% (w/v) FBS and 50 U mL−1 penicillin and 50 U mL−1 streptomycin (completed medium) in an incubator at 37 °C with a 5% CO2 atmosphere for normoxic conditions. The cells was cultured in a hypoxia modular incubator chamber (Billups-Rothenberg) supplemented with an appropriate humidified gas mixture (1% O2/5% CO2/balance N2) at 37 °C for hypoxic conditions. The effect of placebo micelle supplementation on the intracellular GSH concentration was analyzed using a commercial GSH assay kit (A006-2, Jiancheng bioengineering institute, China). Free NI was used as the control. The NI concentration in cells was fixed at 38.5 μg mL−1 for both samples. The GSH level was determined at 0 h, 2 h, and 4 h post-sample incubation under hypoxia. The detailed GSH and protein quantification procedures abided by the product protocol.
4.5 Singlet oxygen detection
The fluorescent singlet oxygen sensor green (SOSG) probe was used to detect in vitro singlet oxygen production upon micelle incubation followed by laser treatment. In brief, NCs/Dox + Ce6 micelles were dispersed in D2O (1 mg mL−1) and mixed with SOSG (10 μM). The samples were subjected to a laser beam (660 nm, 100 mW cm−2) for up to 10 min and the fluorescence intensities (Ex: 504 nm, Em: 525 nm) were recorded at 0.5, 1, 2, 3, 5 and 10 min post-irradiation by using a microplate reader (n = 3). The same probe was used to detect intracellular singlet oxygen production by micelles post-irradiation. The 4T1 cells were seeded in a glass cell culture dish (20 mm, NEST biotechnology Co., Ltd) at a density of 1 × 105 cells per dish in 1 mL medium for 24 h. Then l mL NCs/Dox + Ce6 micelle solution in the culture medium was added with a Ce6 concentration of 5 μg mL−1. The system was cultured under hypoxia or normoxia for 4 h, followed by PBS (pH 7.4, 0.18 M) washing in triplicate. Afterwards, SOSG was supplemented (40 μM), followed by laser treatment (660 nm, 100 mW cm−2, 5 min), and fluorescence imaging recording (Ex: 488 nm, Em: 500–550 nm).
4.6 Intracellular triggered drug release
The intracellular drug release upon hypoxia or irradiation triggering was analyzed by CLSM. Briefly, 4T1 cells were seeded in a glass cell culture dish (20 mm) at a density of 1 × 105 cells per dish containing 1 mL medium. After 24 h, the medium was replaced with a fresh one, and the micellar nanocarriers (NCs/Dox + Ce6) were supplemented to reach a final Ce6 concentration of 5 μg mL−1, followed by incubation at 37 °C for 4 h under hypoxia or normoxia. Then all groups of samples were treated by laser irradiation (660 nm and 100 mW cm−2) for 10 min, followed by culturing under the same hypoxic or normoxic conditions for different times. Irradiation-free samples were used as the controls. At the predetermined time point post-irradiation (2 h, 4 h, and 6 h), the cells were washed three times with ice-cold PBS (pH 7.4, 0.18 M), fixed with 4% paraformaldehyde for 20 min, and PBS washing again prior to DAPI staining for 10 min. The fluorescent images of cells were obtained by CLSM (UltraView Vox; PerkingElmer, Inc. USA). The excitation and emission wavelength of different molecules was listed as follows: DAPI (Ex = 405 nm, Em = 415–475 nm), Dox (Ex = 488 nm, Em = 500–550 nm), and Ce6: (Ex = 640 nm, Em = 660–745 nm). The Pearson's correlation coefficient between Dox and DAPI was used to assess the drug release behavior under different triggering conditions.
4.7 Apoptosis and cell viability
4T1 cells were seeded into 6-well plates with a density of 3 × 105 cells per well containing 2 mL medium. After 24 h, the medium was replaced with a fresh one, followed by micelle (NCs/Dox + Ce6) supplementation to reach a final Ce6 concentration of 5 μg mL−1. After 4 h's culturing under hypoxia or normoxia, laser irradiation was applied (660 nm, 100 mW cm−2, 10 min), followed by additional culturing under the same conditions for 24 h. Then the cells were digested with EDTA-free trypsin ready for apoptosis analysis using the Annexin V-FITC apoptosis detection kit (Yishen Biotech, China) using a BD Biosciences FACSCalibur flow cytometer. The cytotoxicity analysis of micellar formulations (NCs/Dox + Ce6) employed the standard MTT method.21 The free drug combination (Dox + Ce6) with the same drug ratio as that in micelles was used as the control. The starting 4T1 cell seeding density in 96-well plates was 4 × 103 cells per well. The samples were first incubated with the cells under hypoxia or normoxia for 4 h, followed by laser treatment (660 nm, 100 mW cm−2, and 10 min). Laser-free samples were used as controls. After 24 h's additional culturing under the same hypoxic or normoxic conditions, the cell viability was determined using the MTT assay protocol (n = 4). The half maximal inhibitory concentration (IC50) of two formulations under different conditions was calculated accordingly. As each sample contained two active drugs, two IC50 values were obtained for each formulation. The drug-free micelles were also assessed in terms of cytotoxicity in both 4T1 and 3T3 cells using the same MTT approach described above.
4.8 Biodistribution
All animal procedures were performed in accordance with the Guidelines for Care and Use of Laboratory Animals of Tianjin University and approved by the Animal Ethics Committee of Tianjin University. Female BALB/c mice (6 weeks, 16–19 g) were purchased from Vital River Laboratory Animal Technology Co. Ltd (Beijing, China). Each mouse was subcutaneously inoculated with 150 μL of the cell suspension on the right flank (3 × 106 4T1 cells per mouse). When the tumor size reached ca. 200 mm3, the 4T1 tumor-bearing mice were divided into two groups for the in vivo biodistribution study (n = 3). Two formulations (200 μL) including micelles (NCs/Dox + Ce6) and free drug (Dox + Ce6) were intravenously administered with an equivalent Ce6 dose of 4.0 mg per kg body weight; the concentration ratio between Dox and Ce6 was ca. 1.4. The fluorescence of Ce6 was used for micelle location tracking. At pre-designed time points (2 h, 4 h, 6 h, 8 h, and 24 h) post-dosing, the mice images were recorded using a Cri Maestro in vivo imaging system with Ce6 as the probe (Ex = 660 nm, Em = 700–950 nm). At the end of the distribution study (24 h post-dosing), both tumors and the major healthy organs (heart, liver, spleen, lung, kidney) were collected and imaged ex vivo with both Ce6 (Ex = 660 nm, Em = 700–950 nm) and Dox (Ex = 532 nm, Em = 560–750 nm) as the fluorescent probe, respectively (n = 3).
4.9
In vivo anti-tumor experiment
The same 4T1 tumor-bearing mice with a tumor volume of ca. 200 mm3 were used to assess antitumor efficacy of micelles (NCs/Dox + Ce6) with the free drug combination (Dox + Ce6) and PBS as the controls. The mice were randomized into six groups and each group contained six mice. The samples were categorized as follows: (1) micelles with irradiation; (2) micelles without irradiation; (3) free drug with irradiation; (4) free drug without irradiation; (5) PBS with irradiation; (6) PBS without irradiation. The Ce6 dose was set at 4.0 mg per kg body weight for both micelles and free drug combination with a constant Dox/Ce6 ratio of 1.4. All formulations were intravenously administered via the tail vein injection. At the pre-determined time points, i.e. 8 h and 24 h post-dosing, the laser irradiation was applied (660 nm, 100 mW cm−2, and 10 min) twice. The tumor size and mice body weight were monitored every two days after the initiation of treatment to plot the tumor inhibition cure and body weight variation curve. At the end of the efficacy experiment, the tumor tissues were collected, which was followed by histological (H&E) staining, apoptotic (TUNEL) staining, and immunohistochemical (HIF-1α) staining, respectively, using the commercial assay kits and published protocols.24 Meanwhile, the major healthy organs were also analyzed by the H&E staining in the end of the efficacy study for biocompatibility and adverse effect determination.
4.10 Statistical analysis
The data were manipulated as the average ± standard deviation. The Student's t-test or analysis of variance (ANOVA) coupled with Tukey's post-hoc analysis was used for significance comparison. The threshold of the significant difference between samples was set as p < 0.05.
Abbreviations
ANOVA | Analysis of variance |
APIs | Active pharmaceutical ingredients |
Ce6 | Chlorin e6 |
CLSM | Confocal laser scanning microscope |
DMEM | Dulbecco's modified Eagle's medium |
DMSO | Dimethyl sulfoxide |
Dox | Doxorubicin |
EPR | Enhanced permeability and retention |
FBS | Fetal bovine serum |
GSSG | Glutathione disulfide |
GSH | Glutathione |
GR | Glutathione reductase |
H&E | Hematoxylin and eosin |
HIF-1α | Hypoxia inducible factor-1α |
MWCO | Molecular weight cut-off |
MTT | 3-(4,5-Dimethylthiazol-2-yl)-2,5-diphenyltetrazolium bromide |
NADPH | Nicotinamide adenine dinucleotide phosphate |
NC | Nanocarrier |
NI | Nitroimidazole |
PAsp | Poly(aspartic acid) |
PEG | Poly(ethylene glycol) |
PBS | Phosphate buffered saline |
PCC | Pearson's correlation coefficient |
PDT | Photodynamic therapy |
ROS | Reactive oxygen species |
SOSG | Singlet oxygen sensor green |
TUNEL | Terminal deoxynucleotidyl transferase dUTP nick end labeling |
Author contributions
All authors have contributed to the manuscript writing and have approved the final version of the manuscript.
Conflicts of interest
The authors declare no competing financial interest.
Acknowledgements
This work was funded by the National Basic Research Program of China (2015CB856500) and the Tianjin Research Program of Application Foundation and Advanced Technology (18JCZDJC35700).
References
- R. M. Webster, Combination Therapies in Oncology, Nat. Rev. Drug Discovery, 2016, 15, 81–82 CrossRef CAS PubMed.
- J. H. Doroshow and R. M. Simon, On the Design of Combination Cancer Therapy, Cell, 2017, 171, 1476–1478 CrossRef CAS PubMed.
- Q. Hu, W. Sun, C. Wang and Z. Gu, Recent Advances of Cocktail Chemotherapy by Combination Drug Delivery Systems, Adv. Drug Delivery Rev., 2016, 98, 19–34 CrossRef CAS PubMed.
- R. Tao, M. Gao, F. Liu, X. Guo, A. Fan, D. Ding, D. Kong, Z. Wang and Y. Zhao, Alleviating the Liver Toxicity of Chemotherapy via pH-Responsive Hepatoprotective Prodrug Micelles, ACS Appl. Mater. Interfaces, 2018, 10, 21836–21846 CrossRef CAS PubMed.
- R. Misra and S. K. Sahoo, Coformulation of Doxorubicin and Curcumin in Poly(D,L-Lactide-co-Glycolide) Nanoparticles Suppresses the Development of Multidrug Resistance in K562 Cells, Mol. Pharm., 2011, 8, 852–866 CrossRef CAS PubMed.
- L. Liao, J. Liu, E. C. Dreaden, S. W. Morton, K. E. Shopsowitz, P. T. Hammond and J. A. Johnson, A Convergent Synthetic Platform for Single-Nanoparticle Combination Cancer Therapy: Ratiometric Loading and Controlled Release of Cisplatin, Doxorubicin, and Camptothecin, J. Am. Chem. Soc., 2014, 136, 5896–5899 CrossRef CAS PubMed.
- Y. Zhang, F. Huang, C. Ren, L. Yang, J. Liu, Z. Cheng, L. Chu and J. Liu, Targeted Chemo-Photodynamic Combination Platform Based on the DOX Prodrug Nanoparticles for Enhanced Cancer Therapy, ACS Appl. Mater. Interfaces, 2017, 9, 13016–13028 CrossRef CAS PubMed.
- X. Wei, L. Liu, X. Guo, Y. Wang, J. Zhao and S. Zhou, Light-Activated ROS-Responsive Nanoplatform Codelivering Apatinib and Doxorubicin for Enhanced Chemo-Photodynamic Therapy of Multidrug-Resistant Tumors, ACS Appl. Mater. Interfaces, 2018, 10, 17672–17684 CrossRef CAS PubMed.
- H. Lee, J. Han, H. Shin, H. Han, K. Na and H. Kim, Combination of Chemotherapy and Photodynamic Therapy for Cancer Treatment With Sonoporation Effects, J. Controlled Release, 2018, 283, 190–199 CrossRef CAS PubMed.
- S. Xu, X. Zhu, C. Zhang, W. Huang, Y. Zhou and D. Yan, Oxygen and Pt(II) Self-Generating Conjugate for Synergistic Photo-Chemo Therapy of Hypoxic Tumor, Nat. Commun., 2018, 9, 2053 CrossRef PubMed.
- D. E. Dolmans, D. Fukumura and R. K. Jain, Photodynamic Therapy for Cancer, Nat. Rev. Cancer, 2003, 3, 380–387 CrossRef CAS PubMed.
- E. Markovsky, H. Baabur-Cohen and R. Satchi-Fainaro, Anticancer Polymeric Nanomedicine Bearing Synergistic Drug Combination is Superior to a Mixture of Individually-Conjugated Drugs, J. Controlled Release, 2014, 187, 145–157 CrossRef CAS PubMed.
- H. Li, M. Li, C. Chen, A. Fan, D. Kong, Z. Wang and Y. Zhao, On-Demand Combinational Delivery of Curcumin and Doxorubicin via a pH-Labile Micellar Nanocarrier, Int. J. Pharm., 2015, 495, 572–578 CrossRef CAS PubMed.
- R. X. Zhang, H. L. Wong, H. Y. Xue, J. Y. Eoh and X. Y. Wu, Nanomedicine of Synergistic Drug Combinations for Cancer Therapy - Strategies and Perspectives, J. Controlled Release, 2016, 240, 489–503 CrossRef CAS PubMed.
- S. Shen, M. Liu, T. Li, S. Lin and R. Mo, Recent Progress in Nanomedicine-Based Combination Cancer Therapy Using a Site-Specific Co-Delivery Strategy, Biomater. Sci., 2017, 5, 1367–1381 RSC.
- V. P. Torchilin, Micellar Nanocarriers: Pharmaceutical Perspectives, Pharm. Res., 2007, 24, 1–16 CAS.
- Y. Shi, T. Lammers, G. Storm and W. E. Hennink, Physicochemical Strategies to Enhance Stability and Drug Retention of Polymeric Micelles for Tumor-Targeted Drug Delivery, Macromol. Biosci., 2017, 17, 1600160 CrossRef PubMed.
- D. Rosenblum, N. Joshi, W. Tao, J. M. Karp and D. Peer, Progress and Challenges towards Targeted Delivery of Cancer Therapeutics, Nat. Commun., 2018, 9, 1410 CrossRef PubMed.
- Y. Li, K. Xiao, W. Zhu, W. Deng and K. S. Lam, Stimuli-Responsive Cross-Linked Micelles for on-Demand Drug Delivery against Cancers, Adv. Drug Delivery Rev., 2014, 66, 58–73 CrossRef CAS PubMed.
- W. Xiao, N. Suby, K. Xiao, T. Y. Lin, A. N. Al, K. S. Lam and Y. Li, Extremely Long Tumor Retention, Multi-Responsive Boronate Crosslinked Micelles With Superior Therapeutic Efficacy for Ovarian Cancer, J. Controlled Release, 2017, 264, 169–179 CrossRef CAS PubMed.
- X. Li, M. Gao, K. Xin, L. Zhang, D. Ding, D. Kong, Z. Wang, Y. Shi, F. Kiessling, T. Lammers, J. Cheng and Y. Zhao, Singlet Oxygen-Responsive Micelles for Enhanced Photodynamic Therapy, J. Controlled Release, 2017, 260, 12–21 CrossRef CAS PubMed.
- D. Zhang, M. Wu, Z. Cai, N. Liao, K. Ke, H. Liu, M. Li, G. Liu, H. Yang, X. Liu and J. Liu, Chemotherapeutic Drug Based Metal-Organic Particles for Microvesicle-Mediated Deep Penetration and Programmable PH/NIR/Hypoxia Activated Cancer Photochemotherapy, Adv. Sci., 2018, 5, 1700648 CrossRef PubMed.
- X. Zhang, M. Wu, J. Li, S. Lan, Y. Zeng, X. Liu and J. Liu, Light-Enhanced Hypoxia-Response of Conjugated Polymer Nanocarrier for Successive Synergistic Photodynamic and Chemo-Therapy, ACS Appl. Mater. Interfaces, 2018, 10, 21909–21919 CrossRef CAS PubMed.
- J. Li, X. Meng, J. Deng, D. Lu, X. Zhang, Y. Chen, J. Zhu, A. Fan, D. Ding, D. Kong, Z. Wang and Y. Zhao, Multifunctional Micelles Dually Responsive to Hypoxia and Singlet Oxygen: Enhanced Photodynamic Therapy via Interactively Triggered Photosensitizer Delivery, ACS Appl. Mater. Interfaces, 2018, 10, 17117–17128 CrossRef CAS PubMed.
- F. Perche, S. Biswas, T. Wang, L. Zhu and V. P. Torchilin, Hypoxia-Targeted siRNA Delivery, Angew. Chem., Int. Ed., 2014, 53, 3362–3366 CrossRef CAS PubMed.
- P. Kulkarni, M. K. Haldar, S. You, Y. Choi and S. Mallik, Hypoxia-Responsive Polymersomes for Drug Delivery to Hypoxic Pancreatic Cancer Cells, Biomacromolecules, 2016, 17, 2507–2513 CrossRef CAS PubMed.
- C. Qian, J. Yu, Y. Chen, Q. Hu, X. Xiao, W. Sun, C. Wang, P. Feng, Q. D. Shen and Z. Gu, Light-Activated Hypoxia-Responsive Nanocarriers for Enhanced Anticancer Therapy, Adv. Mater., 2016, 28, 3313–3320 CrossRef CAS PubMed.
- L. Wang, X. Huang, B. Wang, J. Zhao, X. Guo, Z. Wang and Y. Zhao, Mechanistic Insight into the Singlet Oxygen-Triggered Expansion of Hypoxia-Responsive Polymeric Micelles, Biomater. Sci., 2018, 6, 1712–1716 RSC.
- A. J. Levine and A. M. Puzio-Kuter, The Control of the Metabolic Switch in Cancers by Oncogenes and Tumor Suppressor Genes, Science, 2010, 330, 1340–1344 CrossRef CAS PubMed.
- G. K. Balendiran, R. Dabur and D. Fraser, The Role of Glutathione in Cancer, Cell Biochem. Funct., 2004, 22, 343–352 CrossRef CAS PubMed.
- Y. Han, W. Yin, J. Li, H. Zhao, Z. Zha, W. Ke, Y. Wang, C. He and Z. Ge, Intracellular Glutathione-Depleting Polymeric Micelles for Cisplatin Prodrug Delivery to Overcome Cisplatin Resistance of Cancers, J. Controlled Release, 2018, 273, 30–39 CrossRef CAS PubMed.
- M. Gao, C. Chen, A. Fan, J. Zhang, D. Kong, Z. Wang and Y. Zhao, Covalent and Non-Covalent Curcumin Loading in Acid-Responsive Polymeric Micellar Nanocarriers, Nanotechnology, 2015, 26, 275101 CrossRef PubMed.
- A. Nunn, K. Linder and H. W. Strauss, Nitroimidazoles and Imaging Hypoxia, Eur. J. Nucl. Med., 1995, 22, 265–280 CrossRef CAS PubMed.
- J. Yu, Y. Zhang, Y. Ye, R. DiSanto, W. Sun, D. Ranson, F. S. Ligler, J. B. Buse and Z. Gu, Microneedle-Array Patches Loaded With Hypoxia-Sensitive Vesicles Provide Fast Glucose-Responsive Insulin Delivery, Proc. Natl. Acad. Sci. U. S. A., 2015, 112, 8260–8265 CrossRef CAS PubMed.
- H. He, R. Zhu, W. Sun, K. Cai, Y. Chen and L. Yin, Selective Cancer Treatment Via Photodynamic Sensitization of Hypoxia-Responsive Drug Delivery, Nanoscale, 2018, 10, 2856–2865 RSC.
- A. P. French, S. Mills, R. Swarup, M. J. Bennett and T. P. Pridmore, Colocalization of Fluorescent Markers in Confocal Microscope Images of Plant Cells, Nat. Protoc., 2008, 3, 619–628 CrossRef CAS PubMed.
- C. Chen, R. Tao, D. Ding, D. Kong, A. Fan, Z. Wang and Y. Zhao, Ratiometric Co-Delivery of Multiple Chemodrugs in a Single Nanocarrier, Eur. J. Pharm. Sci., 2017, 107, 16–23 CrossRef CAS PubMed.
- R. W. Redmond and I. E. Kochevar, Spatially Resolved Cellular Responses to Singlet Oxygen, Photochem. Photobiol., 2006, 82, 1178–1186 CrossRef CAS PubMed.
- T. Maisch, J. A. Baier, B. Franz, M. Maier, M. Landthaler, R. M. Szeimies and W. Baumler, The Role of Singlet Oxygen and Oxygen Concentration in Photodynamic Inactivation of Bacteria, Proc. Natl. Acad. Sci. U. S. A., 2007, 104, 7223–7228 CrossRef CAS PubMed.
- S. Hackbarth, J. Schlothauer, A. Preuss and B. Roder, New Insights to Primary Photodynamic Effects-Singlet Oxygen Kinetics in Living Cells, J. Photochem. Photobiol., B, 2010, 98, 173–179 CrossRef CAS PubMed.
- H. Maeda, J. Wu, T. Sawa, Y. Matsumura and K. Hori, Tumor Vascular Permeability and the EPR Effect in Macromolecular Therapeutics: a Review, J. Controlled Release, 2000, 65, 271–284 CrossRef CAS PubMed.
- Y. Matsumoto, J. W. Nichols, K. Toh, T. Nomoto, H. Cabral, Y. Miura, R. J. Christie, N. Yamada, T. Ogura, M. R. Kano, Y. Matsumura, N. Nishiyama, T. Yamasoba, Y. H. Bae and K. Kataoka, Vascular Bursts Enhance Permeability of Tumour Blood Vessels and Improve Nanoparticle Delivery, Nat. Nanotechnol., 2016, 11, 533–538 CrossRef CAS PubMed.
- E. Blanco, H. Shen and M. Ferrari, Principles of Nanoparticle Design for Overcoming Biological Barriers to Drug Delivery, Nat. Biotechnol., 2015, 33, 941–951 CrossRef CAS PubMed.
- B. T. Castle and D. J. Odde, Optical Control of Microtubule Dynamics in Time and Space, Cell, 2015, 162, 243–245 CrossRef CAS PubMed.
- C. Chen, J. Zhao, M. Gao, X. Meng, A. Fan, Z. Wang and Y. Zhao, Photo-Triggered Micelles: Simultaneous Activation and Release of Microtubule Inhibitors for On-demand Chemotherapy, Biomater. Sci., 2018, 6, 511–518 RSC.
- K. Xin, M. Li, D. Lu, X. Meng, J. Deng, D. Kong, D. Ding, Z. Wang and Y. Zhao, Bioinspired Coordination Micelles Integrating High Stability, Triggered Cargo Release, and Magnetic Resonance Imaging, ACS Appl. Mater. Interfaces, 2017, 9, 80–91 CrossRef CAS PubMed.
Footnotes |
† Electronic supplementary information (ESI) available: Supplementary figures including conjugate structure, micelle stability, absorption and emission spectra of micelles, in vitro drug release and singlet oxygen production, fluorescent detection of the oxamic aldehyde, cytotoxicity of placebo micelles, kinetic drug release from micelles in 4T1 cells under normoxia, histological analysis of normal organs, and summary table of cell viability data. See DOI: 10.1039/c8bm01042k |
‡ J. Deng and F. Liu made an equal contribution to this work. |
|
This journal is © The Royal Society of Chemistry 2019 |