DOI:
10.1039/C9AY00175A
(Paper)
Anal. Methods, 2019,
11, 1331-1337
Label-free and sensitive detection of coralyne and heparin based on target-induced G-quadruplex formation†
Received
22nd January 2019
, Accepted 5th February 2019
First published on 6th February 2019
Abstract
Herein we propose a label-free and sensitive detection method for coralyne and heparin, based on utilizing the complex of adenosine16 (A16) and coralyne to induce the formation of a G-quadruplex scaffold. In the present work, two specific oligonucleotide strands were designed. Upon addition of coralyne, the two oligonucleotide strands formed a double chain through A2–coralyne–A2 coordination, which induced the formation of an intermolecular G-quadruplex accompanied by the proximity of two G-rich sequences. An increase in system fluorescence was observed after the addition of N-methylmorpholine (NMM). In the presence of heparin, the formation of the heparin–coralyne complex caused coralyne to be removed from the A16–coralyne complex, leading to a weaker fluorescence output. At optimal concentrations, a linear coralyne response could be observed from 0.01–5 μM and the detection limit was estimated to be 5.8 nM. The detection limit of the heparin probe was as low as 1 nM, with a linear range from 1–100 nM. Overall, this study offers a sensitive and practical assay for coralyne and heparin detection.
1. Introduction
Functional nucleic acids, such as G-quadruplexes, i-motifs, catalytic nucleic acids, triplexes, aptamers and Holliday junctions, belong to a family of nucleic acids that have been applied for the detection of various targets, from small molecules to proteins and DNAs.1–4 For example, Liu et al. reported a quencher-free fluorescence method for mercury(II) detection utilizing T–Hg2+–T interaction.5 Meanwhile, Guo et al. designed a universal fluorometric aptasensor for the detection of bisphenol A.6 In the study of functional nucleic acids, adenine-rich nucleic acids, especially polydeoxyadenylic acids (poly(dA)), have attracted significant attention in recent years because of their important role in biological processes.7 Meanwhile, various studies have shown that numerous poly(dA) tracts can exclude nucleosomes and assist in the transportation of proteins, influencing the structure of chromatin. Ultimately, this may induce cancers with the overexpression of neopolyadenosine [poly(A)] polymerase.8 As a consequence, more attention has been devoted in recent years to exploring potential drugs that can bind to the poly(dA) sequence.9
As a compound type with a crescent shape, coralyne has attracted much attention for the use in bioanalysis and medicinal chemistry due to its low toxicity and extensive biological activity. Furthermore, coralyne has been reported to show significant antitumor activity in animal models via intercalation with DNA.10–12 Recently, various research groups have shown that poly(dA) can form a tight connection with coralyne, with a binding constant of 1.05 × 105 M−1, leading to the formation of anti-parallel homoadenine duplex DNA chains.13 Based on the importance of coralyne in biological fields, a series of detection methods for coralyne have been proposed over the past few decades, including dual polarization interferometry, fluorescence analysis, gel electrophoresis, multi-spectroscopic techniques, metal nanoparticle-based colorimetry and surface plasmon resonance techniques.14–17 However, some practical drawbacks of these assays include time-consuming processes and the generally costly and complicated operation. This ultimately limits the overall applicability. Therefore, the development of a simple and sensitive detection strategy for coralyne remains a critical, albeit unmet, scientific goal.
Heparin, a linear polysaccharide exhibiting high electronegativity, plays a crucial role in regulating various physiological activities, including cell growth, metabolism and immune defense.18–21 Besides, heparin is also used as an anticoagulant to prevent blood coagulation in clinical applications via reaction with antithrombin III.22–24 However, the manifestation of some disease states can be observed when the heparin concentration in human serum is too high.25–27 Therefore, the continued search for new assays to monitor the content variation of heparin in serum is critically important.28–30 For example, Thirupathi et al. designed a new type of peptide-based sensor to detect the concentration of heparin in aqueous solutions and in serum with very high sensitivity. However, the use of this sensor type generally requires the use of expensive equipment and an overall complicated process.31 Liu and coworkers developed a rapid and simple detection assay for heparin detection based on CuInS2 quantum dots with higher limits of detection.32 However, a simple, rapid and sensitive detection method is still necessary that could be used in a clinical environment.
N-Methylmorpholine (NMM) is a fluorescent dye that has been widely used in biological detection owing to fluorescence reaction with a certain type of G-rich DNA sequence (G-quadruplex DNA).33 Specifically, NMM can generate a dramatic fluorescence intensity enhancement after linking with G-quadruplex DNA.34,35 Based on this principle, NMM has been successfully used for the detection of a series of biological materials, such as small molecules, metal ions, DNA, enzymes and proteins.36–40
Herein, we report a label-free and sensitive detection assay based on intermolecular G-quadruplex formation to monitor the concentration variation of coralyne and heparin. Two designed DNA sequences were used and linked with each other to form a double-stranded DNA via A2–coralyne–A2 coordination. The latter species promoted the formation of an intermolecular G-quadruplex, resulting in strong fluorescence intensity with the addition of NMM. Moreover, in the presence of heparin, heparin could react with coralyne and prevent fluorescence increase. Overall, the proposed method may offer potential applications for coralyne and heparin detection in clinical research.
2. Experimental
2.1 Materials and methods
Coralyne, heparin, ethidium bromide (EB), berberine, methyl red, glucose, glycine and adenosine triphosphate (ATP) were purchased from Sigma-Aldrich. Tris base, potassium chloride (KCl), magnesium chloride (MgCl2), N-methylmorpholine (NMM), hyaluronic acid (HA) and sodium chloride (NaCl) were obtained from Sinopharm Chemical Reagent. Ultrapure water (18.2 MΩ cm) used throughout all experiments was obtained from a Milli-Q water purification system (Millipore Corp, Bedford, MA, USA). The oligonucleotides used throughout the experiments were synthesized by Sangon Biological Engineering Technology & Services Co., Ltd. (Shanghai, China). The DNA sequences used were as follows: T1: gggtagggcgggaaaaaaaaaaaaaaaa; T2: aaaaaaaaaaaaaaaaggg. The reaction buffer was Tris–HCl buffer (50 mM NaCl and 10 mM MgCl2, pH 7.5). The fluorescence measurements with excitation at 399 nm were performed at room temperature on an F-2700 spectrophotometer (Hitachi, Japan), and the emission spectra were collected in the range of 600–640 nm. 10 nm and 5 nm were set as the excitation slit and emission slit widths, respectively.
2.2 Optimization of reaction conditions
In order to find the best reaction conditions, various reaction conditions, such as the concentrations of T1, T2, K+ and NMM and the reaction time of NMM and coralyne, were optimized. For optimizing the concentration of T1, different concentrations of T1 ranging from 30 to 200 nM with 200 nM T2 and 10 μM coralyne were added to the reaction buffer for 20 min at 30 °C. Then, 2.5 μM NMM and 10 μM K+ were mixed with the reaction mixture and incubated for another 30 min at 37 °C. Finally, the fluorescence intensity was measured on an F2700 spectrophotometer. In the same way, the other conditions were optimized, i.e., the concentration of T2 ranged from 50 to 400 nM, the concentration and reaction time of NMM were, respectively, from 1.5 to 3.5 μM and 5 to 50 min, the K concentration range was 4–20 μM and the reaction time of coralyne was 0–50 min. The final reaction volume of all samples was 100 μL and each experiment was repeated three times.
2.3 Coralyne assays
For assaying different concentrations of coralyne, various amounts of coralyne were first mixed with 100 nM T1 and 200 nM T2 in 10 mM Tris–HCl buffer (50 mM NaCl and 10 mM MgCl2, pH 7.5) for 20 min at 30 °C. Then, 10 μM K+ and 2.5 μM NMM were added to the reaction system. Finally, using an F-2700 fluorescence spectrophotometer, the fluorescence intensity of the reaction mixture was measured after reaction for 30 min at 37 °C.
With 5 μM EB, berberine and methyl red in place of the different concentrations of coralyne, the specificity of the system could be validated. The final volume of the reaction system was 100 μL.
2.4 Heparin assays
In a typical heparin assay, different concentrations of heparin with 8 μM coralyne were mixed in 10 mM Tris–HCl buffer (50 mM NaCl and 10 mM MgCl2, pH 7.5) for 10 min at room temperature. Then, 100 nM T1 and 200 nM T2 were added and the mixture was incubated at 30 °C for 20 min. After reaction completion, 10 μM K+ and 2.5 μM NMM were added to the reaction system for incubation for 30 min at 37 °C. An F-2700 fluorescence spectrophotometer was used to measure the fluorescence intensity and the final volume of the reaction system was 100 μL. In order to verify the specificity of the experiment, 500 nM HA, glucose, ATP, glycine and heparin were added to the reaction system.
2.5 Application in real samples
A 100-fold diluted human serum sample was collected from a healthy adult in 10 mM Tris–HCl as the reaction buffer to detect the concentration of heparin in a real sample. Before the actual detection procedures were carried out, various concentrations of heparin were added to the mixture and the resulting mixture was allowed to mix at room temperature for 5 min. 20 nM, 40 nM and 60 nM were detected as the final concentrations of heparin and each experiment was repeated for a total of three times. Human serum samples were collected from Xiangya Hospital, Central South University. The study was approved by the Ethics Committee of Central South University, Changsha, China. All volunteers gave informed consent. We declare that the experiments performed in this study comply with the current laws of the People's Republic of China.
3. Results and discussion
3.1 Sensing mechanism
Scheme 1 describes the principle of the detection method for coralyne and heparin. As a proof-of-concept, we synthesized two special DNA strands, T1 and T2. In the green parts, T1 possessed three GGG repeat units and T2 possessed one GGG repeat unit. In the red sections, an adenine-rich sequence (A16 sequence) could be observed, which can form a tight connection with coralyne by A2–coralyne–A2 coordination. In the absence of coralyne, the two strands were unable to form a double chain without any self-structure pair. And the formation of an intramolecular G-quadruplex needs a nucleic acid strand possessing four, or more than four, poly(dG) domains. However, since there are only three poly(dG) domains in T1 and one poly(dG) domain in T2, it is hard for them to form the G-quadruplexes by themselves, resulting in very low fluorescence intensity generation even after addition of NMM. In the presence of coralyne, which can recognize and bind with the A16 sequence, T1 and T2 were able to form the homo-adenine DNA duplex through the interaction between coralyne and adenine. When the G-rich sequences of T1 and T2 come close to each other, an intermolecular G-quadruplex can be easily formed. As a result, the intermolecular G-quadruplex structure was able to bind with NMM and generate a remarkable fluorescence enhancement. In contrast, upon addition of heparin, coralyne could be adsorbed which prevents any potential ligation reaction between coralyne and the A16 sequence. As a consequence, almost no fluorescence intensity could be detected.
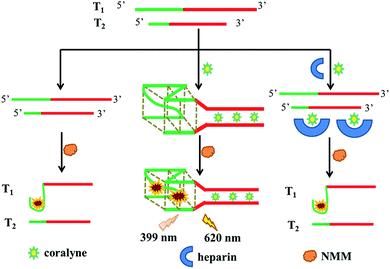 |
| Scheme 1 Schematic representation of coralyne and heparin detection based on G-quadruplex formation. | |
3.2 Feasibility of the assays
We assessed the feasibility for the detection of coralyne and heparin. T1, T2, K+ and NMM were incubated at 37 °C for 30 min and almost no fluorescence could be observed at this point, which proved that T1 or T2 can't form a G-quadruplex structure by themselves without the presence of coralyne (Fig. 1A). After the addition of coralyne to the mixture, T1 and T2 can form a DNA duplex via A2–coralyne–A2 mismatch to generate an intermolecular G-quadruplex structure and result in strong fluorescence emission (Fig. 1B). Then, coralyne was adsorbed by heparin before reacting with T1 and T2, resulting in a low fluorescence intensity (Fig. 1C). All results demonstrated the feasibility of our method for heparin and coralyne detection.
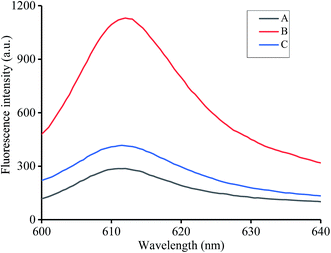 |
| Fig. 1 Fluorescence spectra at different conditions: (A) 200 nM T1, 200 nM T2, and 5 μM NMM; (B) 200 nM T1, 200 nM T2, 10 μM coralyne, and 5 μM NMM; (C) 200 nM T1, 200 nM T2, 10 μM coralyne, 10 μM heparin, and 5 μM NMM. | |
3.3 Optimization of reaction conditions
As shown in Fig. S1 and S2,† the concentration of T1 and T2 was fixed at 100 nM and 200 nM, respectively. Fig. S3† indicates that an NMM concentration of 2.5 μM was the most suitable reaction concentration. We selected a K+ concentration of 10 μM as highlighted in the results shown in Fig. S4.† 20 min was determined to be the most suitable reaction time (Fig. S5†). Finally, the optimized NMM reaction time was determined to be 30 min (Fig. S6†).
3.4 Sensitivity to coralyne
In order to verify the sensitivity of the proposed assay to coralyne, we added various coralyne concentrations ranging from 0 to 10 μM (0, 0.01, 0.1, 0.5, 1, 2, 3, 4, 5, 6, 8, and 10 μM) to the buffer containing K+, T1 and T2 at 30 °C for 20 min. The fluorescence of the mixture was then measured after addition of NMM and incubation for 30 min. As shown in Fig. 2A, an increasing amount of T1 and T2 could link with each other via the formation of an A2–coralyne–A2 mismatch upon increasing the coralyne concentration. This indicates that more intermolecular G-quadruplex structures could be formed resulting in higher fluorescence intensity with the addition of NMM. Therefore, an obvious fluorescence enhancement could be observed as shown in Fig. 2B. In the inset of Fig. 2B, a linear relationship (R2 = 0.9933) between fluorescence intensity and coralyne concentration ranging from 0.01–5 μM could be determined and the detection limit was 5.8 nM according to the 3σ criterion. The latter finding was indeed better than most of the values reported for other coralyne assay methods currently available (Table S1†).
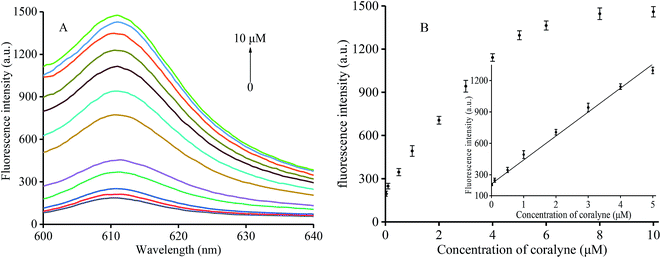 |
| Fig. 2 (A) Fluorescence spectra of the reaction mixture with increasing coralyne concentration from 0 to 10 μM. (B) Relationship between fluorescence intensity (610 nM) and coralyne concentration. The inset shows the linearity of fluorescence intensity with respect to coralyne concentration. Error bars were estimated from three replicate measurements. | |
3.5 Sensitivity to heparin
Under optimum conditions, different fluorescence intensities were detected in the presence of heparin at various concentrations ranging from 0 to 450 nM (0, 1, 5, 10, 30, 50, 80, 100, 200, 300, 400, and 450 nM). The corresponding results are shown in Fig. 3A. We found reduced fluorescence intensity upon increasing the heparin concentration. When the heparin concentrations were between 1 and 100 nM, the fluorescence data showed a linear R2 = 0.9947 (Fig. 3B). A concentration of 1 nM was determined to be the detection limit according to the 3σ criterion, a value that was found to be improved over that of other assay methods (Table 1).
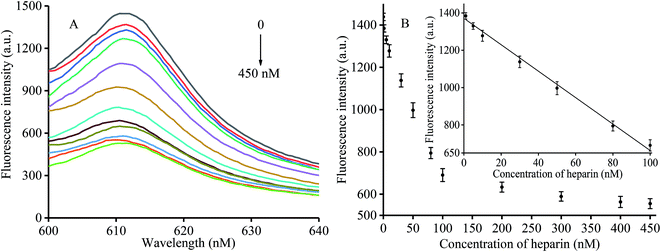 |
| Fig. 3 (A) Fluorescence spectra of the reaction mixture with increasing heparin concentration from 0 to 450 nM. (B) Relationship between fluorescence intensity (610 nM) and heparin concentration. The inset shows the linearity of fluorescence intensity with respect to heparin concentration. Error bars were estimated from three replicate measurements. | |
Table 1 Comparison of different methods for the determination of heparin
Method |
Material |
Detection range (nM) |
LOD (nM) |
Reference |
Colorimetric |
Silver nanoparticles |
4.4–22 |
1.6 |
21
|
Colorimetric |
Gold nanoparticles |
10–400 |
2.5 |
38
|
Rayleigh scattering |
Phloxine B |
8.3–1250 |
4.2 |
22
|
Fluorescence |
Fluorescent peptidyl probe |
2.6–150 |
2.6 |
20
|
Fluorescence |
Pyrene derivatives |
100–2300 |
140 |
26
|
Fluorescence |
Quantum dots |
50–1500 |
12.5 |
29
|
Fluorescence |
Four-armed AIE fluorescent sensor |
2.99–167 |
2.99 |
30
|
Fluorescence |
NMM |
1–100 |
1 |
This work |
3.6 Selectivity of coralyne and heparin activity assays
To evaluate the specificity of the method for coralyne detection, we selected three competing compounds, namely EB, berberine and methyl red. The experiment was designed in an effort to test which compound exhibited similar properties to coralyne. As shown in Fig. S7,† only the mixture containing 5 μM coralyne exhibited high fluorescence intensity, the other compounds at a concentration of 5 μM resulted in negligible fluorescence intensity similar to the blank. Therefore, our assay exhibited a high selectivity for the detection of coralyne.
In order to verify the specificity of the method for heparin detection, several different compounds, including hyaluronic acid (HA), glucose, ATP and glycine, were mixed into the reaction system under optimized conditions. No other compounds, except heparin, reacted with coralyne, which failed to prevent the formation of an intermolecular G-quadruplex. Therefore, higher fluorescence intensity could be observed as shown in Fig. 4 except for the experiment sample with added heparin. The results demonstrated the high specificity of the assay for the determination of heparin.
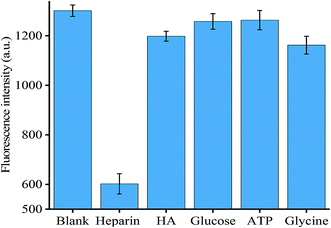 |
| Fig. 4 Specificity of heparin detection. Error bars were estimated from three replicate measurements. | |
3.7 Application in real sample
To demonstrate the analytical applicability of the proposed method in a real sample, 100-fold diluted human serum from a healthy adult was added to Tris–HCl buffer to create a new reaction buffer. Then, different concentrations of heparin (20 nM, 40 nM and 60 nM) were added to the reaction buffer and the mixture was allowed to react for 5 min at room temperature. Table 2 shows that the recovery rates of the three concentrations of heparin were 99.3% for 20 nM, 98.2% for 40 nM and 101.2% for 60 nM. The results obtained suggest that the proposed method was useful and could be applied to the analysis of a real sample.
Table 2 Detection of heparin in a real sample (n = 3)
Sample |
Added (nM) |
Found (nM) |
Recovery (%) |
R.S.D (%) |
Sample 1 |
20 |
19.86 ± 0.86 |
99.3 |
4.36 |
Sample 2 |
40 |
39.28 ± 2.07 |
98.2 |
5.14 |
Sample 3 |
60 |
60.69 ± 2.74 |
101.2 |
4.21 |
4. Conclusions
In conclusion, we introduced a label-free and simple fluorescence assay method for the highly sensitive detection of coralyne and heparin utilizing the specific complex of A2–coralyne–A2 to induce the formation of an intermolecular G-quadruplex, resulting in high fluorescence intensity after adding NMM. The study method exhibited a low detection limit for both coralyne and heparin. Additionally, the proposed method was simple to carry out and cost-effective for the use in the detection of coralyne and heparin with only two DNA probes and NMM. Furthermore, the application of the assay method in a real sample showed that there was no need for any other complex instruments, and thus this strategy may feature a wide range of applications. Furthermore, the proposed assay is expected to be useful in the study of coralyne or heparin and may further provide a new strategy for advanced drug therapy.
Conflicts of interest
There are no conflicts of interest to declare.
Acknowledgements
This work was supported by the National Natural Science Foundation of China (No. 21205142), State Key Laboratory of Chemo/Biosensing and Chemometrics, Hunan University (2017006), and Research Innovation Program for Graduates of Central South University (2018zzts384, 2018zzts399).
References
- S. Zhan, Y. Wu, L. Wang, X. Zhan and P. Zhou, A mini-review on functional nucleic acids-based heavy metal ion detection, Biosens. Bioelectron., 2016, 86, 353–368 CrossRef CAS PubMed.
- M. Yüce, N. Ullah and H. Budak, Trends in aptamer selection methods and applications, Analyst, 2015, 140, 5379–5399 RSC.
- A. Alsaafin and M. Mckeague, Functional nucleic acids as in vivo metabolite and ion biosensors, Biosens. Bioelectron., 2017, 94, 94–106 CrossRef CAS PubMed.
- D. Kong, N. Wang, X. Guo and H. Shen, ‘Turn-on’ detection of Hg2+ ion using a peroxidase-like split G-quadruplex hemin DNAzyme, Analyst, 2010, 135, 545–549 RSC.
- H. Liu, L. Ma, C. Ma, J. Du, M. Wang and K. Wang, Quencher-free fluorescence method for the detection of mercury (II) based on polymerase-aided photoinduced electron transfer strategy, Sensors, 2016, 16, 1945 CrossRef PubMed.
- L. Guo, Y. Hu, Z. Zhang and Y. Tang, Universal fluorometric aptasensor platform based on water-soluble conjugated polymers/graphene oxide, Anal. Bioanal. Chem., 2018, 410, 287–295 CrossRef CAS PubMed.
- T. Hou, X. Wang, X. Liu, S. Du and F. Li, A label-free and colorimetric turn-on assay for coralyne based on coralyne-induced formation of peroxidase-mimicking split DNAzyme, Analyst, 2013, 138, 4728–4731 RSC.
- T. Hou, C. Li, X. Wang, C. Zhao and F. Li, Label-free colorimetric detection of coralyne utilizing peroxidase-like split G-quadruplex DNAzyme, Anal. Methods, 2013, 5, 4761–4764 Search PubMed.
- P. Zhang, Y. Wang, F. Leng, Z. Xiong and C. Huang, Highly selective and sensitive detection of coralyne based on the binding chemistry of aptamer and graphene oxide, Talanta, 2013, 112, 117–122 CrossRef CAS PubMed.
- J. Oh, S. Han, H. Park and J. Lee, Room-temperature colorimetric detection of coralyne using DNA-functionalized nanoparticle probes, Bull. Korean Chem. Soc., 2012, 33, 329–332 CrossRef CAS.
- S. Kumari, A. Badana, G. Mohan, G. Shailender Naik and R. Malla, Synergistic effects of coralyne and paclitaxel on cell migration and proliferation of breast cancer cells lines, Biomed. Pharmacother., 2017, 91, 436–445 CrossRef CAS PubMed.
- K. Park and H. Park, A DNA-templated silver nanocluster probe for label-free, turn-on fluorescence-based screening of homo-adenine binding molecules, Biosens. Bioelectron., 2015, 64, 618–624 CrossRef CAS PubMed.
- Y. Wang, J. Wang, F. Yang and X. Yang, Probing biomolecular interactions with dual polarization interferometry: real-time and label-free coralyne detection by use of homoadenine DNA oligonucleotide, Anal. Chem., 2012, 84, 924–930 CrossRef CAS PubMed.
- Y. Shi, Y. Chen, W. Hu and L. Chang, Detection of Naja atra cardiotoxin using adenosine -based molecular beacon, Toxins, 2017, 9, 24 CrossRef PubMed.
- H. Huang, S. Shin, X. Zheng and T. Yao, Sensitive detection for coralyne and mercury ions based on homo-A/T DNA by exonuclease signal amplification, Biosens. Bioelectron., 2015, 71, 439–444 CrossRef CAS PubMed.
- K. Padmapriy and R. Barthwal, Binding of the alkaloid coralyne to parallel G-quadruplex DNA [d(TTGGGGT)]4 studied by multi-spectroscopic techniques, Biophys. Chem., 2016, 219, 49–58 CrossRef PubMed.
- Z. Lv, H. Wei, B. Li and E. Wang, Colorimetric recognition of the coralyne–poly(dA) interaction using unmodified gold nanoparticle probes, and further detection of coralyne based upon this recognition system, Analyst, 2009, 134, 1647–1651 RSC.
- U. Warttinger, C. Giese, J. Harenberg, E. Holmer and R. Krämer, A fluorescent probe assay (Heparin Red) for direct detection of heparins in human plasma, Anal. Bioanal. Chem., 2016, 408, 8241–8251 CrossRef CAS PubMed.
- H. Jiang, G. Wang and X. Zhang, Detection of heparin based on the conformational switch of DNA, Anal. Methods, 2015, 7, 7852–7857 RSC.
- J. You, Y. Wang and W. Tseng, Adenosine-related compounds as an enhancer for peroxidase-mimicking activity of nanomaterials: application to sensing of heparin level in human plasma and total sulfate glycosaminoglycan content in synthetic cerebrospinal fluid, ACS Appl. Mater. Interfaces, 2018, 10, 37846–37854 CrossRef CAS PubMed.
-
S. Kumar, P. Bhushan and S. Bhattacharya, Positively charged silver nanoparticles as labels for paper-based colorimetric detection of heparin, IFMBE Proceeding, 2017, vol. 67, pp. 235–240 Search PubMed.
- Y. Ling, Z. Gao, Q. Zhou, N. Li and H. Luo, Multidimensional optical sensing platform for detection of heparin and reversible molecular logic gate operation based on the phloxine B/polyethyleneimine system, Anal. Chem., 2015, 87, 1575–1581 CrossRef CAS PubMed.
- B. Casu, A. Naggi and G. Torri, Re-visiting the structure of heparin, Carbohydr. Res., 2015, 403, 60–68 CrossRef CAS PubMed.
- Y. Wang and W. Tseng, Surfen-assembled graphene oxide for fluorescence turn-on detection of sulfated glycosaminoglycans in biological matrix, ACS Sens., 2017, 2, 748–756 CrossRef CAS PubMed.
- P. Thirupathi, J. Park, L. Neupane, M. Kishore and K. Lee, Pyrene excimer-based peptidyl chemosensors for the sensitive detection of low levels of heparin in 100% aqueous solutions and serum samples, ACS Appl. Mater. Interfaces, 2015, 7, 14243–14253 CrossRef CAS PubMed.
- Y. Qiao, Z. Yao, W. Ge, L. Zhang and H. Wu, Rapid and visual detection of heparin based on the disassembly of polyelectrolyte-induced pyrene excimers, Org. Biomol. Chem., 2017, 15, 2569–2574 RSC.
- H. Lee, B. In, P. Mehta, M. Kishore and K. Lee, Dual role of a fluorescent peptidyl probe based on self-assembly for the detection of heparin and for the inhibition of the heparin digestive enzyme reaction, ACS Appl. Mater. Interfaces, 2018, 10, 2282–2290 CrossRef CAS PubMed.
- P. Mehta, H. Lee and K. Lee, Highly sensitive ratiometric detection of heparin and its oversulfated chondroitin sulfate contaminant by fluorescent peptidyl probe, Biosens. Bioelectron., 2017, 91, 545–552 CrossRef CAS PubMed.
- C. Kuoa and W. Tseng, Adenosine-based molecular beacons as light-up probes for sensing heparin in plasma, Chem. Commun., 2013, 49, 4607–4609 RSC.
- Y. Gao, K. Wei, J. Li, Y. Li and J. Hu, A facile four-armed AIE fluorescent sensor for heparin and protamine, Sens. Actuators, B, 2018, 277, 408–414 CrossRef CAS.
- P. Thirupathi, L. Neupane and K. Lee, Fluorescent peptide-based sensors for the ratiometric detection of nanomolar concentration of heparin in aqueous solutions and in serum, Anal. Chim. Acta, 2015, 873, 88–98 CrossRef CAS PubMed.
- Z. Liu, Q. Ma, X. Wang, Z. Lin, H. Zhang, L. Liu and X. Su, A novel fluorescent nanosensor for detection of heparin and heparinase based on CuInS2 quantum dots, Biosens. Bioelectron., 2014, 54, 617–622 CrossRef CAS PubMed.
- F. Khusbu, X. Zhou, H. Chen, C. Ma and K. Wang, Thioflavin T as a fluorescence probe for biosensing applications, Trends Anal. Chem., 2018, 109, 1–18 CrossRef.
- P. Zhang, H. Liu, S. Ma, S. Men, Q. Li, X. Yang, H. Wang and A. Zhang, A label-free ultrasensitive fluorescence detection of viable Salmonella enteritidis using enzyme-induced cascade two-stage toehold strand-displacement-driven assembly of G-quadruplex DNA, Biosens. Bioelectron., 2016, 80, 538–542 CrossRef CAS PubMed.
- D. Ji, H. Meng, J. Ge, L. Zhang, H. Wang, D. Bai, J. Li, L. Qu and Z. Li, Ultrasensitive fluorometric glutathione assay based on a conformational switch of a G-quadruplex mediated by silver(I), Microchim. Acta, 2017, 9, 3325–3332 CrossRef.
- K. Wu, C. Ma, H. Zhao, M. Chen and Z. Deng, Sensitive aptamer-based fluorescene assay for ochratoxin A based on RNase H signal amplification, Food Chem., 2019, 277, 273–278 CrossRef CAS PubMed.
- M. Li, A. Zhao, J. Ren and X. Qu, N-methyl mesoporphyrin IX as an effective probe for monitoring Alzheimer's disease amyloid aggregation in living cells, ACS Chem. Neurosci., 2017, 8, 1299–1304 CrossRef CAS PubMed.
- F. Qu, Y. Liu, H. Lao, Y. Wang and J. You, Colorimetric detection of heparin with high sensitivity based on the aggregation of gold nanoparticles induced by polymer nanoparticles, New J. Chem., 2017, 41, 10592–10597 RSC.
- K. Wu, C. Ma, H. Zhao, H. He and H. Chen, Label-Free G-Quadruplex Aptamer Fluorescence Assay for Ochratoxin A Using a Thioflavin T Probe, Toxins, 2018, 10, 198 CrossRef PubMed.
- K. Wu, C. Ma, Z. Deng, N. Fang, Z. Tang, X. Zhu and K. Wang, Label-free and nicking enzyme-assisted fluorescence signal amplification for RNase H analysis based on a G-quadruplexe/thioflavin T complex, Talanta, 2018, 182, 142–147 CrossRef CAS PubMed.
Footnote |
† Electronic supplementary information (ESI) available. See DOI: 10.1039/c9ay00175a |
|
This journal is © The Royal Society of Chemistry 2019 |
Click here to see how this site uses Cookies. View our privacy policy here.