DOI:
10.1039/C8RA07875K
(Paper)
RSC Adv., 2018,
8, 37765-37773
Facile preparation of degradable multi-arm-star-branched waterborne polyurethane with bio-based tannic acid
Received
21st September 2018
, Accepted 3rd November 2018
First published on 9th November 2018
Abstract
In this research, biodegradable multi-arm-star-branched waterborne polyurethanes (MWPUs) were prepared by incorporation of bio-based material (tannic acid, TA) in the structure of waterborne polyurethanes. The prepared MWPUs were characterized by UV-vis spectrometry and FT-IR spectrometry, confirming the presence of TA in MWPUs. The results of DSC and TGA demonstrated that the incorporation of TA remarkably enhanced the thermal stability of MWPUs. The mechanical strength test indicated that the Young's modulus and tensile strength of the waterborne polyurethanes after incorporation of TA were significantly improved due to the increase of structural rigidity, hydrogen bonding and the molecular interactions of the TA-based MWPU chains. In addition, the synthesized TA-based MWPUs also exhibited excellent antioxidation capacity and outstanding biodegradation property. Given these excellent properties and the sustainability of TA, the developed TA-based MWPUs exhibited great potential in a wide range of practical applications.
Introduction
Eco-friendly waterborne polyurethanes (WPUs) have gained increasing attention in a wide range of applications including coatings, adhesives, elastomers and biomedicine due to their good flexibility, non-toxicity and non-flammability as well as their reduced volatile organic compounds (VOCs) release compared with their solvent-based counterparts.1–3
Recently, bio-based raw materials such as castor oil and soybean oil have been exploited as components to synthesize WPUs, providing an effective and efficient approach for developing bio-based WPUs.4–6 The biological polyurethane material is non-toxic, biodegradable and biocompatible, which is very promising in commercial applications. The degradation properties of biodegradable materials as medical materials are of interest, as a long-term material implanted in the body is generally not expected to degrade. But when used as similar tissue engineering scaffolds, medical adhesives and drug sustained-release materials, it is hoped that they can be gradually degraded in the body. Besides, biodegradable multi-arm-star-branched waterborne polyurethane can be used to make biodegradable condoms.7–10
In this research, biodegradable multi-arm-star-branched waterborne polyurethanes (MWPUs) were prepared by incorporation of bio-based material (tannic acid, TA) in the structure of waterborne polyurethanes. The structure of the prepared MWPUs was characterized with UV-vis spectrometer and FT-IR spectrometer. The effects of incorporation of TA on the thermal stability and mechanical properties of WPUs were investigated. Furthermore, the antioxidation capacity of TA-based MWPUs was tested via DPPH and hydroxyl radical scavenging assay. Finally, the biodegradation behaviour of TA-based MWPUs in simulated body fluid and natural soil was discussed.
Experimental
Materials
Bis(hydroxymethyl) propionic acid (DMPA) was purchased from Shanghai Demand Chemical Co., Ltd, China. Poly (tetramethylene ether glycol) (PTMG, Mn = 2000) and isophorone diisocyanate (IPDI) were obtained from Jining Baichuan Chemical Co., Ltd, China. L-Ascorbic acid and di(phenyl)-(2,4,6-trinitrophenyl) iminoazanium (DPPH) were obtained from Sigma Aldrich (St. Louis, MO, USA). Dibutyltin dilaurate and stannous octoate were provided by Jiangsu Yoke Technology Co., Ltd, China. Triethylamine (TEA), diethylene glycol (DEG), tannic acid (TA), tetrahydrofuran (THF), ethanol, hydrogen peroxide, 2-hydroxybenzoic acid, ferrous sulfate, sodium chloride, potassium chloride, sodium dihydrogen phosphate and potassium dihydrogen phosphate were obtained from Sinopharm Chemical Reagent Shanghai Co., Ltd., China and used as received.
Preparation of TA-based MWPUs and films
The synthesis process of TA-based MWPUs is shown in Scheme 1. A typical procedure is as follows: PTMG-2000, TA and DMPA were preprocessed to remove the moisture before using via drying them in a vacuum oven at 110 °C, 75 °C and 75 °C for 2 h, respectively. Then, PTMG-2000 (100 g, 0.05 mol) was placed in a 50 ml four-neck round-bottom flask under 65 °C, followed by addition of IPDI (33 g, 0.15 mol). Afterwards, the mixture was heated to 80 °C and kept for 2 h to prepare the –NCO-ended polyurethane prepolymer. DMPA (7.98 g, 0.06 mol), DEG, dibutyltin dilaurate and stannous octoate were then added for further reaction of 4 h to get the polyurethane prepolymer with –COOH groups. The mixture was cooled to 25 °C, and then TA solution dissolved in THF was added, followed by keeping the reaction at 60 °C for 5 h. After that, the mixture was placed in a water bath until the temperature dropped to 25 °C, and was then neutralized by slowly adding TEA (6.02 g, 0.06 mol)under stirring (175–200 rpm) in 45 min. Subsequently, the polymer mixture was vigorously stirred (1400–1500 rpm) for 30 min and emulsified with a certain amount of deionized water to obtain a WPU dispersion. Finally, the WPU solution with solid content of 35% was obtained by removal of THF via vacuum evaporation. A series of WPUs (WPU0, MWPU1, MWPU2, MWPU3) were prepared via adjusting the ratios of TA (Table 1).
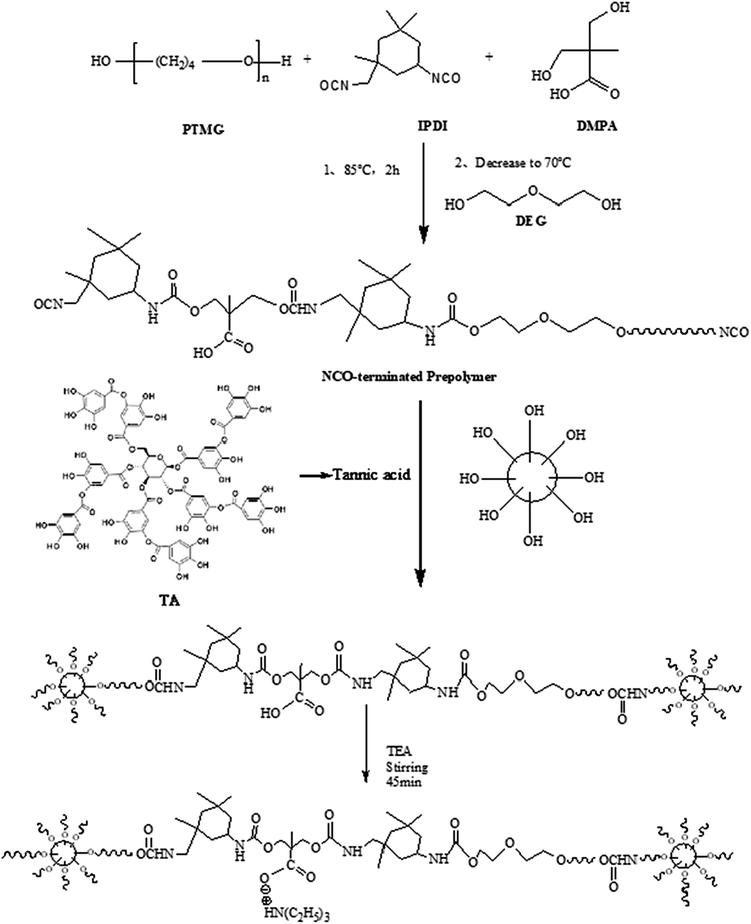 |
| Scheme 1 Synthesis of TA-based MWPUs. | |
Table 1 Composition of reactants for synthesis of WPUs and TA-based MWPUs
Materials |
WPU0 |
MWPU1 |
MWPU2 |
MWPU3 |
PTMG (mol) |
1.00 |
1.00 |
1.00 |
1.00 |
IPDI (mol) |
3.00 |
3.00 |
3.00 |
3.00 |
DMPA (mol) |
1.20 |
1.20 |
1.20 |
1.20 |
DEG (mol) |
0.30 |
0.23 |
0.15 |
0.18 |
TA (mol) |
0 |
0.05 |
0.10 |
0.15 |
TEA (mol) |
1.20 |
1.20 |
1.20 |
1.20 |
NCO/OH (ratio) |
1.20 |
1.20 |
1.20 |
1.20 |
The polyurethane films were prepared by pouring WPUs and MWPUs dispersions into PTFE module, and then drying at room temperature for 24 h. Then as-prepared films with thickness of 0.5 mm was further dried in a vacuum oven to a constant weight at 60 °C and were then stored in a desiccator prior to testing and characterization.
Free radical scavenging assay
DPPH free radical scavenging assay. The mechanism of DPPH radical scavenging assay was shown in Fig. 1. Typically, DPPH˙ is an available organic nitrogen radical in ethanol solution and has strong absorption maximum at 517 nm. DPPH˙ can accept hydrogen from an antioxidant to form DPPH2, which results in the colour of solution turning from purple to yellow and the decrease of UV absorption at 517 nm. Therefore, the antioxidant capacity can be easily evaluated by measurement of the UV absorption at 517 nm of DPPH˙ solution.
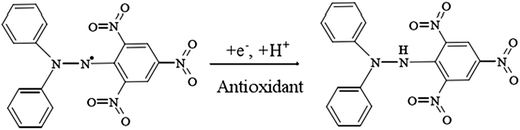 |
| Fig. 1 Mechanism of DPPH radical scavenging assay. | |
In our study, to test the radical scavenging activity, 1 ml of 0.1 M DPPH (in 50% ethanol solution) was mixed with 1 ml of tannic acid-based hyperbranched WPUs solution (0.2–2.0 mg ml−1 in double distilled water). The reaction mixture was incubated at room temperature for 20 min under dark conditions. Then the absorbance was measured at 517 nm, and the scavenging activity of tannic acid-based hyperbranched WPUs was measured by using the following eqn (1),
|
 | (1) |
where
A1 is the absorbance of DPPH solution with the presence of samples and
A0 is the absorbance of the control. For the control, a same volume of DI water was added into the test tube instead of polymeric solution. In addition,
L-ascorbic acid was used as a positive control.
11–13
Hydroxyl free radical scavenging assay. To measure the hydroxyl radical scavenging, the prepared multi-arm-star-branched WPUs was dissolved in deionized water with concentration of 0.2–2.0 mg ml−1 2 ml of polymeric solution was taken out and added into the test tube. Then, ferrous sulfated aqueous solution (0.1 M) and salicylic acid solution (0.1 M) in ethanol were added into the test tube, followed by adding H2O2 (0.1 M, 2 ml). The mixture was shaken well and incubated in 37 °C water bath for 30 min. The absorbance was measured at 510 nm, and the scavenging ability for hydroxyl radicals was calculated by using the following eqn (2), |
 | (2) |
where Aa is the absorbance of the samples and A2 is the absorbance of the control. For the control, a same volume of DI water was added into the test tube instead of polymeric solution. In addition, L-ascorbic acid was used as a positive control.14–16
Degradation behaviour of MWPUs in the simulated body fluid
Before testing degradation behaviour of WPU and multi-arm-star-branched WPU films in the simulated body fluid, the film coupons with dimension of 10 mm × 10 mm × 1 mm were dried in a vacuum oven to a constant weight at 50 °C and weighed via digital balance (W0). Then the coupons were immersed into a glass vial containing 10 ml of the PBS simulated body fluid (pH = 7.4) which was prepared by dissolving 0.8 g of NaCl, 0.2 g of KCl, 1.44 g of NaHPO4, and 0.24 g of KH2PO4 in 1 L of DI water, followed by incubation at 37 °C. The PBS simulated body fluid was replaced once a day, and the coupons were taken out at predetermined time intervals, washed with DI water for three times and dried in the vacuum oven at 45 °C. The weight of dried coupons (Wt) were then measured. The weight loss ratio was calculated using the eqn (3), |
 | (3) |
where W0 and Wt are the weights of the samples before and after degradation.17,18
Biodegradation study of multi-arm-star-branched WPU in soil
The biodegradation behaviour of multi-arm-star-branched WPU in soil was further investigated following a previously reported protocol. Typically, the soil taken from the lawn located in the Department of Material Science of Tongji University was dried under sunlight for three days. Then the soil was shattered, and the grain of sand was removed from soil. The treated soil was placed in a 250 ml beaker, and 100 ml of DI water was then added into the beaker. Before introducing the film samples into the pasty soils, each sample was cut into pieces of dimension of 10 mm × 10 mm × 1 mm and dried in a vacuum oven to a constant weight (Wi) at 50 °C. The beaker containing the film samples were buried in a soil bed at a depth of around 15–20 cm from the top of the ground surface. The biodegradation experiments were carried out under ambient conditions with regular addition of water (except in rainy days). Samples were taken out carefully from each beaker after 30 days, 60 days, 90 days and 120 days, respectively, and washed repeatedly with water to remove the adhered soil from the surface of the films. Then the samples were dried in a vacuum oven until a constant weight was obtained. Finally, the weight of samples was measured (Wt). The weight loss ratio was calculated using the eqn (4), |
 | (4) |
where Wi and Wt are the weights of the samples before and after degradation.19–22
Characterization
The IR spectra of the prepared multi-arm-star-branched WPUs were obtained using a Nicolet 6700 FT-IR Spectrometer (Thermo Scientific), and the structure of the film samples before and after degradation was measured under the attenuated total reflection (ATR) mode. To evaluate the relative molecular weight and its distribution of the synthesized multi-arm-star-branched WPUs, gel permeation chromatography (GPC) spectrum was measured in THF with a concentration of 2 mg ml−1. The flow rate and temperature were set at 1.0 ml min−1 and 40 °C, respectively. The intrinsic viscosity [ηr] of the synthesized multi-arm-star-branched WPUs solutions was determined at 20 °C with a Bohlin Rotational Rheometer (Malvern Instrument, UK) equipped with cone-and-plate geometry (40 mm diameter, cone angle 4°). The rotate speed was 300 radians per second. UV-vis absorption spectra of the WPUs and multi-arm-star-branched WPUs samples were recorded on a HITACHI U-1800 spectrophotometer at room temperature. The concentration of the WPUs and multi-arm-star-branched WPUs solutions was 0.1 mg ml−1. The surface morphology of the film samples prepared with WPUs and multi-arm-star-branched WPUs was observed using a scanning electron microscopy (SEM, FEI, USA). All samples were coated with 10 nm thick platinum (Pt) before SEM observation. To study the thermal stabilities of the WPUs and multi-arm-star-branched WPUs, thermogravimetric analysis (TGA) was performed on TA Instruments Q500 (New Castle, DE, USA) in N2 atmosphere with a purge rate of 50 ml min−1. The data was recorded from 20 to 800 °C with a scanning rate of 20 °C min−1. Besides, differential scanning calorimetry (DSC) was also carried out on a TA Instruments DSC-Q20 from −80 to 200 °C with a heating/cooling rate of 10 °C min−1 in nitrogen atmosphere. To study the mechanical properties of the samples, the tensile strength, Young's modulus and elongation at break were studied by using a tensile tester (INSTRON5565, USA) at a speed of 50 mm min−1 at room temperature. Three specimens were tested and averaged for each sample. The specimen was molded into 45 mm long, 8 mm wide and 0.2 mm thick.
Results and discussion
Characterization of MWPUs with TA
As shown in Scheme 1, there are five branches in the structure of TA, which are generating from a pyranose heterocycle, 25 hydroxyl groups, and 10 ester linkages. Therefore, in our study, bio-based tannic acid was used as an alternative to polyol to synthesize the multi-arm-star-branched WPUs. To confirm the presence of TA in the synthesized MWPU1, MWPU2 and MWPU3, the UV-vis absorption spectroscopy was used to track the characteristic absorbance peak of the TA at around 280 nm. As shown in Fig. 2a, the prominent absorption peaks were observed for MWPU1, MWPU2 and MWPU3, and the intensity of peaks of the synthesized WPUs exhibited an obvious increase ranging from MWPU1 to MWPU3, indicating the higher TA content in the structure of MWPU3 compared with MWPU1 and MWPU2.
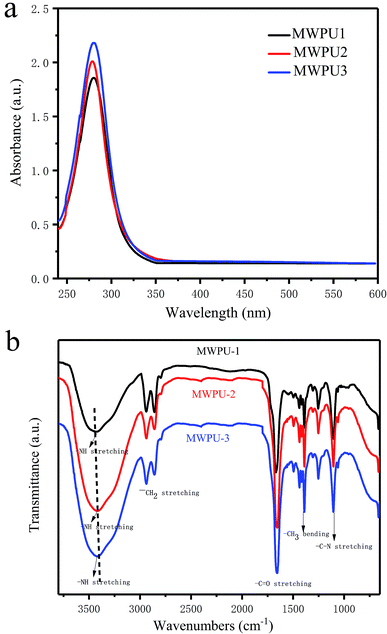 |
| Fig. 2 UV-vis spectra (a) and FT-IR spectra (b) of synthesized MWPU1, MWPU2 and MWPU3. | |
FT-IR spectroscopy was further employed to assess the chemical structures of synthesized MWPUs with different TA content. As shown in Fig. 2b, for the spectrum of MWPU1, the absorption peaks focused at 3422 cm−1, 1705 cm−1 and 1556 cm−1 are mainly attributed to the symmetric stretching vibration of −N–H bond, –C
O group and the bending vibration of −N–H bond, respectively, indicating the successful formation of carbamate in MWPU1. The peaks observed at 2940 cm−1, 2860 cm−1, 1105 cm−1 and 1254 cm−1 are ascribed to the symmetric stretching vibration of –CH2 group, the asymmetric stretching vibration of –CH3 group, the symmetric blending vibration of C–O–C bond and the stretching vibration of –C–N bond. In addition, the peak positions of –C–N bonds in the spectra of MWPU2 and MWPU3 exhibited an obvious blue shift, which is due to the hydrogen bonds formed between the catechol groups of TA and –C–N bond and –C
O group of carbamates, and the number of hydrogen bond increases with the increase of TA content in MWPU2 and MWPU3. This result further confirmed the presence of TA in the synthesized MWPU1, MWPU2 and MWPU3.
Then the molecular weight and distribution of the synthesized MWPU1, MWPU2 and MWPU3 were measured via GPC and the results presented in Table 2 revealed that the weight average molecular weight (Mw) of the obtained MWPU3 exhibited a significant increase to 47
200 Da from 33
100 Da of MWPU1 with the increase of the addition amount of TA in the reaction system. The polydispersity index (PDI) of the obtained MWPU1, MWPU2 and MWPU3 was measured to be 1.40, 1.34, and 1.34, respectively, indicating that all MWPUs have narrow molecular weight distribution and the TA content in the MWPUs did not affect the molecular weight distribution significantly. Thus, the molecular weights of the MWPUs can be well controlled via adjusting the addition amount of TA in the synthesis process, without affecting the molecular weight distribution. The intrinsic viscosities of the obtained MWPU1, MWPU2 and MWPU3 solution were determined with a rotational rheometer. Compared with the reported WPUs, MWPUs prepared with TA exhibited clearly reduced intrinsic viscosities (0.441, 0.349 and 0.321 mg L−1 for MWPU1, MWPU2 and MWPU3, respectively), which is due to the very compact three-dimensional structures of MWPU1, MWPU2 and MWPU3 that reduces the chain interactions significantly. This result is also generally consistent with the previously reported results of other hyperbranched polymers.
Table 2 Weight average (Mw) and number average (Mn) molecular weight, PDI and solution viscosity of synthesized MWPUs
Parameter |
MWPU1 |
MWPU2 |
MWPU3 |
Mw |
33 100 |
45 000 |
47 200 |
Mn |
23 600 |
33 600 |
35 200 |
PDI |
1.40 |
1.34 |
1.34 |
Viscosity (ml g−1) |
0.441 |
0.349 |
0.321 |
The influences of TA on the thermal properties of the synthesized MWPUs
To evaluate the influences of TA on thermal properties of MWPUs, the samples of WPU0 and MWPUs were analysed by DCS and TGA. As shown in Fig. 3, an obvious absorption peak at 15 °C appeared for the sample of WPU0 due to the melting of the soft segment, indicating a certain degree of micro-crystallization. However, the other MWPUs samples did not have any melting absorption peaks, indicating that the introduction of rigid tannic acid disturbed the crystallization region of the pristine waterborne polyurethane.
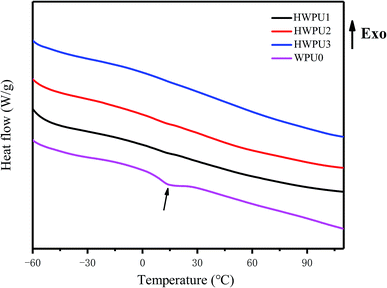 |
| Fig. 3 DSC curves of synthesized WPU0 and MWPUs. | |
As shown in Fig. 4, the synthesized WPU0 and MWPUs exhibited three-stage thermal degradation process: (i) at the first stage, the decomposition of some thermolabile urethane bonds and ester bonds occurred around 250 °C; (ii) the second stage was corresponding to the decomposition of the aromatic rings from tannic acid (TA) around 300 °C; (iii) the third stage was around 420 °C, involving the decomposition of the soft segment on the polyurethane backbone. Furthermore, the temperatures at different weight loss ratios for the samples of WPU0 and MWPUs were listed in Table 3. It could be seen that as the content of TA increases, the thermal decomposition temperature of the polyurethanes increases. For example, the T10% of WPU0 and MWPU3 were 269.66 °C and 277.02 °C. The T30% of WPU0 and MWPU3 were 365.44 °C and 378.91 °C. So, the introduction of TA increased the thermal decomposition temperature of the waterborne polyurethanes. This was not only because of the introductive large amount of thermally-stable benzene ring, but also due to the secondary interactions between the ester bonds and heterocycles. Besides, it should be noted that the effect of TA was mainly on the first two thermal decomposition stages. Besides, residual polyurethanes at 700 °C enhanced as the content of introductive TA increased.23–25
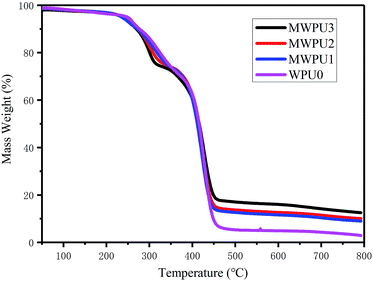 |
| Fig. 4 TGA curves of synthesized WPU0 and MWPUs. | |
Table 3 Thermal decomposition temperature corresponding to different numerical Thermogravimetry
Sample |
T10% (°C) |
T20% (°C) |
T30% (°C) |
T40% (°C) |
T50% (°C) |
WPU0 |
269.66 |
300.76 |
365.44 |
400.23 |
415.73 |
MWPU1 |
271.84 |
308.46 |
373.58 |
401.23 |
411.39 |
MWPU2 |
272.18 |
317.47 |
376.58 |
402.72 |
414.06 |
MWPU3 |
277.02 |
324.83 |
378.91 |
404.39 |
416.06 |
The influences of TA on the mechanical properties of the synthesized MWPUs
The mechanical properties of the waterborne polyurethanes were analysed by the Young's modulus, tensile strength and elongation at break. The results were shown in Fig. 5 and Table 4. Young's modulus is a physical quantity that describes the deformation resistance of solid materials, which depends only on the physical properties of the material itself. The size of young's modulus indicates the rigidity of the material. The larger young's modulus is, the less likely it is to deform.
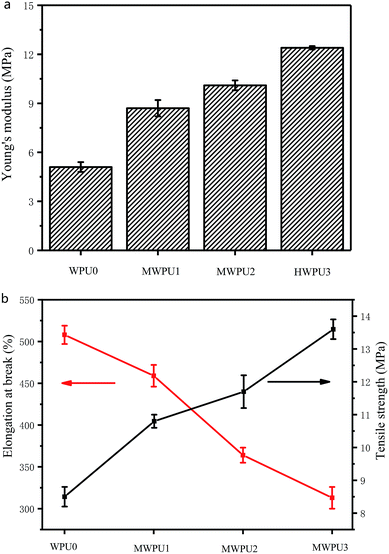 |
| Fig. 5 Young's modulus (a), tensile strength and elongation at break (b) of synthesized WPU0 and MWPUs. | |
Table 4 Young's modulus, tensile strength and elongation at break of the synthesized WPU0 and MWPUs
Sample |
Young's modulus (MPa) |
Tensile strength (MPa) |
Elongation at break (%) |
WPU0 |
5.1 ± 0.3 |
8.5 ± 0.3 |
508 ± 11 |
MWPU1 |
8.7 ± 0.5 |
10.8 ± 0.2 |
459 ± 13 |
MWPU2 |
10.1 ± 0.3 |
11.7 ± 0.5 |
364 ± 9 |
MWPU3 |
12.4 ± 0.1 |
13.6 ± 0.3 |
313 ± 13 |
As the content of TA increases, the Young's modulus and tensile strength of the waterborne polyurethanes increased. Specifically, the Young's modulus of WPU0 and MWPU3 were 5.1 MPa and 12.4 MPa, respectively. Meanwhile, the tensile strength of WPU0 and MWPU3 were 8.5 MPa and 13.6 MPa, respectively. The increase of the Young's modulus and tensile strength of the waterborne polyurethanes after incorporation of TA is attributed to the fact that the introduction of TA not only enhances the structural rigidity of waterborne polyurethanes, but also increases the hydrogen bonds and the molecular interactions of waterborne polyurethanes, thus ultimately enhancing the mechanical strength of waterborne polyurethanes. However, it is worth noting that the increase of TA content in the synthesized MWPUs resulted in a decrease in the elongation at break of the waterborne polyurethanes (Table 4), which is due to the decrease of the flexibility of chains of MWPUs.
The antioxidation capacity of the synthesized MWPUs
DPPH radical scavenging assay. Typically, the antioxidation capacity of materials is mainly depending on their ability to donate electron to radical. To investigate the effects of incorporation of TA in MWPUs on their antioxidation capacity, the DPPH radical scavenging activities of the synthesized WPU0 and MWPUs were measured. As illustrated in Fig. 6, the WPU0 without TA did not exhibited any DPPH radical scavenging activity, whereas, the synthesized TA-based MWPUs showed outstanding radical scavenging activity. Besides, the radical scavenging activity of TA-based MWPUs enhanced as TA content increases. This result demonstrated that TA is the main component in MWPUs contributing to the antioxidant activity. In addition, the radical scavenging activity of MWPUs also get strengthen as the concentration of MWPUs increase. Particularly, when the solution concentration of MWPUs is 2 mg ml−1, the DPPH radical scavenging efficiency is up to 67%. This excellent DPPH radical scavenging activity is mainly attributed to the catechol groups of TA that can serve as electron donors and react with free radicals effectively to convert them into more stable products.26,27
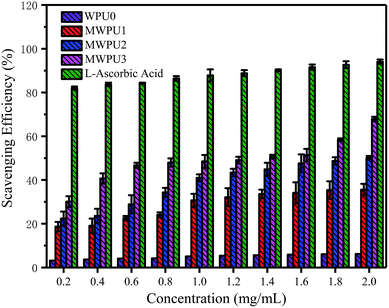 |
| Fig. 6 DPPH radical scavenging efficiency of synthesized WPU0 and MWPUs. | |
Hydroxyl radical scavenging assay. Hydroxyl radical, as the most toxic radical among the reactive oxygen species (ROS) and one of the most active radicals, can react with a range of substances in human body, thus leading to a variety of diseases. Given that, hydroxyl radical scavenging activities of the synthesized WPU0 and MWPUs were further investigated. As presented in Fig. 7, WPU0 did not exhibit any scavenging activities for hydroxyl radical, and the hydroxyl radical scavenging activities of TA-based MWPUs increase with the increase of TA content and the concentration of MWPUs in solution. These results are generally consistent with the results of DPPH radical. Particularly, when the concentration of MWPU3 solution is 2 mg ml−1, the hydroxyl radical scavenging efficiency is over 50%, further confirming the excellent antioxidation capacity of the TA-based MWPUs.
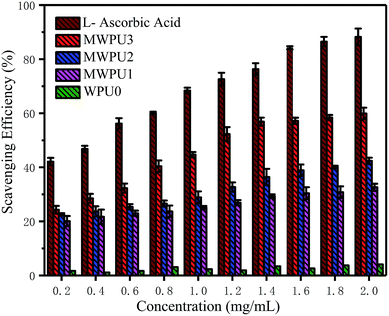 |
| Fig. 7 Hydroxyl radical scavenging efficiency of synthesized WPU0 and MWPUs. | |
The degradation behaviours of WPU0 and MWPUs
The degradation behaviours of WPU0 and MWPUs were studied by determining weight loss over time under two different conditions including in simulated body fluid and in soil. Fig. 8a represented the degradation curves of WPU0 and MWPUs in simulated body fluid (PBS solution). It is found that after 5 weeks of degradation in simulated body fluid, the TA-based MWPUs exhibited much high weight loss compared with WPU0, indicating that TA-based MWPUs have better degradation performances. And as the increase of TA content in MWPUs, the weight loss of TA-based MWPUs rising. For instance, the weight loss of MWPU3 after 5 weeks' degradation is up to 18%, which is higher than the performance of MWPU1 and MWPU2. The outstanding degradation performance of MWPU3 is due to the presence of large number of ester bonds in the chains of MWPU3 that is liable to hydrolysis.
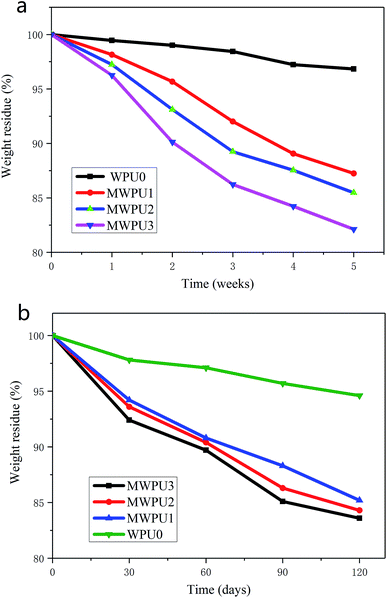 |
| Fig. 8 Degradation curves of synthesized WPU0 and MWPUs in simulated body fluid (a) and in soil (b). | |
The degradation behaviours of WPU0 and MWPUs in soil were also investigated and the results are shown in Fig. 8b. After 120 days degradation in soil, TA-based MWPUs exhibited 15–17% weight loss, which is much higher than 5% weight loss of WPU0, further confirming the better degradation performance of TA-based MWPUs. It is worth noting that although the weight loss of TA-based MWPUs also exhibited a same trend in soil with the increase of TA content in MWPUs, the increase of TA-based MWPUs in soil is much lower than that in simulated body fluid.
To further investigate the degradation process of MWPU3 in soil, the structure of MWPU3 and the surface morphology of MWPU3 films were characterized with FT-IR ATR and SEM, respectively. As shown in Fig. 9, significant changes of three peaks concentrate upon 2864–2940 cm−1, 1710 cm−1 and 1130 cm−1 have been observed. Particularly, the peak at 2864–2940 cm−1 is attributed to the –CH2 group and the peak intensity exhibited an obvious decrease after 120 days of degradation, which is mainly due to the degradation of C–H bond caused by the hydrocarbon-degrading bacteria. The second peak with significant change at 1710 cm−1, which is ascribed to the –C
O group. The peak intensity exhibited an apparent decrease after 60 days of degradation and this peak disappeared completely after 120 days. The degradation of –C
O group is mainly caused by hydrolysis and biodegradation of bacteria. The peak attributed to C–O–C bond (1130 cm−1) did not exhibited significant change after 60 days, whereas the peak intensity showed a clear decrease after 120 days of degradation, which is probably due to the degradation of enzymes or bacteria in soil. That result also indicated that the degradation of C–O–C bonds mainly occurred in last stage. According to these findings, the degradation of TA-based MWPUs is mainly due to degradation of ester bonds, C–H bonds and C–O–C bonds caused by the bacteria, enzymes and water existed in soil.28,29
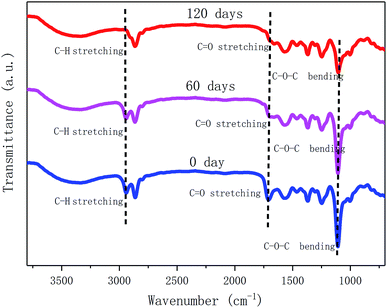 |
| Fig. 9 FT-IR spectra of MWPUs before and after 60 days and 120 days degradation. | |
The change of surface morphology of MWPU3 film during the degradation process was presented in Fig. 10. At the initial state, MWPU3 film exhibited a very smooth surface without any defect. However, as time goes, the surface of MWPU3 film become rough and some holes were observed on the film surface after 120 days of degradation, further confirmed the degradation of MWPU3.
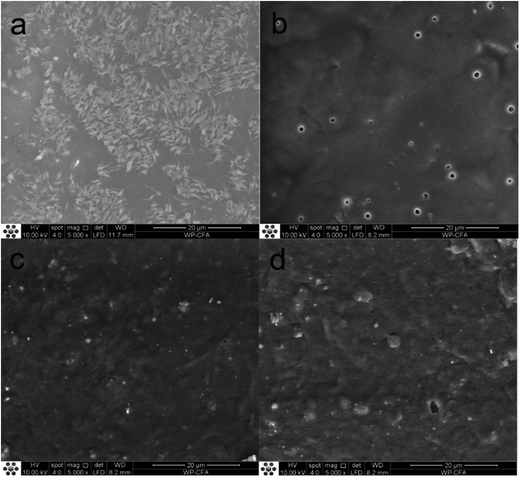 |
| Fig. 10 Change of surface morphology of MWPU3 film before and after degradation. (a) 0 days, (b) 30 days, (c) 60 days and (d) 120 days. | |
Conclusions
In this study, biodegradable MWPUs were prepared by incorporation of TA in the structure of waterborne polyurethane. The UV-vis spectra and FT-IR spectra of the synthesized MWPUs confirmed the presence of TA. The result of DSC and TGA demonstrated that the incorporation of TA enhanced the thermal stability of MWPUs, and Young's modulus and tensile strength of the waterborne polyurethanes after incorporation of TA were increased due to the increase of structural rigidity, hydrogen bonds and molecular interactions of the MWPU chains. Besides, the synthesized TA-based MWPUs exhibited excellent antioxidation capacity and outstanding biodegradation property. Along with these excellent properties and sustainability of TA, the developed TA-based MWPUs exhibited great potential in a wide range of practical applications. MWPU is non-toxic, biodegradable and biocompatible, which is very promising in commercial applications. The degradation property of biodegradable materials as medical materials is concerned, as a long-term material implanted in the body, it is generally not expected to degrade. But, when used as similar tissue engineering scaffolds, medical adhesives and drug sustained-release materials, it is hoped that they can be gradually degraded in the body. Besides, MWPU can be used to make biodegradable condoms.
Conflicts of interest
There are no conflicts to declare.
Acknowledgements
This work was financially supported by the National Natural Science Foundation of China (81571801, 81671944), the Fundamental Research Funds for the Central Universities and the Open Funds for Characterization of Tongji University.
References
- X. Ji, H. Wang and X. Ma, RSC Adv., 2017, 7(54), 34086–34095 RSC.
- A. Santamaria-Echart, L. Ugarte and C. García-Astrain, Carbohydr. Polym., 2016, 151, 1203–1209 CrossRef CAS PubMed.
- Z. Zhong, S. Luo and K. Yang, RSC Adv., 2017, 7(67), 42296–42304 RSC.
- B. B. R. Silva, R. M. C. Santana and M. M. C. Forte, Int. J. Adhes. Adhes., 2010, 30(7), 559–565 CrossRef CAS.
- K. Liu, Z. Su and S. Miao, RSC Adv., 2016, 6(38), 31698–31704 RSC.
- Z. Luo, Y. Shi and D. Zhao, Procedia Eng., 2011, 18(18), 37–42 CrossRef.
- I. Phiwchai, W. Yuensook and N. Sawaengsiriphon, Eur. J. Pharm. Sci., 2017, 114, 64 CrossRef PubMed.
- B. Ghosh, S. Gogoi, S. Thakur and N. Karak, Prog. Org. Coat., 2016, 90, 324–330 CrossRef CAS.
- X. Lai, Z. Hu and Y. Shen, Petrochem. Technol., 2017, 46(1), 83–89 CAS.
- W. Y. Li, Y. M. Cao and X. Q. Zhou, Appl. Mech. Mater., 2012, 249–250, 842–848 Search PubMed.
- D. Villaño, M. S. Fernández-Pachón, M. L. Moyá, A. M. Troncoso and M. C. García-Parrilla, Talanta, 2007, 71(1), 230–235 CrossRef PubMed.
- Q. Li, L. Qiu and W. Tan, RSC Adv., 2017, 7(67), 42225–42232 RSC.
- X. G. Zhang, L. Tang and R. L. Shang, Storage Process, 2017, 17(6), 39–46 Search PubMed.
- N. Singh and P. S. Rajini, Food Chem., 2004, 85(4), 611–616 CrossRef CAS.
- L. Yu, S. Haley and J. Perret, J. Agric. Food Chem., 2002, 50(6), 1619–1624 CrossRef CAS PubMed.
- T. Kokila, P. S. Ramesh and D. Geetha, Appl. Nanosci., 2015, 5(8), 911–920 CrossRef CAS.
- H. Nur Maulida, F. Qulub and A. F. Rosdiani, J. Biomimetics, Biomater. Biomed. Eng., 2016, 28, 78–84 Search PubMed.
- J. L. Ryszkowska, M. Auguścik and A. Sheikh, Compos. Sci. Technol., 2010, 70(13), 1894–1908 CrossRef CAS.
- J. Liu, X. Qi and D. Zhang, Adv. Mater. Sci. Eng., 2017, 3, 1–14 Search PubMed.
- B. L. Dou, A. N. Qiu-Feng and M. A. Jun-Jian, Appl. Chem. Ind., 2010, 39, 189–191 CAS.
- B. Ou, M. Chen and R. Huang, RSC Adv., 2016, 6(52), 47138–47144 RSC.
- J. Wang, P. Sun and Z. Zheng, Polym. Degrad. Stab., 2012, 97(11), 2294–2300 CrossRef CAS.
- G. Zheng, M. Lu and X. Rui, Appl. Surf. Sci., 2016, 399, 272–281 CrossRef.
- H. Fu, Y. Wang and X. Li, Compos. Sci. Technol., 2016, 126, 86–93 CrossRef CAS.
- X. Li, Y. Chen and S. Wu, Polym. Mater.: Sci. Eng., 2017, 33(7), 138–143 CAS.
- E. Montanari, A. Gennari and M. Pelliccia, Macromol. Biosci., 2016, 16(12), 1815 CrossRef CAS PubMed.
- M. Shin, K. Kim and W. Shim, ACS Biomater. Sci. Eng., 2016, 2(4), 687–696 CrossRef CAS.
- L. Cosgrove, P. L. Mcgeechan, G. D. Robson and P. S. Handley, Appl. Environ. Microbiol., 2007, 73(18), 5817 CrossRef CAS PubMed.
- J. C. Peeler, S. Schedin-Weiss and M. Soula, J. Biol. Chem., 2017, 292(52), 21623–21630 CrossRef PubMed.
|
This journal is © The Royal Society of Chemistry 2018 |