One-step mild biorefinery of functional biomolecules from microalgae extracts
Received
4th August 2017
, Accepted 17th November 2017
First published on 17th November 2017
Abstract
Fractionation of complex matrices such as biomass into diverse functional biomolecules without disrupting the biomolecule functionalities is a real challenge. Known separation processes are designed for the recovery of single products such as hydrophilic proteins or hydrophobic pigments, discarding the other products as waste. Here we present a concept for one-step biorefinery of hydrophobic and hydrophilic biomolecules from complex matrices using an ionic liquid-based surfactant free emulsion stabilised by microgel particles, while maintaining the product integrity. We demonstrate the process by simultaneous extraction of pigments and proteins from a microalgae extract; the hydrophobic pigments are transferred to the ionic liquid droplets and the proteins to the aqueous continuous phase. In contrast to most solvent extraction procedures, with this concept, the proteins retain their nativity, opening up an extraction pathway to fully functioning proteins while harvesting hydrophobes. A perspective towards continuous operation of this novel process is presented.
Introduction
Two-phase systems have long been exploited for the separation and purification of biomolecules in chemical and biotechnological industries. Aqueous two-phase systems were primarily used for the isolation and separation of water-soluble molecules such as proteins,1 whereas water–organic solvent-based two-phase systems were used for the extraction of hydrophobic molecules such as pigments and lipids.2,3 Generally, existing separation methods are focused on the recovery of single components from a range of feedstocks such as corn, yeast, fungi, CHO cells (Straathof et al., 2011)3 and also from microalgae (Orr et al., 2017, Roux et al., 2017, Cuellar-Bermudez et al., 2014).3 However, for a truly biobased economy, complete utilization of biomass is needed, which is, depending on the type of biomass (e.g. microalgae), a valuable source of phenols, alkaloids, proteins, pigments and lipids. Therefore, it is necessary to separate the components within a biorefinery concept into hydrophilic and hydrophobic components before further fractionation.4 Classically, emulsions form by mixing two incompatible liquids with a surfactant that kinetically or thermodynamically stabilises the dispersed state.5 Emulsion-based separation systems could provide a solution for the simultaneous extraction of hydrophilic and hydrophobic biomolecules from biomass.5 They have been used for both food and cosmetics as well as for the separation of biomolecules, again in most cases focusing on the isolation of one single product. Unfortunately, both organic solvents6,7 and detergents8 were found to negatively affect the structure and functionality of water-soluble biomolecules like proteins and enzymes.6–8 Reversed micelles, which are water-in-organic solvent microemulsions stabilized by detergents, have been used to extract proteins and enzymes.9 The disadvantages are the complexity of the system (detergent/organic solvent/water) and poor back extraction of biomolecules from the hydrophilic core,10 damaging the products. Therefore, it is of high importance to find alternative systems for the simultaneous extraction of hydrophobic components (e.g. lipids, pigments) and hydrophilic (fragile) proteins from biomass without influencing their functionality and retaining their high value.
Ionic liquids (ILs), which are salts that are liquid at temperatures below 100 °C, have a negligible vapour pressure and therefore are seen as ‘green’ solvents in the separation of different biomass streams.11–14 The cost of ILs is still too high (Klein-Marcuschamer et al., 2011),14 but further development of low cost ILs, as is the case for lignocellulosic biomass pretreatment, is in progress and these should be able to compete in the near future with low cost chemicals (George et al., 2015).14 There is a growing interest in IL-based emulsions and microemulsions.15 By selecting the chemical composition of the IL phase, such systems offer a unique possibility to control, e.g., the hydrophobic/hydrophilic balance of the dispersed phase16 and concomitant extraction efficiencies. These compounds are natural candidates for (mild) extraction17 and pre-treatment processes.18 Only few studies can be found on the use of IL-based microemulsions as extraction media.19,20 Chen et al.19 studied the extraction of herbal compounds using an IL-in-water microemulsion modified by an IL surfactant. Optimization of the various components and their concentrations improved the extraction yields up to twofold compared to volatile organic solvents. The system described is, however, only suitable for small chemical components and analytical purposes and not for fragile biomolecules (e.g. proteins) and large scale application. In another study,20 a dual ionic liquid-based microemulsion, wherein one IL is a surfactant and the other is a substitute for an organic solvent, was used for the selective extraction of haemoglobin from whole blood, yet again focused on single product recovery.
This manuscript describes the simultaneous separation and extraction of hydrophobic and hydrophilic components from biomass using an ionic liquid-in-water emulsion stabilised by poly(N-isopropylacrylamide (polyNIPAm) microgel particles. In previous studies,21,22 it was shown that these microgel particles spontaneously adsorb onto the IL–water interface and stabilise it resembling the robust Pickering stabilisation. Once at the IL/water interface, they allow for the transport of small molecules across the interface.21,22 Furthermore, these microgel particles exhibit lower critical solution temperature (LCST) behaviour. Above approximately 35 °C, they collapse and then the IL/water emulsion breaks.21,22 Hence, by a simple temperature switch one can easily recover both the IL phase and the microgel particles and prepare these for reuse.21,22 In this study, we did not employ this feature yet. The polyNIPAm particles are compatible with proteins in solutions and unlike small surfactants they are not likely to negatively influence the protein stability.
To demonstrate the potential of the extraction method, separation of components from microalgae using a biorefinery approach would be challenging; microalgae are known as a potential source of biofuel. For the studies in this manuscript, Haematococcus pluvialis (H. pluvialis) is used as an example (Lorentz et al., 2000).22H. pluvialis is a freshwater species of Chlorophyta from the family Haematococcaceae and contains valuable components such as proteins,23 pigments (astaxanthin24–27) and small amounts of lipids and carbohydrates. H. pluvialis is especially known for its high astaxanthin content, which is an important product for aquaculture and cosmetics.28 Therefore, H. pluvialis is a suitable microalgal species for the selective recovery of high value components by separating the hydrophobic (e.g. astaxanthin) and hydrophilic (e.g. proteins) compounds with this novel extraction technology while keeping the proteins intact. After separation, we show by native gel electrophoresis that the major hydrophilic component, i.e. a notoriously fragile protein, RuBisCO (ribulose-1,5-biphosphate carboxylase/oxygenase), retains its native conformation. Apparently, the finite concentration of the IL in the water phase was compatible with the native state of this protein. Also, the absence of surfactants in the system must have contributed to this positive result. As a first step towards application, we will discuss preliminary experiments of the separation process in continuous operation mode.
Experimental
Materials
The ionic liquid Cyphos 109 (trihexyl(tetradecyl)phosphonium bistriflamide, purity 98%) was ordered from Iolitec (Cyphos® is a trademark of Cytec, now a subsidiary of Solvay) and used without prior treatment. Poly-NIPAm microgel particles were synthesized and characterized as described previously.21 Spray dried H. pluvialis cells were provided by Feyecon B.V. (Weesp, Netherlands). N-Isopropylacrylamide (NIPAm), N,N-methylbisacrylamide (BIS), methacrylic acid (MA), potassium persulfate (KPS), styrene, sodium dodecyl sulphate (SDS) and phosphoric acid were purchased from Sigma Aldrich. Organic solvents such as methanol, tertiary methyl butyl ether and acetone were also purchased from Sigma Aldrich. Standard astaxanthin (∼97% purity of synthetic origin) was purchased from Ehrenstorfer GmBH. Milli-Q water was used for all studies. For protein analysis, a commercial assay kit (DC™ protein assay) was purchased from Bio-Rad and bovine serum albumin from Sigma–Aldrich (A7030) was used as the protein standard. For electrophoresis, Tris glycine 4–20% Criterion TGX precast gels were bought from Bio-Rad and the Pierce™ Silver Stain kit was purchased from Thermo Fisher Scientific.
Preparation and separation using a microgel stabilised emulsion
The microgel particles, synthesized in house as described elsewhere,21,22 were characterized by dynamic light scattering, revealing a hydrodynamic radius of 365 nm.21,22
For the batch experiments, the microgel-stabilized emulsion was prepared by diluting a stock solution of microgel particles (23 g l−1) to the desired concentration with Milli-Q water, combining it with an aqueous cell suspension (disrupted cells) and then adding the mixture to the ionic liquid solution. The emulsion is polydisperse and the droplets are ∼100–200 μm in size. The cell suspension loading (disrupted cells) of the aqueous phase was kept constant at 15 g l−1 for all studies. Two IL to aqueous phase ratios (1
:
9 and 3
:
7) and two concentrations of microgel particles in the aqueous phase (0.5 and 1 g l−1) were investigated. These ratios were selected as they are within the working window of the emulsion, i.e. 1
:
9 to 4
:
6. Outside this range, the emulsion is not stable. The samples were mixed by using a vortex tube shaker to facilitate separation of proteins and pigments in the aqueous and IL phases, respectively. The separation of hydrophilic and hydrophobic components from the cells was determined after different mixing times (1–180 minutes). The emulsion is separated from the rest by centrifugation at 5000 rpm for 5 minutes. The aqueous phase was analysed for protein content and the IL phase for pigment content.
For a continuous process, a set-up was made, as depicted in Fig. 1. The flow rates were set such that the ratio of the IL phase to the water phase in the mixing vessel is 3
:
7. The cell suspension was mixed with the aqueous microgel solution under magnetic stirring and was equilibrated for an initial 30 minutes followed by running in continuous mode until 100 minutes in the mixing vessel with the ionic liquid solution, whereas the aqueous phase was collected in the collection tank. Samples were taken from the mixing vessel at different time intervals (0–100 minutes), centrifuged to sediment the emulsion and finally analysed.
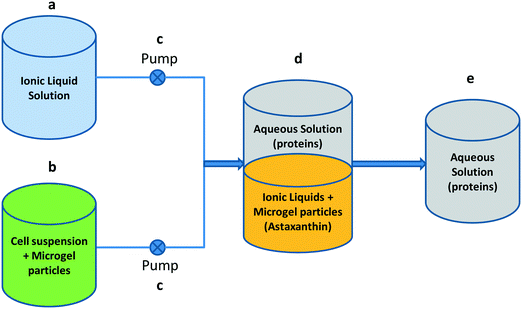 |
| Fig. 1 Schematic of continuous set up: a) ionic liquid – Cyphos 109 (200 ml solution); b) cell suspension + microgel particles (200 ml solution); c) peristaltic pump; d) mixing vessel (500 ml solution); e) collection tank (200 ml solution) with the collected aqueous phase. | |
The batch experiments were conducted in duplicate, and for the continuous studies, one sample was taken from each time point.21,22 The concentrated droplet phase might subsequently be broken by a temperature switch, the microgels separate from the IL phase and both can be recycled for reuse.
Protein analysis
After removal of the emulsion, the aqueous phase is analysed for total soluble protein content. The supernatant of disrupted H. pluvialis cells in water was analysed for total soluble protein as the control. The protein content was determined with the commercial assay kit DC™ protein assay (Bio-Rad, U.S.) using bovine serum albumin as the protein standard. The microplate assay protocol was used and the absorbance was measured at 750 nm using a microplate reader (Infinite M200, Switzerland). The protein yield is expressed as the percentage of the total protein in the cells.
Gel electrophoresis
To check whether the proteins keep their native structure in the IL-in-water emulsion gel, electrophoresis was performed. The aqueous phase was diluted 1
:
1 with native sample buffer. About 25 μl of the diluted sample was then applied on a 4–20% Criterion TGX, Tris glycine precast gel and run with 10× Tris glycine native buffer at 125 V for 75 min. The gel was stained for 30 minutes at room temperature (25 °C) under standard light conditions (not in the dark) using a Pierce™ Silver Stain Kit (User Guide: Pierce Silver Stain Kit UG (Number 24612)).
Pigment (astaxanthin) analysis
The pigment content in the IL phase was analysed by HPLC using a Thermo Scientific system coupled with Diode Array Detector-Dionex 3000. Chromeleon software version 7 was used for controlling the system and for data acquisition. The samples were analysed using a YMC carotenoid column (4.6 × 250 mm, 5 microns). A gradient program was run using a ternary mobile phase comprising methanol (A) : tertiary methyl butyl ether (B) : 1% v/v phosphoric acid (C) with the following details: 1) 0 minutes, 81% A : 15% B : 4% C; 2) 15 minutes, 66% A : 30% B : 4% C; 3) 23 minutes, 16% A : 80% B : 4% C; 4) 27 minutes, 16% A : 80% B : 4% C; 5) 27.1 minutes, 81% A : 15% B : 4% C; 6) 35 minutes, 81% A : 15% B : 4% C. The flow rate was set at 1 ml min−1 and the detection was performed at a λmax of 480 nm. The column temperature was set at 35 °C. A calibration curve in the concentration range of 1–11 μg ml−1 was obtained to determine the amount of astaxanthin in the samples. The standards were run in duplicate. The samples were diluted (10 times) using concentrated acetone (HPLC grade, purity ≥99.8%). The pigment yield is expressed as the percentage of the total pigment in the cells.
%pigment = 100% × ((pigment concentration (μg/ml) in extracted sample)/(pigment concentration (μg/ml) in disrupted biomass)) |
Results and discussion
Separation using surfactant-free emulsions
The potential of the microgel-stabilized IL emulsion to separate the hydrophilic and hydrophobic components from microalgae biomass was investigated. A cell suspension of broken H. pluvialis cells mixed with a solution of microgel particles was added to the IL (Cyphos 109; see Methods) and vortexed (approximately 5 seconds). Samples were taken after different mixing times (1–180 minutes) and analysed for protein and pigment content to determine the extraction efficiency. It was observed that the pigments are extracted in the discontinuous IL phase (Fig. 2) and subsequent analysis of the aqueous phase confirmed accumulation of proteins in the continuous aqueous phase. These results confirm the separation of hydrophilic and hydrophobic components in a single step.
 |
| Fig. 2
H. pluvialis cell suspension + microgel particles + IL. a) Before mixing. b) After mixing; aqueous phase and IL droplets containing proteins and astaxanthin, respectively; the inset shows the macroscopic picture of IL droplets in the emulsion. | |
Some of the key factors influencing the extraction efficiency of these components were investigated: the microgel particle concentration and the ratio of the IL to the aqueous phase. It was found that when the relative amount of IL is increased from 1
:
9 to 3
:
7 (the window of stability of the Cyphos 109-in-water emulsion), the amount of astaxanthin extracted after 180 minutes increases from 52% to 62% of the total astaxanthin present in the cells (Fig. 3). Increasing the microgel particle concentration from 0.5 g l−1 to 1 g l−1 leads to a higher extraction efficiency of the pigment (data not shown) for mixing times up to 120 minutes. This can be explained by an increase in the total interface between the water and IL phases, which speeds up the separation process.
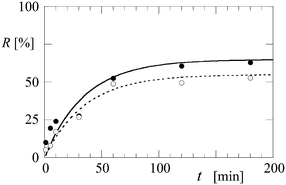 |
| Fig. 3 Recovery (R) of astaxanthin (in%) as a function of time t (min) for two IL : water ratios, 3 : 7 and 1 : 9, indicated by closed and open symbols, respectively (experimental error 10%). Microgel concentration: 0.5 g L−1. Curves are drawn to guide the eye. | |
For all tested microgel particle concentrations (0.5 g L−1 microgels is depicted in Fig. 4) and IL-aqueous ratios studied, the soluble proteins remain in the aqueous phase which as benefit that it contains only water soluble buffer components (e.g. sodium phosphate, potassium nitrate).
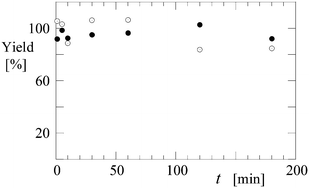 |
| Fig. 4 Recovery of proteins (in%) as a function of time t (min) for IL : water extraction ratios of 3 : 7 and 1 : 9 indicated by closed and open symbols, respectively (experimental error 10%). The microgel particle concentration is 0.5 g L−1. | |
Additionally, the nativity of the proteins after the separation process was investigated using native gel electrophoresis. As shown in Fig. 5, the band for the fragile biomarker protein RuBisCO, which is the main protein in the microalgae with a molecular mass of approx. 540 kDa, is intact and the protein is not broken into its subunits (RuBisCO (ref. 27) consists of 8 non-covalently linked large subunits of 55 kDa each and 8 small subunits of 13 kDa each), indicating that the proteins are not denatured in the process of separation. It is known that ionic liquids are able to denature RuBisCO,28 but apparently in our separation system the microgel particles effectively shield the water-soluble components from the IL and the equilibrium concentrations of the IL in water were below the denaturation threshold.
 |
| Fig. 5 Gel electrophoresis (native). M: Molecular weight marker, Std: RuBisCO standard, CS: control sample supernatant after cell disruption, Aq: aqueous phase of emulsion before adding the ionic liquid containing the disrupted cells in the same amount as the samples, 1 minute: aqueous phase after 1 minute of mixing, and 180 min: aqueous phase after 180 minutes of mixing. | |
The extraction efficiency of astaxanthin (approx. 62%) is lower compared to that using conventional solvent extraction methods2 (approx. 98%) and can be attributed to mass transfer limitation as the pigment has to be extracted from the broken cells and move across the microgel particles to the IL phase where it is soluble. However, the extraction can be optimised by modifying the biomass loading, using a monodisperse emulsion, the use of another IL, etc. The results demonstrate the feasibility of using microgel-stabilised IL emulsions for separating hydrophilic and hydrophobic components from a complex matrix such as microalgal biomass and simultaneously retaining the functionality of separated components.
Continuous extraction process
A continuous process is preferred over a batch system, primarily because it is less time consuming. As a proof of concept for the use of microgel-stabilized IL emulsions in a continuous separation process, a test run was made using the set-up depicted in Fig. 1. Samples were taken from the mixing vessel at different time intervals, centrifuged to sediment the emulsion and finally analysed. It was observed that the astaxanthin extraction efficiency was ∼45% and that the protein content was ≥85% (see Fig. 6) after 30 minutes, comparable to the batch experiment with similar residence time. However, further improvements in the set-up, such as the use of a top stirrer instead of a magnetic stirrer, could help by increasing the contact time between the phases and thus improving the mass transfer rates. Additionally, the separation between the phases can be enhanced by using a temperature-controlled settler since the polyNIPAm particles collapse at temperatures higher than 32 °C, which promotes the breaking of emulsion.18 Before back extraction, we can break the emulsion with a temperature switch and then clean the ionic liquid with ethylacetate.20 The microemulsion particles can be centrifuged, recovered and reused again so that recycling of the components is feasible.
 |
| Fig. 6 Extraction yield (%) of protein (closed symbols) and astaxanthin (open symbols) as a function of time (min) in a continuous set-up. | |
Conclusion
The ability of microgel-stabilised IL-in-water emulsions to simultaneously separate hydrophilic and hydrophobic components from a complex matrix was investigated in this work using microalgal biomass as an example. The hydrophobic pigment, astaxanthin from H. pluvialis cracked cells, is extracted in the IL droplets and the proteins remain in the continuous aqueous phase of the emulsion. The astaxanthin extraction efficiency obtained in the process was ∼62% and that of proteins in the aqueous phase is on average above 90% and in many experiments close to 100%. The proteins recovered in the aqueous phase remained in their native state after separation. This success is attributed to the fact that our system is free of surfactants, the polyNIPAm particles are inert towards fragile proteins and the solubility of the IL in water was below the denaturation threshold of proteins. This sets our method apart from other separation technologies, such as alkaline or solvent extraction which leads to (protein) denaturation, or classical emulsion-based technologies that are even more likely to produce denatured products due to the action of the surfactants/oil. The system provides a promising novel alternative for the separation of diverse biomolecules from complex matrices and has potential for analytical applications. Future research should focus on the separation of biomolecules of interest from diverse complex matrices such as bacteria, yeast, and fungi. We believe that this is realistic because the system has the intrinsic option to select the IL that is least harmful for the proteins of interest while keeping a high efficiency to take up hydrophobic components. This optimisation option is linked to the fact that polyNIPAm microgels are universal emulsifiers used to formulate many IL–water emulsions. A perspective and approach for continuous processing is presented. Further optimization studies are needed to improve the process kinetics and economics.
Conflicts of interest
There are no conflicts to declare.
Acknowledgements
The authors would like to thank the Dutch “Toegepaste en Technische Wetenschappen” (TTW), which is the applied division of the Dutch Scientific Organisation NWO, and the Institute for Sustainable Process Technology (ISPT) for the financial support.
Notes and references
- P.-A. Albertsson, Nature, 1958, 182, 709–711 CrossRef CAS PubMed.
- C. Yin, S. Yang, X. Liu and H. Yan, Chin. J. Chem. Eng., 2013, 21, 776–780 CrossRef CAS.
- S. P. Cuellar-Bermudez, I. Aquilar-Hernandez, D. L. Cardenas-Chavez, N. Ornelas-Soto, M. A. Romero-Ogawa and R. Parra-Saldivar, Microb. Biotechnol., 2014, 8, 190–209 CrossRef CAS PubMed;
A. J. J. Straathof, 2.57 - The Proportion of Downstream Costs in Fermentative Production Processes A2 - in: Comprehensive Biotechnology, ed. M. Moo-Young, Academic Press. Burlington, 2nd edn, 2011, pp. 811–814 CrossRef; V. C. A. Orr, N. V. Plechkova, K. R. Seddon and L. Rehmann, ACS Sustainable Chem. Eng., 2016, 4, 591–600 CrossRef; J.-M. Roux, H. Lamotte and J.-L. Achard, Energy Procedia, 2017, 112, 680–688 CrossRef.
- M. Vanthoor-Koopmans, R. H. Wijffels, M. J. Barbosa and M. H. M. Eppink, Bioresour. Technol., 2012, 135, 142–149 CrossRef PubMed.
- J. L. Burguera and M. Burguera, Talanta, 2012, 96, 11–20 CrossRef CAS PubMed.
- Y. V. Wu and G. E. Inglett, J. Food Sci., 1974, 39, 218–225 CrossRef CAS.
- A. M. Klibanov, Trends Biotechnol., 1997, 15, 97–101 CrossRef CAS PubMed.
- K. Bhuyan, Biopolymers, 2010, 93, 186–199 CrossRef PubMed.
- P. L. Luisi, M. Giomini, M. P. Pileni and B. H. Robinson, Biochim. Biophys. Acta, Rev. Biomembr., 1988, 947, 209–246 CrossRef CAS.
-
E. P. Melo, M. R. Aires-Barros and J. M. S. Cabral, Biotechnology Annual Review, Elsevier, 2001, pp. 87–129 Search PubMed.
- R. P. Swatloski, S. K. Spear, J. D. Holbrey and R. D. Rogers, J. Am. Chem. Soc., 2002, 124, 4974–4975 CrossRef CAS PubMed.
-
R. P. Swatloski, R. D. Rogers and J. D. Holbrey, US 6824599, The University of Alabama, 2002.
-
P. Saling, in: Sustainable Solutions for Modern Economies, ed. R. Höfer, RSC Green Chem No. 4, Cambridge: RSC Publ., 2009, pp. 25–36 Search PubMed.
- J. Hulsbosch, D. E. De Vos, K. Binnemans and R. Ameloot, Biobased Ionic Liquids, ACS Sustainable Chem. Eng., 2016, 4, 2917–2931 CrossRef CAS; D. Klein-Marcuschamer, B. A. Simmons and H. W. Blanch, Biofuels, Bioprod. Biorefin., 2011, 5, 562–569 CrossRef; A. George, A. Brandt, K. Tran, S. M. S. N. S. Zahari, D. Klein–Marcuschamer, N. Sun, N. Sathitsuksanoh, J. Shi, V. Stavila, R. Parthasarathi, S. Singh, B. M. Holmet, T. Welton, B. A. Simmons and J. P. Hallett, Green Chem., 2015, 17, 1728–1734 RSC.
- Z. Qiu and J. Texter, Curr. Opin. Colloid Interface Sci., 2008, 13, 252–262 CrossRef CAS.
-
M. Freemantle, Chemical & Engineering News Archive, 1998, vol. 76, pp. 32–37 Search PubMed.
- R. D. Rogers and K. R. Seddon, Science, 2003, 302, 792–793 CrossRef PubMed.
-
A. A. Rosatella and C. A. M. Afonso, Chapter 2. Ionic Liquids in the Biorefinery Concept: Challenges and Perspectives, The Royal Society of Chemistry, 2016, pp. 38–64 Search PubMed.
- J. Chen, J. Cao, W. Gao, L. W. Qi and P. Li, Analyst, 2013, 138, 5933–5941 RSC.
- Q.-X. Mao, H. Wang, Y. Shu, X.-W. Chen and J.-H. Wang, RSC Adv., 2014, 4, 8177–8182 RSC.
- H. J. M. Monteillet, M. J. Workamp, J. Appel, J. M. Kleijn, F. A. M. Leermakers and J. H. B. Sprakel, Adv. Mater. Interfaces, 2014, 1, 1–9 CrossRef.
- H. J. M. Monteillet, M. J. Workamp, X. Li, B. Schuur, J. M. Kleijn, F. A. M. Leermakers and J. H. B. Sprakel, Chem Commun., 2014, 50, 12197–12200 RSC; R. T. Lorentz and G. R. Cysewski, Trends Biotechnol., 2000, 18, 160–167 CrossRef CAS.
- R. K. Desai, R. K. M. Streefland, R. H. Wijffels and M. H. M. Eppink, Green Chem., 2014, 16, 2670–2679 RSC.
- I. Higuera-Ciapara, L. Félix-Valenzuela and F. M. Goycoolea, Crit. Rev. Food Sci. Nutr., 2006, 46, 185–196 CrossRef CAS PubMed.
-
Y. Liang, T. Kashdan, C. Sterner, L. Dombrowski, I. Petrick, M. Kröger and R. Höfer, Algal Biorefineries, in: Industrial Biorefineries and White Biotechnology, ed. A. Pandey, R. Höfer, M. Taherzadeh, K. Madhavan Nampoothiri and C. Larroche, Elsevier, Amsterdam, Oxford, Waltham, 2015, pp. 35–90 Search PubMed.
- Md. M. R. Shah, Y. Liang, J. J. Cheng and M. Daroch, Front. Plant Sci., 2016, 7, 531 Search PubMed.
-
J. M. Berg, J. L. Tymoczko and L. Stryer, Biochemistry, W. H. Freeman, New York, 5th edn, 2002, section 20.1 Search PubMed.
- R. K. Desai, M. Streefland, R. H. Wijffels and M. H. M. Eppink, Green Chem., 2016, 18, 1261–1267 RSC.
|
This journal is © The Royal Society of Chemistry 2018 |