DOI:
10.1039/C8AN01626G
(Paper)
Analyst, 2019,
144, 191-196
Two-photon imaging of the endoplasmic reticulum thiol flux in the brains of mice with depression phenotypes†
Received
21st August 2018
, Accepted 22nd October 2018
First published on 23rd October 2018
Abstract
Depression is a common mental illness with high morbidity and mortality. Mounting evidence suggests that an imbalance of the oxidant–antioxidant defence system is strongly correlated with depression and the dysfunction of the endoplasmic reticulum (ER) is strongly related to the oxidative stress. Therefore, as vital and abundant antioxidants in the ER, biothiols may contribute to the etiology of depression. However, ideal two-photon (TP) fluorescent probes for in vivo imaging of ER-associated thiols in the brains of mice with depression phenotypes are still lacking. Hence, we describe a fluorescent probe (ER-SH) to visualize thiols in living systems. ER-SH displays high sensitivity, excellent ER-targeting ability, outstanding TP properties and low cytotoxicity. Using this ER-SH probe, we succeeded in revealing an increase in the endogenous thiol levels under ER stress induced by DTT. Significantly, TP in vivo imaging showed for the first time that the thiol levels are reduced in brains of mice with depression phenotypes. Collectively, this work can assist in further understanding the molecular mechanism of depression and offers a crucial dimension for diagnosis and anti-depression treatments.
Introduction
Major depression, one of the most frequently encountered forms of mental illness and a leading cause of disability worldwide, has a notably high morbidity and mortality rate.1,2 However, understanding of the pathophysiology of depression is still rudimentary.3 Previous findings suggest that an imbalance of oxidant and antioxidant defence systems contributes to the pathogenesis of depression.4–6 Intracellular thiols such as cysteine (Cys), homocysteine (Hcy), and glutathione (GSH) help in maintaining the higher-order structures of proteins and control redox homeostasis via the equilibrium between thiols and disulphides (RSSR). As a vital antioxidant and the most abundant biothiol in cells, GSH is closely related to oxidative stress.7,8 Studies have shown that ER stress is closely relevant to oxidative stress.9,10 Therefore, monitoring of the reductive small-molecule thiols of the ER in the brains of mice with depression phenotypes has important implications for understanding the physiological and pathological processes of depression.
Due to its high spatiotemporal resolution, fluorescence imaging is increasingly recognized as a promising and powerful tool for monitoring thiols in living cells and in vivo.11–14 Two-photon microscopy (TPM) offers deeper tissue penetration, higher spatial resolution with minimum background emission, and a longer observation time than one-photon (OP) imaging.15–17 Given the complex construction of the brain, TP fluorescence imaging is expected to be more appropriate for in vivo brain imaging.18,19 Nevertheless, ideal TP fluorescent probes for in vivo imaging of thiols in the ER with superior optical performance are still lacking.
Thus, we designed an ER-targeted TP fluorescent probe known as ER-SH for imaging of thiols in living cells and in vivo. ER-SH consists of three components. In consideration of the excellent two-photon properties and high quantum yield, 1,8-naphthalene dimethylamine was chosen as the fluorophore,20,21 and 2,4-dinitrobenzene sulphonamide (DNBS), the well-known thiol-specific recognition site, was selected as the reporter group (Scheme 1).22–25 Methyl benzene sulphonamide, as we previously reported, is used as an ER-targeting moiety.26 The fluorescence of the 1,8-naphthalene dimethylamine fluorophore is effectively quenched by DNBS through the photo-induced electron transfer (PET) mechanism. Once the DNBS is hydrolysed by thiols, the PET process is terminated, and the fluorescence is restored. Experimental results demonstrate that ER-SH possesses high sensitivity and selectivity to thiols and a remarkable ER-targeting ability. Using this probe, we visualized thiols in PC12 cells and the brains of mice with depression phenotypes.
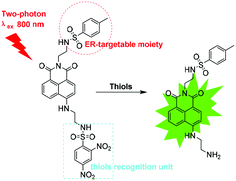 |
| Scheme 1 Structures of ER-SH and the corresponding reaction mechanism. | |
Experimental
Apparatus and reagents
Details on the apparatus and reagents used in the studies can be found in the ESI.†
Kinetic studies
The kinetic profiles of the reaction were generated under pseudo-first order conditions with a large excess of Cys, GSH and Hcy (100 equiv.) over the ER-SH probe at room temperature. The pseudo-first-order rate constants k′ for the reaction were calculated to be 4.73 × 10−4 S−1, 2.40 × 10−4 S−1 and 2.38 × 10−4 S−1 for ER-SH according to eqn (1): | ln [(Fmax − Ft)/Fmax] = −k′t | (1) |
where Ft and Fmax are the fluorescence intensities at time t and the maximum value obtained after the reaction was complete (535 nm for ER-SH). k′ is the pseudo-first-order rate constant.
Synthesis
Compound 1 was synthesized in our previous report.27
ER-SH: Under the Ar gas conditions, compound 1 (0.453 g, 1.0 mmol) and compound 2 (0.267 g, 1.0 mmol) were dissolved in 10 mL of dichloromethane. The mixture was stirred at 25 °C for 2 h. Then the solvents were removed, and the residue was purified by silica gel chromatography with dichloromethane/methanol (30
:
1 v/v) to give ER-SH as a light yellow solid (0.18 g, 40%). 1H NMR (400 MHz, d6-DMSO) δ(ppm): δ 8.58 (s, 1H), 8.49 (s, 1H), 8.40 (d, J = 8.2 Hz, 1H), 8.32 (d, J = 7.2 Hz, 1H), 8.15–8.10 (m, 2H), 8.05 (d, J = 8.7 Hz, 1H), 7.76 (t, J = 6.2 Hz, 1H), 7.61 (d, J = 15.9 Hz, 4H), 7.27 (d, J = 8.1 Hz, 2H), 6.71 (d, J = 8.7 Hz, 1H), 4.05 (dd, J = 15.5, 8.4 Hz, 4H), 3.54–3.49 (m, 2H), 3.07–2.98 (m, 2H), 2.28 (s, 3H); 13C NMR (100 MHz, d6-DMSO) δ(ppm): 163.78, 163.12, 150.41, 148.85, 147.30, 143.04, 138.30, 138.02, 134.34, 131.50, 131.13, 129.92, 129.64, 128.67, 127.42, 126.72, 124.46, 122.05, 120.29, 119.98, 108.39, 104.18, 42.50, 41.64, 39.31, 29.19, 22.57, 21.36, 14.39. HRMS (ESI) m/z calcd for C29H26N6O10S2 [M + Na+]: 705.1044, found 705.1040.
Ethical statement
Adult male C57BL/6J mice (age: 6 weeks; average body weight: 18 ± 2 g) were purchased from the Experimental Animal Center of Shandong University (Jinan, PR China). All animal care and experimental protocols complied with the Animal Management Rules of the Ministry of Health of the People's Republic of China and were approved by the Animal Care Committee of Shandong Normal University.
Results and discussion
The photophysical properties of the probe ER-SH
The synthesis of compound ER-SH is shown in Scheme 2. The spectroscopic properties of the compound and its fluorescence responses to Cys were evaluated in detail.
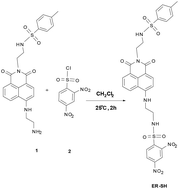 |
| Scheme 2 Design and synthesis of ER-SH. | |
We first assessed the spectral response to thiols under physiological conditions (10 mM HEPES buffer, pH 7.4). The absorption spectra were measured, and the probe displays a distinct absorption maximum peak at 420 nm, as shown in Fig. S1 of the ESI.† The absorption intensity at 420 nm increased slightly in the absence or presence of Cys. Additionally, upon addition of different concentrations of Cys, the fluorescence intensity of ER-SH at 535 nm increased dramatically (Fig. 1A). The fluorescence quantum yield increased from 0.008 to 0.39 in the probe blank or in the presence of Cys. In addition, the two-photon absorption cross-section was 85 GM. Moreover, linear regressions were obtained between the fluorescence intensity at 535 nm and the Cys concentration in the range of 0–400 μM with a detection limit of 1.67 × 10−7 M (Fig. 1B). Under these same conditions, ER-SH exhibited different fluorescence responses to GSH and Hcy with detection limits of 4.70 × 10−6 M and 9.62 × 10−7 M, respectively (Fig. S2†). The intracellular concentration of Cys ranges from 30 to 200 μM, the GSH concentration is about 1–10 mM, and Hcy in living cells is about 1.05 μM.28–30 All these physiological concentration ranges are well within the ER-SH detection threshold of the low and high concentration ranges. In brief, ER-SH shows excellent sensitivity to thiols.
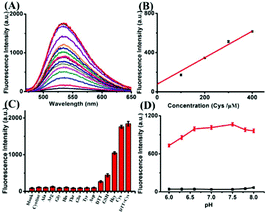 |
| Fig. 1 Fluorescence response and selectivity of ER-SH. (A) Fluorescence spectra of 10 μM ER-SH with different concentrations of Cys (0–200 equiv.). (B) Linear plot of 10 μM ER-SH with different concentrations of Cys (0–400 μM). (C) Fluorescence responses of ER-SH (10 μM) reacted with other amino acids and other stimulants: blank, cystine (100 μM), Ala (100 μM), Arg (100 μM), Gly (100 μM), His (100 μM), Thr (100 μM), Glu (100 μM), Tyr (100 μM), Asp (100 μM), DTT (2 mM), GSH (2 mM), Hcy (2 mM), Cys (2 mM), and DTT + Cys (2 mM + 2 mM). (D) Fluorescence responses of ER-SH (10 μM) before and after the addition of Cys (100 equiv.) at different pH values (black line and red line, respectively). All of the spectra were acquired in 10 mM HEPES buffer (pH 7.4) at λex = 420 nm and λem = 535 nm. Error bars are ± SD (n = 5). | |
Subsequently, to examine the selectivity, the ER-SH probe was treated with various biologically relevant species. As shown in Fig. 1C, the fluorescence intensity of ER-SH displayed almost no enhancement in the presence of various amino acids (cystine, Ala, Arg, Gly, His, Thr, Glu, Tyr, Asp and DTT). Similarly, we found no fluorescence intensity change when treated with common metal ions (Na+, K+, Mn2+, Cu2+, Al3+, Fe3+, Mg2+, Zn2+, and Ca2+), reductants (citric acid, Na2S2O3), ROS (H2O2, 1O2, and TBHP) and RNS (NO, ONOO−) (Fig. S3A†). However, an obvious increase in the fluorescence intensity was observed only on adding Cys, Hcy and GSH, verifying that ER-SH could be used to selectively detect these three thiols. Moreover, to test the H2O2-dependent thiol homeostasis, we used the probe to study the changes in the level of Cys in the presence of H2O2 over time. The fluorescence intensity of ER-SH showed no noticeable change when H2O2 was added (Fig. S3B†), indicating a decrease of the Cys level. This result testifies that the probe can indicate the slow oxidation of thiol. Additionally, the fluorescence responses of ER-SH to Cys under different pH values were determined. The pH values did not affect the fluorescence response of ER-SH to thiols, and the response was especially better under physiological conditions (Fig. 1D). Furthermore, time-dependent fluorescence spectra of ER-SH with Cys, Hcy and GSH were studied. The pseudo-first-order rate constants were calculated as k′1 = 4.73 × 10−4 S−1, k′2 = 2.40 × 10−4 S−1, and k′3 = 2.38 × 10−4 S−1 for Cys, Hcy and GSH, respectively, with 100 equiv. at 535 nm (Fig. 2). Collectively, ER-SH is highly selective to these three thiols and possesses excellent photostability (Fig. S4†). Further experiments indicated that ER-SH had almost no cytotoxicity (Fig. S5†). All of these data suggest that ER-SH is a suitable sensor for exclusive imaging of thiols under physiological conditions.
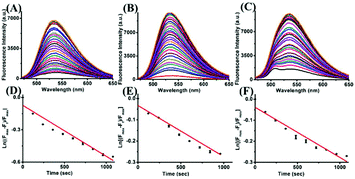 |
| Fig. 2 Fluorescence responses of 10 μM ER-SH to 100 equiv. Cys (A), Hcy (B) and GSH (C) with excitation at different times, respectively. Spectra were recorded every two minutes (0–50 min). The pseudo-first-order rate constants k′ for ER-SH were obtained from the slope of the plot of ln [(Fmax – Ft)/Fmax] against time (excitation wavelength at 420 nm and emission wavelength at 535 nm). Error bars are ± SD (n = 5). | |
The subcellular localization experiment of the probe ER-SH
To explore whether ER-SH can be applied in cellular imaging, the subcellular localization of ER-SH was investigated in live HL7702 cells. The fluorescence of ER-SH was well co-localized with that of commercially available ER-tracker Red (Fig. 3A and B). The overlap coefficient is 0.92, demonstrating that ER-SH exhibited excellent ER-targeting ability. To further test the ER-targeting ability of the probe, we performed z-stacks (z = 0–8 μm) in the co-localization experiment. As shown in Fig. 3C, the fluorescence of ER-SH still overlaps with that of the commercial dye when the z-axis changes. Furthermore, the intrinsic ER-targeting ability of ER-SH was also found in 4T1 cells (Fig. S6†). These data verify that ER-SH has admirable ER targetability, which is expected to facilitate imaging of thiols in the ER.
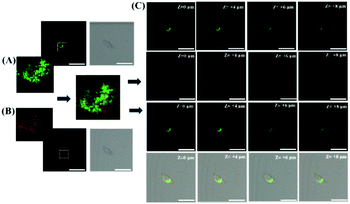 |
| Fig. 3 Co-localization imaging of ER-SH (20 μM) with commercial ER-Tracker Red dye (500 nM) in HL7702 cells. (A) ER-SH is the green channel, λex = 405 nm, collected at 500–550 nm. (B) ER-Tracker Red is the red channel, λex = 561 nm, collected 590–650 nm. (C) Z-stacks are 0 μm, +4 μm, +6 μm, and +8 μm. Scale bar: 50 μm. | |
Exogenous thiol imaging in live cells with ER-SH
Inspired by the favourable results of live-cell staining with the ER-SH probe, we explored TP imaging for exogenous thiols. When PC12 cells were pretreated with Cys (200 μM) and Hcy (200 μM), the cells exhibited extraordinarily bright fluorescence (average 2.3-fold) (Fig. 4). To confirm that the increase in fluorescence is caused by thiols, the cells were incubated with N-ethylmaleimide (NEM, a thiol blocking agent).31 Obviously weaker fluorescence was observed (4.4-fold), indicating a decrease in the concentration of thiols. In addition, we noticed that the fluorescence intensity decreased 1.8-fold when H2O2 was added in living cells. The results suggest that thiols are oxidized by H2O2. Moreover, a similar experimental result was discovered for zebrafish with 2.1-fold decrease in fluorescence compared with the control (Fig. S7A†). Besides, the fluorescence in the zebrafish gradually increased with the increase in the ER-SH incubation time, reaching the maximum fluorescence at 1 h. Then, it stabilized at 2 h, 12 h, and 48 h (Fig. S7B†). We found that the fluorescence decreased with time till becoming faint at 2 h (Fig. S7C†). This indicates the good absorption and metabolism of ER-SH, which is essential for bioimaging. In summary, ER-SH is an excellent fluorescent probe that can detect the thiol levels in living cells and zebrafish.
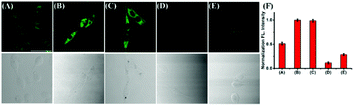 |
| Fig. 4 TP imaging of Cys and Hcy with ER-SH in PC12 cells. (A) The cells were incubated only with the probe ER-SH (20 μM) for 30 min. (B) and (C) Cells were incubated with Cys (200 μM) and Hcy (200 μM) for 30 min, and then treated with ER-SH (20 μM) for another 30 min. (D) The cells were incubated with NEM (1 mM), and then incubated with ER-SH (20 μM) for another 30 min. (E) The cells were incubated with H2O2 (200 μM) for 30 min, and then treated with ER-SH (20 μM) for another 30 min. The second line contains bright-field images. (F) Normalized fluorescence intensities of PC12 cells in images A–E under different conditions. The two-photon excitation wavelength was at 800 nm and emission windows: 500–600 nm. Scale bar: 20 μm. | |
Endogenous thiol imaging in live cells with ER-SH
Studies have shown that the ER stress increases with significant correlation with oxidative stress, which inspired us to visualize the flux of endogenous thiols in live cells under ER stress. Dithiothreitol (DTT) is a thiol reductant and a protective agent that can disturb the protein's disulfide bonds and induce ER stress.32 In order to study the flux of thiols in live cells at the earlier stage of ER stress, we first incubated the cells with the probe for 30 minutes, and then stimulated with DTT over time. Subsequently, we acquired the fluorescence in PC12 cells, and observed that the fluorescence intensity gradually enhanced till approximately 20 min, and then seemed to be stable. In addition, we found consistent results in the fluorescence imaging of DTT-treated 4T1 cells (Fig. S8†). Next, to investigate whether prolonged ER stress can lead to decrease in thiol oxidation, we observed the fluorescence intensity of ER-SH in three cell groups. As Fig. 5 shows, the control group was incubated only with ER-SH for 30 min. The second and third groups were pretreated with DTT for 60 min or 120 min to induce prolonged ER stress, and then incubated with ER-SH for imaging. The fluorescence of the second and third groups was weaker than that of the control group, indicating the oxidation of the thiol. This result unraveled that the fluctuation of thiols in ER was presumably due to the over-production of ROS under prolonged ER stress, causing the imbalance of redox.26 Collectively, the probe is demonstrated to satisfactorily image endogenous thiols in the ER under DTT stimuli.
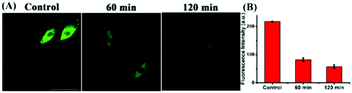 |
| Fig. 5 TP fluorescence images of PC12 cells. (A) The control group was incubated with ER-SH (20 μM) for 30 min. The second group was pre-treated with DTT (5 mM) for 60 min, and then incubated with ER-SH (20 μM) for another 30 min. The third group was pre-treated with DTT (5 mM) for 120 min, and then incubated with ER-SH (20 μM) for another 30 min. (B) Relative fluorescence intensities of PC12 cells in image (A). The two-photon excitation wavelength was at 800 nm and emission windows: 500–600 nm. Scale bar: 20 μm. | |
The two-photon fluorescence imaging of thiols in mice
To test the ability of ER-SH to recognize thiols in vivo, we noticed an average 3.4-fold fluorescence enhancement when mice were injected with exogenous thiols in the abdomen (Fig. S9†). Furthermore, we applied it to the detection of thiols in the abdomen of mice under ER stress. The control group mice were only injected with ER-SH (100 μM) for 15 minutes. DTT group mice were pre-treated with DTT (10 mM) for 4 hours and injected with ER-SH (100 μM) for 15 minutes. As shown in Fig. 6, the fluorescence intensity in the tissue of DTT-treated mice was obviously higher than that in control mice, indicating that the DTT-treated mice possessed higher thiol levels (3.5-fold). To further confirm that the fluorescence enhancement was based on the burst of thiols, the mice were pre-treated with NEM. The mice were pre-treated with NEM (1 mM) for 4 hours and injected with ER-SH for 15 minutes. These mice displayed a weaker fluorescence intensity compared with the control mice (3.3-fold), demonstrating the reduction of thiols under stimulation by NEM. This result demonstrates that ER-SH could be used to image the thiol levels in vivo.
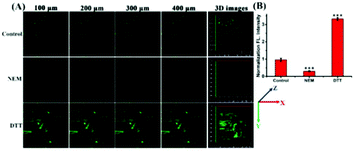 |
| Fig. 6 TP fluorescence imaging of the abdomen of mice. (A) TP imaging at different depths and 3D images of the abdomen of mice treated with ER-SH (100 μM). Control (n = 5), NEM-induced mice (n = 5) and DTT-induced mice (n = 5). (B) Normalized fluorescence intensity statistics were output by selecting five representative regions. Relative fluorescence intensities of 3D images of living tissue in (A). Data are expressed as the mean ± S.D., ***P < 0.001 for NEM, DTT vs. control. The two-photon laser was at 800 nm, and fluorescence was collected from 500 to 600 nm. Scale bar = 50 μm. | |
Motivated by the successful application of ER-SH in the abdomen of mice, we applied this construct to gain an insight into the level of thiols in the brains of mice with depression phenotypes. Findings suggested that an imbalance of oxidant and antioxidant defence systems contributes to the pathogenesis of depression. Prolonged oxidative stress always accompanies a decline of endogenous anti-oxidants. Hence, we explored the level of thiols in the brains of mice exposed to chronic unpredictable mild stress (CUMS). The control group and the CUMS group were injected with ER-SH (100 μM). After 10 min, we performed TP fluorescence imaging of the brains of CUMS and normal mice by TP confocal microscopy. In the brains of these mice (Fig. 7B), we found a 2.6-fold decrease in fluorescence compared with those of the normal mice (Fig. 7A), validating the reduction of thiols in the brains of mice with depression phenotypes. Importantly, the results supply relevant evidence for the negative correlation between the level of thiols and the depression status, supporting the role of oxidative damage in the occurrence and development of depression. These data further illustrate that ER-SH is an ideal TP fluorescence imaging tool for visualization of thiols in the brain of mice with depression phenotypes.
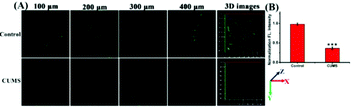 |
| Fig. 7 TP fluorescence imaging in the brain of mice with depression phenotypes. (A) TP fluorescence imaging at different depths and 3D images of the brain of mice treated with ER-SH (100 μM) via i.p. injection. Control (n = 5) and CUMS (n = 5). (B) Normalized fluorescence intensity statistics were output by selecting five representative regions. Relative fluorescence intensities of 3D images of living brains in (A). Data are expressed as the mean ± S.D., ***P < 0.001 for CUMS vs. control. Fluorescence images were obtained with an 800 nm light source. Fluorescence emission windows: 500–600 nm. Scale bar = 50 μm. | |
Conclusions
In conclusion, we present an ER-targeting fluorescent probe known as ER-SH. The probe can respond to thiols sensitively and selectively. ER-SH also exhibits excellent photostability, low cytotoxicity and insensitivity to pH changes. In view of those favorable properties, ER-SH was successfully used to examine thiol variations under ER stress through TP fluorescence imaging. Furthermore, ER-SH was used to selectively and sensitively recognize the reduction of thiols in the brains of mice with depression-like behaviors for the first time, uncovering the connection between ER stress and depression based on thiols in an unprecedented manner. This work can assist in further understanding the molecular mechanism of depression and offers a crucial dimension for diagnosis and anti-depression treatments.
Conflicts of interest
There are no conflicts to declare.
Acknowledgements
This work was supported by the National Natural Science Foundation of China (21535004, 91753111, 21675105, 21475079, and 21390411) and the Natural Science Foundation of Shandong Province of China (ZR2017ZC0225).
Notes and references
- R. C. Kessler, P. Berglund, O. Demler, R. C. Kessler, P. Berglund, O. Demler, R. Jin, K. R. Merikangas and E. E. Walters, Arch. Gen. Psychiatry, 2005, 62, 593–602 CrossRef PubMed.
- E. J. Nestler, M. Barrot, R. J. DiLeone, A. J. Eisch, S. J. Gold and L. M. Monteggia, Neuron, 2002, 34, 13–25 CrossRef CAS PubMed.
- V. Krishnan and E. Nestler, Nature, 2008, 455, 894–902 CrossRef CAS PubMed.
- M. Maesa, P. Galeckib, Y. S. Changc and M. Berkd, Prog. Neuro-Psychopharmacol., 2011, 35, 676–692 CrossRef PubMed.
- S. D. Khanzode, G. N. Dakhale, S. S. Khanzode, A. Saoji and R. Palasodkar, Redox Rep., 2003, 8, 365–370 CrossRef CAS PubMed.
- M. Bilicia, H. Efeb, M. A. Köroğlua, H. A. Uydub, M. Bekaroğlua and O. Değerb, Affective Disord., 2001, 64, 43–51 CrossRef.
- C. S. Lim, G. Masanta, H. J. Kim, J. H. Han, H. M. Kim and B. R. Cho, J. Am. Chem. Soc., 2011, 133, 11132–11135 CrossRef CAS PubMed.
- S. V. Mulay, Y. Kim, M. Choi, D. Y. Lee, J. Choi, Y. Lee, S. Jon and D. G. Churchill, Anal. Chem., 2018, 90, 2648–2654 CrossRef CAS PubMed.
- F. Urano, X. Wang, A. Bertolotti, Y. Zhang, P. Chung, H. P. Harding and D. Ron, Science, 2000, 287, 664–666 CrossRef CAS PubMed.
- J. T. Coyle and P. Puttfarcken, Science, 1993, 262, 689–695 CrossRef CAS PubMed.
- A. C. Sedgwick, J. E. Gardiner, G. Kim, M. Yevglevskis, M. D. Lloyd, A. T. A. Jenkins, S. D. Bull, J. Yoon and T. D. James, Chem. Commun., 2018, 54, 4786–4789 RSC.
- C. Yin, F. Huo, J. Zhang, R. Martínez-Máñez, Y. Yang, H. Lv and S. Li, Chem. Soc. Rev., 2013, 42, 6032–6059 RSC.
- X. Chen, Y. Zhou, X. Peng and J. Yoon, Chem. Soc. Rev., 2010, 39, 2120–2135 RSC.
- V. S. Lin, W. Chen, M. Xian and C. J. Chang, Chem. Soc. Rev., 2015, 44, 4596–4618 RSC.
- H. W. Liu, X. B. Zhang, J. Zhang, Q. Q. Wang, X. X. Hu, P. Wang and W. Tan, Anal. Chem., 2015, 87, 8896–8903 CrossRef CAS PubMed.
- F. Helmchen and W. Denk, Nat. Methods, 2005, 2, 932–940 CrossRef CAS PubMed.
- W. R. Zipfel, R. M. Williams and W. W. Webb, Nat. Biotechnol., 2003, 21, 1369–1377 CrossRef CAS PubMed.
- H. M. Kim and B. R. Cho, Chem. Rev., 2015, 115, 5014–5055 CrossRef CAS PubMed.
- H. M. Kim and B. R. Cho, Acc. Chem. Res., 2009, 42, 863–872 CrossRef CAS PubMed.
- Z. R. Dai, G. B. Ge, L. Feng, J. Ning, L. H. Hu, Q. Jin, D. D. Wang, X. Lv, T. Y. Dou, J. N. Cui and L. Yang, J. Am. Chem. Soc., 2015, 137, 14488–14495 CrossRef CAS PubMed.
- M. H. Lee, J. H. Han, J. H. Lee, H. G. Choi, C. Kang and J. S. Kim, J. Am. Chem. Soc., 2012, 134, 17314–17319 CrossRef CAS PubMed.
- J. Yin, Y. Kwon, D. Kim, D. Lee, G. Kim, Y. Hu, J.-H. Ryu and J. Yoon, J. Am. Chem. Soc., 2014, 136, 5351–5358 CrossRef CAS PubMed.
- H. Chen, Y. Tang, M. Ren and W. Lin, Chem. Sci., 2016, 7, 1896–1903 RSC.
- J. Fan, Z. Han, Y. Kang and X. Peng, Sci. Rep., 2016, 6, 19562–19569 CrossRef CAS PubMed.
- M. Wei, P. Yin, Y. Shen, L. Zhang, J. Deng, S. Xue, H. Li, B. Guo, Y. Zhang and S. Yao, Chem. Commun., 2013, 49, 4640–4642 RSC.
- H. Xiao, P. Li, X. Hu, X. Shi, W. Zhang and B. Tang, Chem. Sci., 2016, 7, 6153–6159 RSC.
- H. Xiao, C. Wu, P. Li and Bo Tang, Anal. Chem., 2018, 90, 6081–6088 CrossRef CAS PubMed.
- T. K. Chung, M. A. Funk and D. H. Baker, J. Nutr., 1990, 120, 158–165 CrossRef CAS PubMed.
- R. Hong, G. Han, J. M. Fernández, B. Kim, N. S. Forbes and V. M. Rotello, J. Am. Chem. Soc., 2006, 128, 1078–1079 CrossRef CAS PubMed.
- J. B. Ubbinka, W. J. H. Vermaak, A. v. d. Merwe and P. J. Beckerb, Clin. Chim. Acta, 1992, 207, 119–128 CrossRef.
- P. D. Sadowitz, B. A. Hubbard, J. C. Dabrowiak, J. Goodisman, K. A. Tacka, K. M. Aktas, M. J. Cunningham, R. L. Dubowy and A.-K. Souid, Drug Metab. Dispos., 2002, 30, 183–190 CrossRef CAS.
- A. Bertolotti, Y. Zhang, L. M. Hendershot, H. P. Harding and D. Ron, Nat. Cell Biol., 2000, 2, 326–332 CrossRef CAS PubMed.
Footnotes |
† Electronic supplementary information (ESI) available. See DOI: 10.1039/c8an01626g |
‡ These authors contributed equally to this work. |
|
This journal is © The Royal Society of Chemistry 2019 |