Photoluminescence enhancement of carbon dots induced by hybrids of photonic crystals and gold–silver alloy nanoparticles†
Received
22nd October 2017
, Accepted 29th November 2017
First published on 11th December 2017
Abstract
Carbon dots are attracting worldwide interest owing to their unique optical properties and great potential for application. However, orange/red emission carbon dots are still rare and exhibit relatively low efficiency. In this work, we present a novel strategy to enhance the fluorescence intensity of orange carbon dots, by synergistically manipulating an electromagnetic field through opal photonic crystals and the localized surface plasmon resonance of a metal structure. An optimum intensity enhancement of 25-fold was obtained for a modulated sample of PMMA opal photonic crystals and 53-fold for PMMA opal photonic crystals/Au–Ag alloy plasmon hybrids. The local electromagnetic field distribution for the PMMA opal photonic crystals/Au–Ag hybrid is calculated by the finite difference time domain method. Furthermore, a fingerprint identification system based on synergistic modulation is realized in the composite system, which provides novel insights into the potential applications of carbon dot films.
Introduction
The study of carbon dots (CDs) is attracting much research interest because of their characteristic physical and chemical properties, including tunable and stabilized photoluminescence, high optical absorptivity, favorable biocompatibility, low toxicity, low cost, and readily available reagents.1–3 These characteristics of CDs offer the potential for application in biomedical imaging, analytical detection, and light-emitting devices.2,4,5 In the past decade, rapid development has been achieved in the field of luminescent CDs. For instance, high-efficiency liquid CDs have been prepared, especially blue CDs with a quantum efficiency (QY) of more than 80%.6 In addition, liquid green, yellow, and orange emissions have also been reported, with QY values of more than 50%.7 Despite this progress, solid orange carbon dots (OCDs) still show low efficiency, which limits their application, especially in devices such as white LEDs. Therefore, much effort has been devoted to improving the luminescence intensity and QY of CDs.8,9 Of the various methods, local electromagnetic field modulation is a powerful technique to enhance the photoluminescence of emitters in the vicinity, including exploring metal nanostructures, photonic crystals, and so on.10,11 Metal nanostructures have been widely used to improve the fluorescence intensity of dyes,12,13 quantum dots,14 rare earth-doped nanoparticles15,16 and carbon dots,9 using the metal-enhanced fluorescence effect. Metal surfaces, particularly gold and silver, constitute substrates for surface plasmon resonance enhancement, a recognized fluorescence enhancement technique.17–20 Plasmonics is the study of light–matter interactions on nano-sized metal structures. The main characteristics of plasmons are determined by the metallic nanostructure, which leads to strong coupling between the incident photons and the collective oscillations of free electrons on the metal surface. The plasmon effects focus and guide light on a nanometer scale. It is well known that plasmon nanostructures can concentrate incident light into a strong localized electric field distributed in the subwavelength region near the surface of the nanostructures.21 The field enhancement process is a near-field effect that almost decays exponentially away from the metal surface.15
The use of photonic crystals, which are optical nanostructures that exhibit periodic spatial variation, is another effective method to adjust the local electromagnetic field.22,23 It can be expected that the luminescence intensity of CDs can be improved more effectively by a combination of the surface plasmon and photonic crystal effects.24,25 In this work, we propose a hybrid structure of gold–silver alloy nanoparticles (Au–Ag NPs) and PMMA opal photonic crystals (OPCs), which can effectively combine the photonic crystal effect of three-dimensional PMMA opal and the surface plasmon effect of the Au–Ag NPs to improve the fluorescence intensity of the CD film. Upon modulation of the Au–Ag NPs/PMMA OPC hybrids, the emission intensity of the OCDs was improved by a factor of more than 53, and the external quantum efficiency of the films was increased from 7.9% (glass substrate) to 13.3% (composite substrate). This device was also applied to high-resolution fluorescent fingerprint identification.15,25,26
Experimental
Synthesis of OCDs
1 mmol p-phenylenediamine (PPD, 99.9%) and 10 mL methylbenzene were stirred at room temperature for 1 h, and then the solution was transferred into a 30 mL Teflon-lined stainless-steel autoclave. The sealed autoclave was heated at 180 °C in an oven for 12 h and naturally cooled to room temperature. The samples were purified by centrifugation of toluene and ethanol. The final products were dried and collected under vacuum at 50 °C and dissolved in ethanol, and could remain stable for several months.
Synthesis of Au–Ag NPs
Part 1.
Ag nanospheres: first, a mixture of 25 mL of ethylene glycol (EG) and 3.3 g of polyvinylpyrrolidone (PVP) was kept stirred for 2 h and then heated to 120 °C. Next, 0.25 g of AgNO3 was added to the resulting solution under N2 protection, and the solution was stirred for 1 h. Finally, Ag nanospheres were obtained by washing with ethanol and acetone. The size of the Ag nanospheres was controlled by the amount of AgNO3 added.
Part 2.
Au–Ag nanoalloys: first, 9 mL of deionized water, 15 mg of PVP and 1 mL of the synthesized Ag nanosphere solution synthesized in part 1 were mixed and maintained at 100 °C for 2 min under reflux conditions. Then, 2.5 mL of chloroauric acid (0.25 mmol L−1) was added to the above-mentioned solution at a rate of 30 mL h−1. After 20 min, NaCl was added to the resulting solution. Finally, the excess PVP and NaCl were removed by washing with water. The size of the nanoalloys was controlled by the size of the Ag nanospheres used.
Preparation of the OCDs/Au–Ag NPs/OPC composite film
The OPC films were prepared by a self-assembly method, and the photonic stop bands of the photonic crystals were controlled by adjusting the PMMA microspheres. On the prepared opal film, a Au–Ag alloy film was prepared by a suspension coating method, and it was dried at 30 °C for 2 h. Next, a 0.002 g mL−1 PVP-K30 ethanol solution and a certain concentration of OCDs–PVP-K30 ethanol solution were added to the surface of the drying film, and the CD composite film was prepared by suspension coating and dried at 30 °C for 2 h.
Results and discussion
Fabrication and characterization of the OCDs/Au–Ag NPs/OPCs composite film
The preparation process for the stromatolithic nanostructured OCDs/Au–Ag NPs/OPCs is shown in Scheme 1(a). Here, the fluorescence of the OCDs was measured by the reflection method, and the optical circuit is shown in Scheme 1(b), and the light path remains the same during the whole experiment.
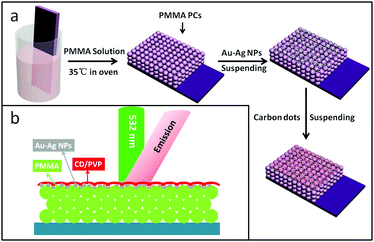 |
| Scheme 1 (a) Schematic illustration of the fabrication process for the OCDs/Au–Ag NPs/OPC composite thin films. (b) Schematic diagram of the film and incident direction of excitation. | |
Characterization of the OCD-liquid
A transmission electron microscopy (TEM) image of the as-prepared OCDs is shown in Fig. 1(a), which was recorded after the dissolution of the OCDs in ethanol and extraction of the nanoparticles. The TEM image reveals that relatively uniform OCD nanoparticles are formed, exhibiting average diameters of approximately 2.2 nm. In addition, the high-resolution TEM (HR-TEM) image in the inset of Fig. 1(a) reveals a crystalline organization of OCDs, with a lattice spacing of 0.25 nm corresponding to the (100) planes of the graphite core. This indicates that the black particles inside are carbonized OCDs. The Fourier-transform infrared spectra of the OCDs (Fig. 1(b)) indicate an abundance of chemical groups on their surfaces. The following bonds were observed: stretching vibrations of O–H at 3428 cm−1, N–H at 3338 cm−1, C–H at 2923 cm−1, and C
O at 1643 cm−1, bending vibrations of C–H at 1358 cm−1, and asymmetric stretching vibrations of C–NH–C at 1120 cm−1, and epoxide at 1011 cm−1.6,27,28 With these hydrophilic oxygen groups, OCDs can be easily dispersed in aqueous solution and also have potential in bio-labeling applications.
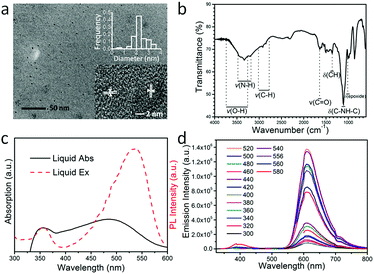 |
| Fig. 1 (a) TEM images of the OCDs. Inset: The size distribution of the OCDs and high-resolution TEM image of the OCDs. (b) The FT-IR spectra of the OCDs. (c) Normalized absorption and FL excitation spectra of the OCDs (the monitored emission wavelength is at 608 nm). (d) The luminescence emission spectra (with progressively longer excitation wavelengths from 300 nm in 20 nm increments) of the OCDs in ethanol solution. | |
In the ultraviolet-visible absorption spectra, two obvious peaks were observed at 354 nm and 489 nm in an aqueous solution of OCDs, which have high quality and high conjugation. According to previous reports, the two absorption states originate from the defect states transition and surface molecular states transition.29,30 In the excitation spectra, OCDs have optimal excitation wavelength at 354 nm and 532 nm while monitoring the red emission at 608 nm (Fig. 1(c)). The deviation of the excitation band from the absorption band may be caused by nonradiative relaxation of CDs at higher energy states. Excitation-independent photoluminescence was observed, which shows a fixed emission peak at 608 nm under excitation with various wavelengths (Fig. 1(d)). This behavior can be attributed to the surface state transition.30,31 The maximum emission intensity was obtained when the OCDs were pumped at 532 nm, with a Stokes shift of 76 nm. This is consistent with the excitation spectra. The relationship between the excitation wavelength and emission intensity indicates that 532 nm is the ideal excitation wavelength (Fig. S1, ESI†). Therefore, the fluorescence enhancement of OCDs was investigated upon excitation at 532 nm. Additionally, these excitation-independent OCDs would be more beneficial to study the luminescent enhancement by changing the location of the photon stop band and/or the plasmon resonance. The concentration of OCDs in PVP ethanol solution was fixed at 0.2 mg mL−1 (Fig. S2, ESI†).
Fluorescent enhancement of OCDs/OPC composite films
It is worth mentioning that the thickness of the samples directly affects the luminous intensity of the material.15 Therefore, we must control the relative thickness of the OCD film on the reference and composite substrates. Table 1 lists the thicknesses of the OCDs on various photonic crystals relative to that on the glass substrate, deduced according to the Beer–Lambert law. The absorbance is linearly related to the thickness of the film (dn), and thus the relative thickness of OCDs in different samples can be obtained by the measurement of Tn. It can be observed that the OCD thin films on the different substrates have the same thickness (d(OPCs)/d(Glass) ≈ 1). The absolute thickness of CDs on the glass surface is 15 nm (Fig. S3(a1), ESI†).
Table 1 Relative thickness of the OCD layer on the glass substrate and on the OPC film and the ratio of d(OPCs)/d(Glass)
Stop band (nm) |
511 |
521 |
553 |
584 |
647 |
690 |
807 |
d(OPCs)/d(Glass) |
0.998 |
1.001 |
1.00048 |
0.998 |
0.991 |
0.982 |
0.998 |
First, we tested the effect of different OPC stop bands on the enhancement effect of OCDs/OPCs. Fig. 2(a) shows the transmission spectra of OPCs with different stop band positions (upper) and the typical emission spectrum of the OCDs/OPC samples (lower). The transmittance spectra of various OPCs indicate that the photonic stop band of the OPCs can be tuned in the range of 500–810 nm, which is allowed to overlap with the excitation and emission lines of the OCDs. It is worth noting that the photonic stop band of the OPCs was tuned by controlling the diameter of the PMMA spheres (Fig. S4, ESI†). The fluorescence enhancement factor of the OCDs versus the photonic stop band of the OPCs is shown in Fig. 2(b). The largest enhancement factor was obtained when the photonic stop band was tuned to the excitation wavelength (532 nm) and had little overlap with the emission wavelength. The enhancement factor decreased when the stop band and emission spectrum showed significant overlap. When the stop band was far away from the excitation and emission wavelength of the OCDs, the enhancement factor would randomly increase. The reason for this result was two-fold. First, the OPCs played a regulatory role in the optical field. Second, the nanometer OCD film was attached to the PMMA spheres, and some OCDs would appear inside the photonic crystal, which was detrimental to the enhancement. This is in agreement with the conclusion in our previous work, in which we studied the coupling of PMMA OPCs with upconversion nanoparticles (UCNPs) and observed that the enhancement factor depended strongly on the OPC photonic stop band.32 In addition, we characterized the effects of the thickness of the OCDs on the enhancement factor, in which we changed the thickness of the fluorescent film by varying the amount of PVP in the ethanol solution (Fig. S3(b), ESI†). It can be concluded that the enhancement factor decreased exponentially with increased OCD film thickness, which is attributed to the effective interaction distance between the fluorescent materials and the substrates.11 The stop band effect was more obvious with the increased stop band depth (Fig. S5, ESI†) and the OPC thickness effect was not distinct (Fig. S6, ESI†).33 The external quantum efficiency of the OCD films was measured to be 11.3% on the OPC substrates.
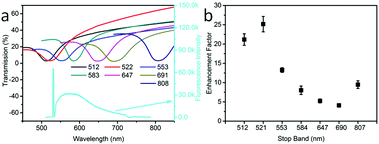 |
| Fig. 2 (a) The transmission spectra of OPCs with different particle sizes (upper) and the typical emission spectrum (lower) of the OCDs/OPCs under 532 nm excitation. (b) The enhancement factor versus the stop band for OPCs which have different sizes. | |
Next, we prepared the Ag NPs that were used as seeds for the growth of the Au–Ag alloy. The composition of the alloy was controlled by the relative amounts of added Au and Ag salts.34,35 The Au–Ag products were monodispersed ellipsoidal alloy NPs with approximate dimensions of 27 nm (semi-major axis) and 18 nm (semi-minor axis) (see Fig. S7, ESI†) and the nanoshells were obtained by increasing the Au content.36 The atomic content of Au and Ag was measured by EDAX (see Fig. S8, ESI†). The extinction spectra of Au–Ag NP aqueous solutions with various concentrations of Au and Ag are presented in Fig. 3(a). The extinction peaks show a red shift with the increased concentration ratio of Au to Ag and the position of the excitation peak can be tuned in the range of 410–800 nm.37 Then, we divided the extinction value of OCDs at 532 nm (excitation) by the value at 577 nm (emission), as shown by the red line in Fig. 3(b). As a result, the extinction ratio first increased and then decreased with the increase in the concentration ratio of Au to Ag. According to the results of OCD films on the Au–Ag NPs/PMMA OPC substrates, there was also a trend of the enhancement factor first increasing and then decreasing with the increasing concentration ratio of Au to Ag, as shown by the black line in Fig. 3(b). The introduction of the shell structure was more conducive to the amplification of the electromagnetic field.36,38 Then, as the Au content increases, the thickness of the shell decreases, and the advantage of the nanoshell diminishes. The maximum enhancement factor was 53, with an external quantum efficiency of 13.3%. In addition, the number of Au–Ag NPs on the surface of the OPCs would also affect the value of the enhancement factor. When the coverage of the alloy nanoparticles reaches a certain extent, the surface plasmon resonance can be significantly enhanced, and the OPCs also exhibit long-range control over light (see the experimental result in Fig. S9, ESI† and the simulated local electromagnetic field distribution in Fig. 4). In addition, the enhancement factor of the Au–Ag NPs/OCD films and OCDs is 8.95 (see Fig. S10, ESI†). The fluorescence spectra taken from 50 randomly selected points were very similar (see Fig. S11, ESI†).
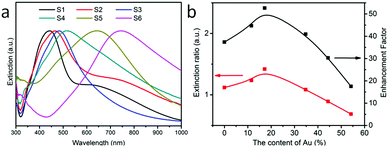 |
| Fig. 3 (a) The extinction spectra of Au–Ag NPs with varied Au content in the Au–Ag NPs. S1-0, S2-11.55%, S3-17.23%, S4-34.76%, S5-44.38%, S6-54.20%. (b) Ratio of extinction at 577 nm to that at 532 nm, and the enhancement factor versus the content of Au in Au–Ag NPs. | |
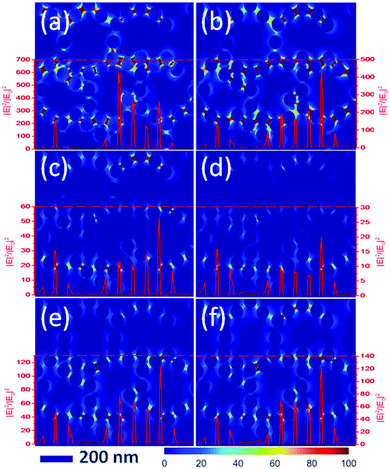 |
| Fig. 4 Simulated local electromagnetic field distribution of AgNPs/PMMA OPCs versus different wavelengths of incident light. (a) 450 nm; (b) 532 nm; (c) 577 nm; (d) 608 nm; (e) 650 nm; (f) 700 nm. | |
In order to reveal the fluorescence enhancement mechanism, the fluorescent dynamics in different samples were investigated under 485 nm excitation (see Table 2 and Fig. S12, ESI†). The lifetime of OCDs in ethanol solution is higher than that of the OCD films on the other substrates. The difference in the lifetimes can be attributed to different media surrounding the nanoparticles. Based on the Lorentz oscillator model, the radiative lifetime can be written as
| 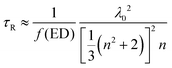 | (1) |
where
f(ED) is the oscillator strength for the electric dipole transition,
λ0 is the wavelength of incident light, and
n is the refractive index.
39 This shows that the radiative lifetime increases with a decrease in the material refractive index. The refractive index can be expressed as
| n(x)eff = xnOCD + (1 − x)nmed | (2) |
where
n(
x)
eff =
n is the effective refractive index of OCDs (
nOCD) surrounded by the medium (
nmed), and
x is the filling factor. In addition, the refractive index values of the media are 1.36 (ethanol) and 1.50 (PVP film).
40 This indicates that the effective refractive index of the OCD ethanol solution is higher than the effective refractive index of the OCDs/PVP film, and the radiative lifetime of the OCDs in ethanol solution is longer than that of the OCDs/PVP film.
Table 2 A list of decay lifetime constants of OCDs in different substrates
|
λ
Ex (nm) |
λ
Em (nm) |
A
1
|
T
1 (ns) |
A
2
|
T
2 (ns) |
τ (ns) |
χ
2
|
Ethanol |
485 |
608 |
0.01 |
1.91 |
0.02 |
9.45 |
8.76 |
1.05 |
Glass |
577 |
0.06 |
1.23 |
0.02 |
7.85 |
5.73 |
1.12 |
OPCs |
0.15 |
4.39 |
0.01 |
6.58 |
4.59 |
1.05 |
Au–Ag NPs/OPCs |
0.16 |
4.07 |
0.01 |
6.24 |
4.26 |
1.03 |
Moreover, the fluorescence intensity of the OCDs–PVP films is much greater in the Au–Ag NPs/OPC composite substrate, which has a shorter lifetime and higher transition rate.41 The quantum efficiency Q is given by
|  | (3) |
where
ΓR is the radiative decay rate and
ΓNR is the non-radiative decay rate. And the fluorescence lifetime is expressed as
| 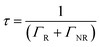 | (4) |
According to the above test results regarding quantum efficiency and lifetime, the radiative transition rates of OCDs/Au–Ag NPs/PMMA OPCs are higher than that of OCDs. The effect of a background of opal photonic crystals and surface plasmon resonance would modify the radiative decay rate of the OCDs.
19,41 According to the previous conclusion, the reduced lifetime is caused by the changes in the radiative and nonradiative dacay rates.
42,43 It is shown that the optical field amplification of the composite film is due to not only the enhancement of the excitation field, but also the enhancement of the emission field.
In order to understand the electromagnetic field distribution (|E|2/|E0|2) of plasma resonance and photonic crystal effects, we performed a theoretical calculation by finite difference time domain (FDTD) (see Fig. 4). The substrate of this FDTD model was a composition of Ag NPs and PMMA OPCs with a stop band at 521 nm, and the incident light was a plane wave. These images showed the amplifying effect of Ag NPs/OPCs by different wavelengths of incident light. It is important to note that we used an incident light wavelength range of 2 nm in Fig. 4. In addition, we simulated the amplification effect of different wavelength ranges of incident light according to the excitation light and emission light in the experiment (see Fig. S13, ESI†). The results indicated that the amplification effect did not change with the wavelength range of incident light. In Fig. 4, the red line shows the amplification of the light field intensity. From the images in Fig. 4, it can be seen that the highest amplification was obtained for 450 nm (the resonance absorption of Ag NPs) and 532 nm (the resonance absorption of PMMA OPCs). When the incident light was coupled with the resonance absorption of AgNPs, the maximum magnification factor was greater than 600 fold. Moreover, the amplification effect was relatively weak with the increased incident wavelength. There was still light field amplification at the position of the fluorescence emission wavelength, which was consistent with previous results. However, the optical field amplification increased with further increase in the incident light wavelength, which is due to the changes in the scattering effect.15 Finally, the calculation result of the density of metal nanoparticles was presented in Fig. S14 (ESI†), and it indicated that the best optical field amplification structure was the composition of plasma resonance and photonic crystal effects.
Technically, the synergy of the plasmon resonance effect and photonic crystal effect of the OCD device shows promise to be utilized in various applications. Here, we further investigated the high-resolution fingerprint identification (see Fig. 5). Fig. 5(b1 and c1) shows the fingerprints obtained by different samples. It can be observed that the fingertip pressed directly on the OCDs/glass substrate was indistinct, dark and without fluorescence for fingers excited by 365 nm laser light. However, using the surface plasmon resonance and photonic crystal effect OCDs, the fingerprint was bright and could easily be recognized. When the fingerprint was imaged using confocal microscopy, a high-resolution image was obtained. At higher magnifications (Fig. 5(c2 and c3)), certain details such as circles and bifurcations are clearly visible. The images clearly showed the typical fingerprint ridge pattern in sufficient detail that it would enable the identification of an individual. Fig. 5(e) shows the emission spectrum of a fingerprint on the surface of Au–Ag NPs/OPCs. The high-magnification image indicates that the synergy of the plasmon resonance effect and photonic crystal effect OCDs is powerful in high-resolution imaging.
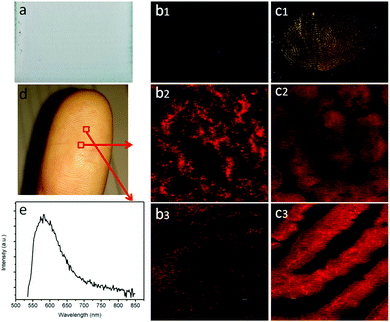 |
| Fig. 5 (a) The optical image of a complex film on the glass. (b1 and c1) The digital images of a fingerprint on the surface of the glass and the complex film. (b2, b3, c2 and c3) Confocal fluorescence images of a fingerprint on the surface of the glass and the complex film. (d) A digital image of the finger. (e) PL intensity of the fingerprint. | |
Conclusions
In summary, we present a novel strategy to enhance the fluorescent intensity of OCDs, through a synergistic modulation involving the far-field photonic crystal effect and near-field localized surface plasmon resonance. Both the photonic stop band of PMMA OPCs and the position of the plasma shock were tuned to 532 nm. An optimum enhancement factor of 53 in the Au–Ag alloy plasmon/PMMA OPCs was obtained for the intensity of the OCDs under 532 nm excitation. Additionally, the same results could be obtained through a FDTD theoretical calculation. Furthermore, fingerprint identification based on synergistic modulation is realized in the composite system, which provides novel insights into the potential applications of CD films.
Conflicts of interest
There are no conflicts to declare.
Acknowledgements
This work was supported by the National Key Research and Development Program (2016YFC0207101), the Major State Basic Research Development Program of China (973 Program) (No. 2014CB643506, 2014CB921302), the National Natural Science Foundation of China (Grant No. 61775080, 11374127, 11674126, 11674127, 11504131, 61674067), the Jilin Province Natural Science Foundation of China (No. 20150520090JH, 20170101170JC) and Jilin Province Science Fund for Excellent Young Scholars (No. 20170520129JH, 20170520111JH).
Notes and references
- S. Hamd-Ghadareh, A. Salimi, F. Fathi and S. Bahrami, Biosens. Bioelectron., 2017, 96, 308–316 CrossRef CAS PubMed.
- K. Jiang, S. Sun, L. Zhang, Y. Lu, A. Wu, C. Cai and H. Lin, Angew. Chem., 2015, 54, 5360–5363 CrossRef CAS PubMed.
- X. L. Shao, H. Gu, Z. Wang, X. L. Chai, Y. Tian and G. Y. Shi, Anal. Chem., 2013, 85, 418–425 CrossRef CAS PubMed.
- J. Jin, S. J. Zhu, Y. B. Song, H. Y. Zhao, Z. Zhang, Y. Guo, J. B. Li, W. Song, B. Yang and B. Zhao, ACS Appl. Mater. Interfaces, 2016, 8, 27956–27965 CAS.
- X. Y. Zhang, Y. Zhang, Y. Wang, S. Kalytchuk, S. V. Kershaw, Y. H. Wang, P. Wang, T. Q. Zhang, Y. Zhao, H. Z. Zhang, T. Cui, Y. D. Wang, J. Zhao, W. W. Yu and A. L. Rogach, ACS Nano, 2013, 7, 11234–11241 CrossRef CAS PubMed.
- S. Zhu, Q. Meng, L. Wang, J. Zhang, Y. Song, H. Jin, K. Zhang, H. Sun, H. Wang and B. Yang, Angew. Chem., 2013, 52, 3953–3957 CrossRef CAS PubMed.
- F. L. Yuan, Z. B. Wang, X. H. Li, Y. C. Li, Z. A. Tan, L. Z. Fan and S. H. Yang, Adv. Mater., 2017, 29, 6 Search PubMed.
- S. Lin, Z. Wang, Y. Zhang, Y. Huang, R. Yuan, W. Xiang and Y. Zhou, J. Alloys Compd., 2017, 700, 75–82 CrossRef CAS.
- Y. Liu, C. Y. Liu, Z. Y. Zhang, W. D. Yang and S. D. Nie, J. Mater. Chem. C, 2015, 3, 2881–2885 RSC.
- Z. Yin, H. Li, W. Xu, S. B. Cui, D. L. Zhou, X. Chen, Y. S. Zhu, G. S. Qin and H. W. Song, Adv. Mater., 2016, 28, 2518–2525 CrossRef CAS PubMed.
- H. Wang, Z. Yin, W. Xu, D. L. Zhou, S. B. Cui, X. Chen, H. N. Cui and H. W. Song, Nanoscale, 2016, 8, 10004–10009 RSC.
- A. M. Michaels, M. Nirmal and L. E. Brus, J. Am. Chem. Soc., 1999, 121, 9932–9939 CrossRef CAS.
- K. Kim, J. W. Lee and K. S. Shin, ACS Appl. Mater. Interfaces, 2012, 4, 5498–5504 CAS.
- K. M. Abualnaja, L. Siller and B. R. Horrocks, Nanotechnology, 2015, 26, 12 CrossRef PubMed.
- X. Chen, W. Xu, L. H. Zhang, X. Bai, S. B. Cui, D. L. Zhou, Z. Yin, H. W. Song and D. H. Kim, Adv. Funct. Mater., 2015, 25, 5462–5471 CrossRef CAS.
- J. J. He, W. Zheng, F. L. Ligmajer, C. F. Chan, Z. Y. Bao, K. L. Wong, X. Y. Chen, J. H. Hao, J. Y. Dai, S. F. Yu and D. Y. Lei, Light: Sci. Appl., 2017, 6, 11 Search PubMed.
- H. Y. Jang, S. K. Kim and S. Park, J. Phys. Chem. C, 2015, 119, 10585–10591 CAS.
- K. Park, K. Jung, S. J. Kwon, H. S. Jang, D. Byun, I. K. Han and H. Ko, Adv. Funct. Mater., 2016, 26, 7836–7846 CrossRef CAS.
- A. Hatef, S. M. Sadeghi and M. R. Singh, Nanotechnology, 2012, 23, 9 Search PubMed.
- D. Schindel and M. R. Singh, J. Phys.: Condens. Matter, 2015, 27, 8 CrossRef PubMed.
- X. C. Ma, Y. Dai, L. Yu and B. B. Huang, Light: Sci. Appl., 2016, 5, 13 Search PubMed.
- M. R. Singh, C. Racknor and D. Schindel, Appl. Phys. Lett., 2012, 101, 4 Search PubMed.
- J. D. Cox, M. R. Singh, G. Gumbs, M. A. Anton and F. Carreno, Phys. Rev. B: Condens. Matter Mater. Phys., 2012, 86, 10 CrossRef.
- P. Alivisatos, Nat. Biotechnol., 2004, 22, 47–52 CrossRef CAS PubMed.
- H. B. Chen, K. W. Chang, X. J. Men, K. Sun, X. F. Fang, C. Ma, Y. X. Zhao, S. Y. Yin, W. P. Qin and C. F. Wu, ACS Appl. Mater. Interfaces, 2015, 7, 14477–14484 CAS.
- R. D. Roorda, A. C. Ribes, S. Damaskinos, A. E. Dixon and E. R. Menzel, J. Forensic Sci., 2000, 45, 563–567 CAS.
- M. Algarra, B. B. Campos, K. Radotic, D. Mutavdzic, T. Bandosz, J. Jimenez-Jimenez, E. Rodriguez-Castellon and J. da Silva, J. Mater. Chem. A, 2014, 2, 8342–8351 CAS.
- L. Zhang, W. L. Hou, Q. J. Lu, M. L. Liu, C. Chen, Y. Y. Zhang and S. Z. Yao, Anal. Chim. Acta, 2016, 947, 23–31 CrossRef CAS PubMed.
- L. Wang, S.-J. Zhu, H.-Y. Wang, S.-N. Qu, Y.-L. Zhang, J.-H. Zhang, Q.-D. Chen, H.-L. Xu, W. Han, B. Yang and H.-B. Sun, ACS Nano, 2014, 8, 2541–2547 CrossRef CAS PubMed.
- T. X. Zhang, J. Y. Zhu, Y. Zhai, H. Wang, X. Bai, B. Dong, H. Y. Wang and H. W. Song, Nanoscale, 2017, 9, 13042–13051 RSC.
- K. Dohnalova, A. N. Poddubny, A. A. Prokofiev, W. de Boer, C. P. Umesh, J. M. J. Paulusse, H. Zuilhof and T. Gregorkiewicz, Light: Sci. Appl., 2013, 2, 6 Search PubMed.
- Z. Yin, Y. S. Zhu, W. Xu, J. Wang, S. Xu, B. Dong, L. Xu, S. Zhang and H. W. Song, Chem. Commun., 2013, 49, 3781–3783 RSC.
- S. Xu, W. Xu, Y. F. Wang, S. Zhang, Y. S. Zhu, L. Tao, L. Xia, P. W. Zhou and H. W. Song, Nanoscale, 2014, 6, 5859–5870 RSC.
- S. Link, Z. L. Wang and M. A. El-Sayed, J. Phys. Chem. B, 1999, 103, 3529–3533 CrossRef CAS.
- S. Patskovsky, E. Bergeron, D. Rioux, M. Simard and M. Meunier, Analyst, 2014, 139, 5247–5253 RSC.
- L. Au, Y. C. Chen, F. Zhou, P. H. C. Camargo, B. Lim, Z. Y. Li, D. S. Ginger and Y. N. Xia, Nano Res., 2008, 1, 441–449 CrossRef CAS PubMed.
- Y. Cheng, C. Chen, X. Chen, J. J. Jin, H. Li, H. W. Song and Q. L. Dai, J. Mater. Chem. A, 2017, 5, 6515–6521 CAS.
- X. Chen, D. L. Zhou, W. Xu, J. Y. Zhu, G. C. Pan, Z. Yin, H. Wang, Y. S. Zhu, S. B. Cui and H. W. Song, Sci. Rep., 2017, 7, 8 CrossRef PubMed.
- R. S. Meltzer, S. P. Feofilov, B. Tissue and H. B. Yuan, Phys. Rev. B: Condens. Matter Mater. Phys., 1999, 60, 14012–14015 CrossRef.
- Q. Y. Zhang, J. Shen, J. Wang, G. M. Wu and L. Y. Chen, Int. J. Inorg. Mater., 2000, 2, 319–323 CrossRef CAS.
- C. Racknor, M. R. Singh, Y. N. Zhang, D. J. S. Birch and Y. Chen, Methods Appl. Fluoresc., 2014, 2, 6 Search PubMed.
- S. Dey and J. Zhao, J. Phys. Chem. Lett., 2016, 7, 2921–2929 CrossRef CAS PubMed.
- S. Dey, Y. D. Zhou, X. D. Tian, J. A. Jenkins, O. Chen, S. L. Zou and J. Zhao, Nanoscale, 2015, 7, 6851–6858 RSC.
Footnote |
† Electronic supplementary information (ESI) available: Luminescence emission spectra, AFM images and the decay time of OCD films, TEM, absorption of OPC films, EDAX and SEM images of Au–Ag NPs and FDTD theoretical calculations. See DOI: 10.1039/c7tc04824f |
|
This journal is © The Royal Society of Chemistry 2018 |
Click here to see how this site uses Cookies. View our privacy policy here.