DOI:
10.1039/C8RA07601D
(Paper)
RSC Adv., 2018,
8, 34634-34649
Nanocharacterization of liposomes for the encapsulation of water soluble compounds from Cordyceps sinensis CS1197 by a supercritical gas anti-solvent technique†
Received
12th September 2018
, Accepted 24th September 2018
First published on 9th October 2018
Abstract
Nano-liposomes were designed for the sustained release of water soluble compounds from C. sinensis CS1197 using a supercritical gas anti-solvent (SC-GAS) method at various pressures, temperatures and Tween 80 concentrations. The SC-GAS method was compared to the Bangham method of liposome production in terms of mean diameter, coefficient of uniformity (Cu), encapsulation efficiency, morphology, viscosity and actual energy required for liposome formation. Liposome production via the SC-GAS method under optimized formulation conditions, i.e., 180 bar; 50 °C; 0.75% Tween 80; and a depressurization rate of 25 bar min−1, yielded nano-liposomes exhibiting the lowest Cu value (1.10 ± 0.012) with a mean diameter of 0.072 ± 0.002 μm and better encapsulation efficiencies of 75.48 ± 2.5, 74.9 ± 2.1 and 70.23 ± 2.9% for adenosine, cordycepin and polysaccharides, respectively. Nano-liposomes were characterized using FTIR, XRD, DSC and TGA techniques. The stability indices and viscosities of the prepared liposome suspensions indicated good stability of up to 2 months and near-Newtonian behavior. The in vitro release of CS1197 water soluble compounds exhibited biphasic and sustained release patterns.
Introduction
Cordyceps sinensis (CS) is a highly valued traditional Chinese medicine with various health benefits. C. sinensis is an excellent nourishment option for the kidneys, lungs and liver and is an effective aphrodisiac. Water soluble compounds, such as nucleosides and polysaccharides, from C. sinensis possess unbelievable pharmacological properties.1,2 C. sinensis water extract has been effectively used to treat various pathological conditions and has also been used in various functional food preparations. C. sinensis based therapies may face difficulties, such as a low therapeutic effect, due to their polar nature, which may cause increased intracellular absorption. Nucleosides in C. sinensis water extract are small molecules (<500 D), which are affected by rapid clearance and suboptimal biodistribution. To address current challenging tasks, like the development of sustained and targeted drugs or active principles, the development of nano-carriers with the best information from nano-science is a sound potential approach and is a major focus of current research.
Among the available nano-carriers, nano-liposomes have attracted much attention as potential carriers of drugs.3 The idea of nano-processing C. sinensis based active ingredients for the development of smart drug delivery systems, like those involving liposomes, has become an emerging trend to achieve targeted and sustained release. This is because liposomes are potent drug delivery systems because of their ability to meet several requirements, such as the suitable stability of active molecules, yield and efficiency of drug encapsulation, reproducibility of the micro/nano-particle quality and the drug release profile, and residual levels of organic solvent in the particles. Basically, liposomes are composed of one or more bilayers of amphipathic molecules (e.g., phospholipids) surrounded by an aqueous continuous phase. The major advantage of liposomal encapsulation is that both hydrophilic and hydrophobic molecules can be intercalated.4 Moreover the surfaces of liposomes offer flexibility for any modifications to achieve the site specific unloading of active principles and/or drugs.
Many methods have been developed for liposome production.5 The supercritical method is a novel approach through which liposomes can be made in a single step by making use of supercritical carbon dioxide (SCCO2).6 Zarena et al. (2011) reviewed variants of the method to produce submicron- and nano-particles using SCCO2, such as: the rapid expansion of supercritical solutions (RESS); the gas anti-solvent (GAS) method; the precipitation with compressed anti-solvent process (PCA); solution enhanced dispersion via supercritical fluids (SEDS); and the particles from gas-saturated solutions (PGSS) method.7 Further, GAS is a more universal process and offers greater flexibility than the other variants because the compounds to be encapsulated do not need to be dissolved in SCCO2. Both hydrophilic and hydrophobic compounds can be entrapped in lipid vesicles. Kadimi et al. (2007) claimed an intercalation efficiency (IE) of 20% for amphotericin B and, in a similar study, an IE of 28% for seabuckthorn leaf extract with a narrow range of size distribution (0.48–1.07 μm), using a SCCO2 method.8,9 Liposome preparation in batch mode via a modified supercritical fluid method improved particle sizes in the range of 265 and 214 nm.10,11 Further efforts have been made to decrease the particle size to 146 nm, with the superior characteristics of liposomes over traditional methods, and a similar study proposed the production of liposomes in a continuous mode using a supercritical method.12,13
Preliminary attempts to design liposomes using the GAS method8,9 have been successful in the submicron range (<1 μm) in the author's lab. To the best of our knowledge, this is the first study reporting the fed-batch mode development of nano-sized liposomes for the entrapment of water soluble compounds from C. sinensis CS1197. The major objective of the current study is to optimize the formulation variables, i.e., pressure, temperature, and Tween 80 concentration, to design nano-liposomes via a SC GAS method. The nano-liposomes produced via the SC method were well characterized using FTIR, DSC, TGA and XRD techniques. Liposomes prepared with the SC method were compared to those obtained with the Bangham method.
Experimental
Materials and methods
Materials. The CS1197 strain of C. sinensis was obtained from NTCC, FRI, Dehradun, India. Water extract (WE) from dried CS1197 mycelium was obtained following a method reported earlier.14 Soy lecithin (SL) was obtained from M s−1 Shakti soyas Ltd., TN, India, and comprised a natural mixture of phospholipids (phosphatidylcholine (PC), phosphatidylethanolamine (PE), phosphatidylinositol (PI) and phosphatidic acid (PA)), glycolipids and triglycerides. Cholesterol (Chol, 99% purity) and Tween 80 were purchased from Sigma Chemical (Bangalore, India). Other reagents of analytical grade were from Rankem (Bangalore, India) and Merck (Bangalore, India). Food grade CO2 (purity of 99.9%) was from local suppliers (Mysore, India).
Preparation of nano-sized liposomes via a SC-GAS method. The apparatus for and process of liposome production for the encapsulation of water soluble compounds from C. sinensis CS1197 (CS liposomes) based on GAS principles are detailed elsewhere.5 Briefly, the process involves two major steps: (a) the SCCO2 assisted drying of the liposomal contents; and (b) the hydration of lipids under pressure to form large unilamellar vesicles (LUVs) following nano-sized liposome production through depressurization. C. sinensis CS1197 WE was mixed with liposomal contents such as SL and Chol (3
:
1 w/w) in the extract
:
liposomal content ratio of 1
:
10 (w/w). The liposomal contents were dissolved using a solvent mixture of chloroform and ethanol (3
:
1 v/v) at a ratio of 1
:
3 (w/v) and loaded into a 500 ml high pressure reactor vessel (Berghof Autoclave, HPR, Germany). Initially, experiments were carried out in batch mode to optimize a favorable operating range for pressure, temperature and surfactant content. The experimental process was optimized via tuning the process parameters, such as pressure, temperature and Tween 80 concentration, between 50 and 200 bar, 40 and 65 °C, and 0 and 3%, respectively.
Preparation of CS liposomes via a thin-film method. Liposomes were also prepared via a conventional thin film process, known as the Bangham method.15 The liposomal contents were dissolved in a mixture of chloroform and ethanol (3
:
1, v/v) at a ratio of 1
:
20 (w/v). The organic solvents were removed under reduced pressure in a rotary evaporator at 65 °C (above the lipid-transition temperature). After 1 h of equilibration, a phosphate buffer solution (PBS) (pH 7.2)/Tween 80 (0.75%, w/v) solution was introduced under vacuum and hydrated for 2 h for better encapsulation.9
Characterization of CS liposomes
Mean particle diameter and coefficient of uniformity (Cu). The mean particle size was determined using a particle size analyzer (model: S3500, Microtrac Inc., USA). Each measurement was carried out in triplicate and the results are expressed as mean ± SD, where SD represents the mean error. Cu is used as a criterion in the particle size distribution of soil to provide the index of gradation. It is known that the higher the value of Cu, the larger the range of particle size and uniformity indicated, when Cu is between 1 and 3.16 In the present study, Cu is used as a criterion to define the disparity of liposome particles, where a value of less than 4 is defined as being uniformly distributed and above 4 as showing a broad distribution range. Cu is defined as the ratio of D60 to D10. The values for D60 and D10 were obtained from particle size distribution data.
Determination of the encapsulation efficiency (EE). A known amount of CS liposome suspension appropriately diluted was used to determine the EE. Liposomes of adenosine, cordycepin and polysaccharides were measured for their EEs. Adenosine and cordycepin content were measured via HPLC and the polysaccharide content was measured via a phenol sulfuric method detailed elsewhere.14 The diluted solution was subjected to centrifugation at 7500 rpm for 1 h at 4 °C. A free extract aliquot was then separated for analysis. The encapsulation efficiency was calculated using the equation: |
 | (1) |
where C1 is the initial content (adenosine, cordycepin and polysaccharides) in the C. sinensis CS1197 water extract used for encapsulation and C2 is the amount of free content present in the liposome suspension.
Morphology of CS liposomes
Phase contrast microscopy. The liposome samples were diluted with triple distilled water in order to obtain better images. About 3 μl of diluted liposome was placed on a glass slide with a micro-syringe and then covered with a cover slip. The slide was then subjected to phase contrast microscopy (Olympus BX-5, Japan) including a digital camera to capture images. All liposome samples were observed under 100× magnification.
Scanning electron microscopy (SEM). Scanning electron microscopy (model: LEO 435 VP; LEO Electron Microscopy Ltd., Cambridge, UK) was used to observe the topography and surface morphology of the liposomes. Samples were freeze dried first on SEM stubs and analyzed for liposome particle sizes and shapes.
Liposome stability index (LSI). The emulsifying activity of the surfactant and the interaction of the lipid mixture under supercritical CO2 for tailoring the nano-liposome particles were assessed via determining the LSI using a turbidimetric method. Freshly made liposomes were diluted appropriately with triple distilled water just before measuring the absorbance at 500 nm using a UV-visible spectrophotometer (UV-1800, Shimadzu, Japan) with 1 cm path length cuvettes. The liposomes were stored in an incubator for 24 h at a temperature of 37 °C, followed by measuring the absorbance at a wavelength of 500 nm.17 The turbidity and LSI of the liposomes were calculated using equations, as mentioned in an earlier report.5
Rheological examination. The viscosities of the CS liposomes were determined using a controlled stress rheometer (Rheostress 6000, Haake, Karlsruhe, Germany) at 25 ± 0.1 °C, using a coaxial cylinder attachment (spindle no. Z 41) by applying the shear rate in a linear manner from 0.1 up to 100 s−1 over a span of 60 s. All measurements were carried out in duplicate and the apparent viscosity of the liposomes was calculated at a shear rate of 100 s−1.18
Differential scanning calorimetric (DSC) analysis. DSC (PerkinElmer DSC 8000, Norwalk, CT, USA) was used to determine the phase transition behavior, such as the onset temperature (To), melting temperature (Tm), completion temperature (Tc), and enthalpy (ΔH, J g−1), of free C. sinensis, CS1197 WE, and empty and C. sinensis CS 1197 WE encapsulated nano-liposomes. A 5 mg sample was placed in an aluminum pan and DSC analysis was carried out at a nitrogen flow rate of 15 ml min−1 and a heating rate of 10 °C min−1 from 30 to 300 °C. An empty aluminum pan was placed on the reference platform.
Thermogravimetric analysis (TGA). TGA of samples was carried out using TGA Q50 apparatus (TA instruments, New Castle, DE USA). The experiments were performed at a heating rate of 10 °C min−1 under a nitrogen flow of 150 ml min−1. Dried C. sinensis CS1197 water extract and encapsulated nano-liposomes were weighed (5–10 mg) accurately in a hanging aluminum pan and the percentage weight losses of the samples were monitored from 30 to 600 °C.
X-ray diffraction (XRD) analysis. X-ray diffraction analysis of C. sinensis CS1197 WE and encapsulated CS liposomes was carried out to observe the purity and crystalline structures using a Reguka Miniflex diffractometer (1.5426 Å) with Cu Kα radiation, operating at 30 kV/15 mA. All measurements were performed at room temperature within the 2θ range of 0–80° and at a speed of 1° min−1.
Fourier transform infrared (FT-IR) analysis. The interactions between C. sinensis CS1197 water soluble compounds and the lipid membranes were evaluated via Fourier transform infrared spectroscopy (FT-IR). FT-IR spectra were recorded with KBr pellets on a FTIR−8400S spectrometer (Shimadzu, iR solution 1.30, Shimadzu Corporation, Japan) between 4000 and 400 cm−1.
Storage stability studies. CS nano-liposomes prepared via both the SC-GAS and Bangham methods were kept for observation for 2 months at different temperatures (4, 25, and 37 °C) and pH values (2, 4, 7, and 10). The samples kept at different temperatures and pH values were withdrawn and checked for stability at regular intervals via measuring the EE, size, viscosity and Cu of the CS nano-liposomes. Fresh samples were considered as controls.
Oxidative stability of nano-CS liposomes. Determinations of oxidative stability were based on the peroxide value (PV) as per the AOCS official method Cd 8-53. A liposome sample (0.1 g) was mixed with a solvent mixture of chloroform
:
methanol (7
:
3 v/v), followed by the addition of 50 μl of both 10 mM xylenol orange solution and ferric chloride solution. After incubation for 5 min in the dark, the absorbance of the mixture was taken at 560 nm using a UV/vis spectrophotometer (UV-1800, Shimadzu, Japan). The standard curve is plotted from a series of Fe(III) standard solutions. PVs were expressed as milli-equivalents (meq) O2/kg liposome:19 |
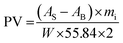 | (2) |
where AS is the absorbance of the sample, AB is the absorbance of the blank, mi is the inverse of the slope of the standard curve, and W is the weight of the sample (g).
In vitro release. To estimate the adenosine, cordycepin and polysaccharides release under in vitro conditions, the leakage of these compounds from the liposomal interior was monitored via a membrane dialysis method. A dialysis bag was filled with 3 ml of CS liposome suspension, immersed in 100 ml of PBS (pH 6.8), and incubated in a water bath at 37 °C with stirring at 100 rpm. At intervals of 0.25, 0.5, 1, 2, 4, 6, 8, 10, 12, 24, 36 and 48 h, 2 ml of the surrounding buffer was sampled and, at the same time, an equal volume of PBS was replenished. The concentration of adenosine, cordycepin and polysaccharides in the extract was determined as described above. The dialysis behavior of free C. sinensis CS1197 WE mixed with PBS was examined using the same method. The rate and release mechanism of C. sinensis CS1197 water soluble compounds from the prepared nano-liposomes was analyzed through fitting the in vitro release data using the following kinetic models: |
(1) ln C = ln C0 − K1t (first order model)
| (3) |
|
(2) Q = K2t1/2 (Higuchi model)
| (4) |
where C is the amount of drug released at time t; K1 is the first order release rate constant (%/h) and K2 is the diffusion rate constant.
Statistical analysis. Statistical analysis of the data was conducted using Microsoft® Excel 2010. Data were reported as mean ± standard deviation (SD). Analysis of variance (ANOVA) was conducted and significant differences between means were obtained via Tukey's multiple comparison tests at a significance level of p < 0.05.
Results and discussion
Preparation of nano-sized CS liposomes via the SC-GAS method
The phenomena involved in liposome formation using the SCCO2-gas anti-solvent technique have been illustrated earlier.5 The basic principle of the GAS method involves the saturation of lipid components with SCCO2, reducing solubility in organic solvents and resulting in precipitation. The lipid drying step was optimized at 15 min, during which lipid saturation with SCCO2 facilitates the rapid diffusion of CO2, with the attainment of an expanded lipid bilayer with the complete removal of remnant solvent. Hydration under pressure and equilibration for 1 h yields LUVs with a bimodal particle size distribution. The depressurization of the SC liquid to reduce the large unilamellar vesicles (LUVs) to small unilamellar vesicles (SUVs) on the nano-scale is an essential step in the GAS technique. A slow depressurization rate (25 bar min−1) offers better control over particle size and distribution, as observed in a previous study.5 Similar observations were made by other authors.20,21 The importance of the depressurization of the SC liposome suspension near the lipid transition temperature is discussed later.
Effects of pressure
The effects of pressure at a constant temperature (50 °C) and Tween 80 concentration (0.75%) on the mean diameter, EE, and Cu of liposomes prepared via the SC-GAS technique are shown in Fig. 1A. The pressure was varied between 50 and 200 bar and it was found that increasing the pressure has a significant (p < 0.05) positive effect on particle size, EE and Cu. The extent of free and diffused CO2 in the phospholipid bi-layer is the determining parameter for the particle size of liposomes. Fig. 1A indicates that a lower pressure of <80 bar is not enough to reduce the particle size to the nano-level (<100 nm) due to the ineffective diffusion of CO2 in the lipid bi-layer. Liposome formation at pressures of 50 and 80 bar cannot ensure the sphericity of particles due to the unequal diffusion of CO2 throughout the lipid layer and its ineffectiveness in the complete unpacking of the bi-layer structure to discrete lipid molecules. Low pressure treatment yields liposomes with a broad range particle size distribution (Fig. 2A). As the pressure was increased from 120 to 200 bar, there was a significant reduction in particle size and this pressure range is effective for producing spherical particles possessing a narrow particle size distribution range (Fig. 2B). Lisha Zhao and co-workers reported operating pressure range to obtain spherically shaped submicron liposomes.10,11 A higher pressure range facilitated the CO2/aqueous/Tween80/phospholipids quaternary system to become more favorable for uniform dispersion of phospholipid molecules, allowing them to unite together after depressurization to form a larger number of nano-sized liposomes per unit volume; thus a better EE can be achieved. Increasing the pressure from 50 to 200 bar drastically reduces the liposome size from 0.978 ± 0.003 to 0.099 ± 0.004 μm, with a greater number of liposomes per unit volume achieving a maximum EE of 75.48 ± 2.45, 74.78 ± 3.2 and 70.31 ± 2.9 for adenosine, cordycepin and polysaccharides, respectively.
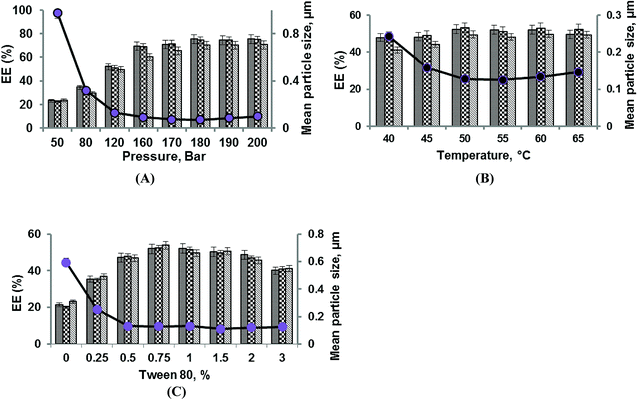 |
| Fig. 1 Effects of pressure (A), temperature (B) and Tween 80% concentration (C) on the mean particle size and the liposomal encapsulation efficiency of adenosine, cordycepin, and polysaccharides in CS1197 WE. : adenosine; : cordycepin; : polysaccharides; and : mean diameter. | |
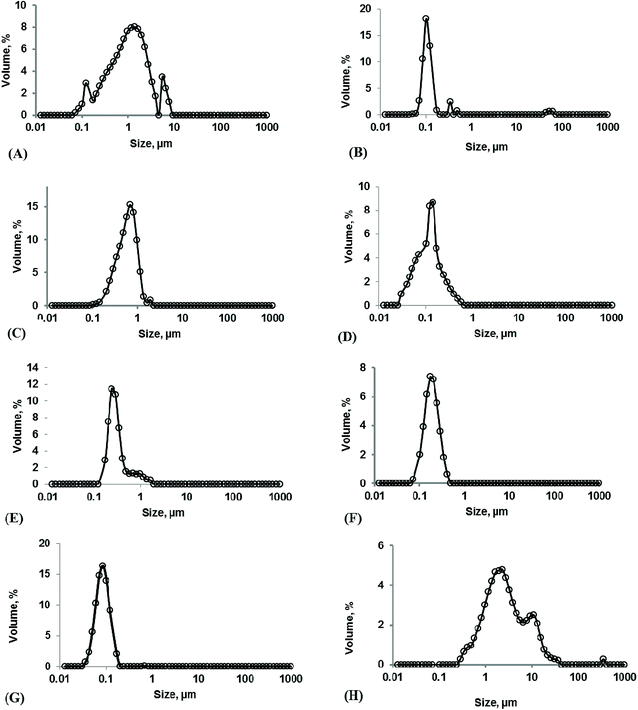 |
| Fig. 2 The size distribution of liposomes obtained: at pressures of 50 bar (A) and 200 bar (B); at Tween 80 concentrations of 0% (C) and 4% (D); at temperatures of 40 °C (E) and 65 °C (F); under optimized conditions of 180 bar, 50 °C and 0.75% Tween 80 (G); and via the Bangham method (H). | |
The current configuration of the nano-liposome production setup was proven to engineer unimodal nano-liposomes over the tested pressure range (50–200 bar), provided the pressure inside the pressure vessel was necessarily kept constant during depressurization. Throughout depressurization, CO2 was released uniformly from every portion of the liquid phase, leading to each particle being influenced by dense CO2 and leading to a low Cu. The increasing pressure reasonably improved the uniformity of the liposomes from 8.32 ± 0.013 to 1.19 ± 0.012 (Table S1†) over the tested pressure range. Further experiments were carried out at pressures between 160 and 200 bar to select the best effective pressure for nano-liposome production. The liposomes produced at a pressure of 180 bar were the smallest liposomes, with a mean particle size of 0.072 ± 0.003 μm (Fig. 2G) and Cu of 1.10 ± 0.012 (Table S1†), with better EE.
Effects of Tween 80 concentration
The presence of a surfactant (Tween 80) plays an important role in the CO2/aqueous/Tween 80/phospholipids quaternary system, wherein the concentration of Tween 80 majorly governs the extent of lipid dispersion into the aqueous phase, which helps liposome recovery during the depressurization step. The concentration of Tween 80 at a constant pressure (120 bar) and temperature (50 °C) was varied between 0 and 3% to evaluate its effect on the mean particle size, EE and Cu of liposomes (Fig. 1C). Even though one can obtain liposomes in the absence of a surfactant, the particle mean diameter is larger (0.593 ± 0.005 μm) with a broad particle size distribution (Fig. 2C) and poor EEs of 21.34 ± 3.1, 20.12 ± 1.9 and 23.2 ± 2.7 are achieved for adenosine, cordycepin and polysaccharides, respectively. This is due to the fact that no repulsive force exists around the liposomes after depressurization to stop coalescence, and moreover there is incomplete dispersion of lipids in the aqueous phase affecting the CO2/aqueous/surfactant/lipids quaternary homogenous phase, and about 50% (by wt) of the liposome material still remained in the pressure vessel, thus affecting the EE. Experiments were carried with small amounts of Tween 80 (0.25%) to produce liposomes, where the particle size was reduced to 0.252 ± 0.003 μm and Cu was 4.65 ± 0.012 (Table S1†). Increasing the concentration of Tween 80 from 0.25 to 0.75% shows a positive effect, from which a minimum particle size of 0.129 ± 0.002 μm (Fig. 1B), maximum EEs of 51.96 ± 1.98, 52.36 ± 2.6 and 53.78 ± 2.1 for adenosine, cordycepin and polysaccharides, respectively, and improved Cu of 3.14 ± 0.011 (Table S1†) were obtained for 0.75%. Liposome production using a Tween 80 concentration in the range of 0.25 to 2% resulted in an 80 to 90% (data not shown) liposome yield, and the rest remained in the vessel due to foam formation, which was unavoidable. A further increase in surfactant concentration from 1 to 3% resulted in a slight increase in the mean diameter (Fig. 2D). This may be due to continued foam formation causing negative effects.22
Effects of temperature
Variations in temperature at a constant pressure (120 bar) and Tween 80 concentration (1%) around the phase transition temperature (40–65 °C) involve melting transitions of lecithin, which is due to van der Waals interactions between adjacent lipid molecules. In the absence of CO2, lecithin exists in a lamellar gel phase at lower temperatures, whereas at higher temperatures it changes to a fluid liquid-crystalline phase. In the presence of dense CO2 and cholesterol, the gel-fluid phase transition temperature is reduced and the fluid liquid-crystalline phase region is broadened.23 In a high pressure system, whatever the temperature, the fluid liquid-crystalline phase is favoured, which is required for SC mediated liposome production as it enables liposomal encapsulation even at mild temperatures.24 In the present study, the effects of temperature at a constant pressure and surfactant content were evaluated with regards to the particle size, EE and Cu of liposomes (Fig. 1B). Temperature has a significant effect (p < 0.05) on the CO2/aqueous/Tween 80/lipids quaternary system, as an increase in temperature from 40 to 50 °C results in the reduction of the particle size from 0.243 ± 0.005 to 0.128 ± 0.002 μm (Fig. 2E). This is due to an increased density of CO2 under the interactive effects of pressure (120 bar) and temperature (45 and 50 °C), which creates the most favorable conditions for increased CO2 solubility in the aqueous phase and the formation of small particles. But at higher temperatures (55–65 °C) the particle size was increased to 0.147 ± 0.004 μm (Fig. 2F) and there was a slight decrease in the EE; this is due to the fact that elevated temperatures decrease the cohesive energy density of CO2, lowering its solubility in the aqueous phase and also causing the partitioning of the surfactant towards the aqueous phase, creating a slightly higher CO2-lecithin phase and a lower surfactant-aqueous phase.25 The unequal availability of surfactant results in the aggregation of liposomes during depressurization. An increase in temperature also results in the formation of a greater number of fine particles, thus increasing the EEs to 52.40 ± 1.68, 53.25 ± 2.9 and 49.57 ± 2.6 for adenosine, cordycepin and polysaccharides, respectively. Cu decreased from 3.18 ± 0.012 to 2.80 ± 0.015 as the temperature increased from 40 to 50 °C (Table S1†) and later increased with temperature to 2.98 ± 0.018, due to phase separation causing a non-uniform distribution of surfactant molecules around the liposomes. Hence, an optimal temperature of 50 °C is recommended for the production of nano-sized liposomes, where better size uniformity and EE can be obtained.
Importance of depressurization and depressurization at the phase inversion temperature to the particle size and EE
The importance of the depressurization step lies in the conversion of LUVs to SUVs via creating a pressure drop through micrometric high pressure tubing. Experiments were carried out under optimized conditions, i.e., 180 bar, 50 °C and 0.75% Tween 80, to understand the importance of depressurization on the particle size and EE. Liposome samples were collected from the pressure vessel just after the hydration step, and liposomes exhibited a large multimodal size distribution with a Cu value of 3.01 ± 0.021 with EEs of 59.23 ± 2.3, 57.13 ± 2.1 and 53.12 ± 1.9 for adenosine, cordycepin and polysaccharides, respectively. A unique feature of the current configuration of the SC-GAS process is that particle formation and encapsulation happen twice, i.e. during hydration under pressure and after depressurization, which is one possible way to obtain liposomes with superior characteristics exhibiting better EE.
It is well understood that the presence of dense CO2 in the CO2/water/surfactant/lipids quaternary system facilitates the phase transition of lecithin at a mild temperature. During the depressurization step, the release of CO2 causes cooling of the liquid phase, creating a sudden shock, which is un-favorable for the re-assembling of lipid molecules to form liposomes. Due to an increase in the phase transition temperature, phospholipids undergo a phase transition from a fluid liquid-crystalline phase to a lamellar gel phase. Fig. 3M clearly proves that depressurization of the SC liquid phase at ambient temperature causes the formation of fewer liposomes, and most of the lipid molecules remain discrete, exhibiting poor EEs of 47.34 ± 2.5, 45.98 ± 3.1 and 41.56 ± 2.1 for adenosine, cordycepin and polysaccharides, respectively. So it is recommended that depressurization to atmospheric pressure occurs at a certain temperature above the lipid phase transition to obtain the highest number of small uniform liposomes.
Characterization of CS nano-liposomes
Morphologies of the liposomes. Liposomes prepared via Bangham and SC-GAS methods were observed under a phase contrast microscope to characterize the morphologies and shapes of the liposomes. All samples were observed under 100× magnification to confirm the occurrence of nano-sized and spherical liposomes, as per the data obtained using the particle size analyzer. From Fig. 3A–E it can be seen that increasing the pressure at a constant temperature, Tween 80 concentration and depressurization rate (25 bar min−1) has a positive effect on particle size reduction and the sphericity of the liposomes. Fig. 3F–J also suggests that increasing the concentration of Tween 80 from 0 to 2% improved the particle dispersion in the aqueous phase, establishing a stabilized liposome system and inhibiting particle growth. The occurrence of large multilamellar liposomes with irregular shape from the Bangham method was evident in Fig. 3L.
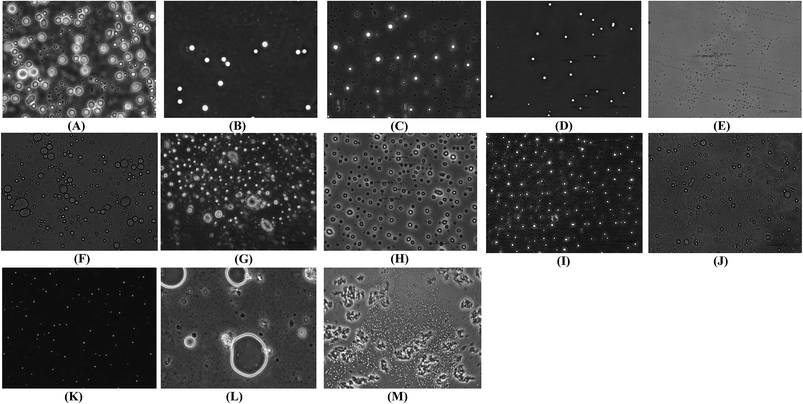 |
| Fig. 3 Phase contrast micrographs of CS1197 WE liposomes prepared using different formulation variables: (A–E) pressures ranging from 50–200 bar, 50 °C, 0.75% Tween 80; (F–J) Tween 80 concentrations of 0, 0.25, 0.5, 1, and 2%, 170 bar, 50 °C; (K) 180 bar, 50 °C, 0.75% Tween 80; (L) using the Bangham method; and (M) at 180 bar, 50 °C, 0.75% Tween 80, with depressurization at room temperature. All liposome samples were observed under 100× magnification. | |
The occurrence of very much smaller sized liposomes (<100 nm) from the SC-GAS method than from the conventional method is evident in SEM pictures of the liposomes, where their occurrence was abundant. Smaller sized liposomes are delivered more efficiently to an infected site compared with larger sized liposomes. The SEM images in Fig. 4A and B confirm the occurrence of particles, supporting the micrographs from phase contrast microscopy. By observing the results regarding liposome size (Fig. 2G), and phase contrast and SEM images (Fig. 3K and 4A, respectively), the proposed configuration for SC-GAS liposome production, at 180 bar and 50 °C with 0.75% Tween 80 and a depressurization rate of 25 bar min−1, can undoubtedly be employed to produce mono-dispersed nano-liposomes with reproducible features.
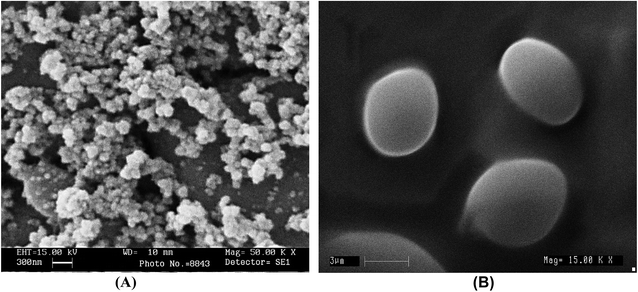 |
| Fig. 4 SEM photomicrographs of liposomes prepared via: the SCCO2-GAS method at 180 bar, 50 and 0.75% Tween 80 (A); and the Bangham method (B). | |
Liposome stability index (LSI). The liposome stability index (LSI) is a quick method to estimate the stability of liposomes. The process temperature, pressure and surfactant concentration had simultaneous effects on LSI. The liposomes were kept under stressed temperature conditions (37 ± 1 °C) to trigger the process of aggregation. If the stability index value is lower, this is a sign of lesser stability in the liposomal formulation. As the pressure increases from 50 to 200 bar, the LSI is found to increase from 179 to 479 (Fig. S1†), indicating that a pressure between 170 and 200 bar is a favorable range to produce stabilized narrow size distributed nano-liposomes. When the temperature increases, the LSI increases (210–476) up to 50 °C, but there was no significant difference when the temperature increased from 50 to 65 °C (Fig. S1†). The emulsifying properties of the liposome system were improved when the Tween 80 concentration increased from 0 to 3%, thus improving the LSI (129–698).
Viscosity of the liposomes. The viscosity of liposomes plays an important role during long term storage and is also an important parameter for stability and efficient drug release. Higher viscosity liposomes are an indication of smaller size and a narrow particle distribution. It is also known that higher viscosity nano-liposomes result in the slower release of active ingredients and have a lower clearance rate after administration. It was observed that all the liposome formulations showed near Newtonian behavior (Fig. S2†). The flow behavior of the liposomes was similar to milk, which is a good example of a stable O/W emulsion where lipid droplets are stabilized by milk proteins.18,26 Table S1† explains the rheological properties of liposomes prepared under different formulation conditions. In the SC-GAS method, viscosity has a significant effect on particle size reduction and so the apparent viscosity of the liposome suspensions was found to increase with increasing pressure between 50 and 160 bar, and it was further stabilized between 160 and 200 bar. As the temperature increased the viscosity was found to increase and later it followed a slight decreasing trend. But an increase in the Tween 80 concentration from 0 to 3% increased the apparent viscosity of the liposomes from 1.16 ± 0.01 to 1.92 ± 0.01 mPa s. At a higher Tween 80 concentration, a larger amount of hydrophilic tails was available to entrap water molecules in the formulation, which may lead to increased viscosity.27 The highest apparent viscosity of 1.79 ± 0.02 mPa s was obtained for nano-liposomes prepared at 180 bar and 50 °C, with 0.75% Tween 80.
Comparison with the Bangham method. The physical properties of liposomes, in terms of mean particle size, EE, and Cu, prepared via SC-GAS and thin-film methods were compared. The particle size distribution for liposomes prepared via the thin film method is shown in the Fig. 2H. Liposome vesicles prepared via the conventional method have a relatively broad size distribution ranging between 0.55 and 10.05 μm with a mean diameter of 5.64 μm, and moreover the vesicles exhibited irregular bilayer thickness (Fig. 3M & 4B), which may reduce the membrane stability and lead to considerable leakage. The Cu and viscosity values were 11.97 ± 0.019 and 1.198 ± 0.01, which implies that the conventionally prepared liposomes were characterized as being highly poly-dispersed. The conventionally prepared liposomes showed poor EEs of 38.34 ± 3.4, 37.57 ± 3.4 and 33.45 ± 3.3% for adenosine, cordycepin and polysaccharides, respectively. Another disadvantage of the conventional method is the poor reproducibility of its features.12 The particle sizes of liposomes formed via the SC-GAS method were markedly lower, exhibiting a thinner appearance (Fig. 5B) than those produced via a thin-film dispersion, which had higher heterogeneity and showed a very thick appearance (Fig. 5A). In comparison with the Bangham method, the SC-GAS process requires less energy, as discussed elsewhere,5 to yield nano-liposomes with a mean particle diameter of 0.072 ± 0.002 μm, Cu of 1.10 ± 0.012 and an improved EE 1.97 times that achieved using the Bangham method (Fig. 3K).
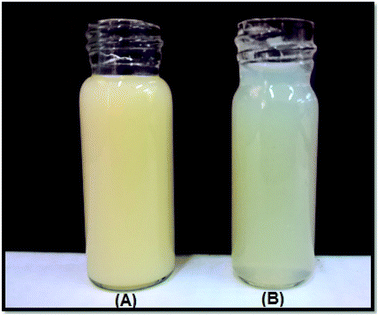 |
| Fig. 5 CS liposomes prepared via the Bangham method (A); and the SC-GAS technique (B). | |
The SC-GAS method in fed-batch mode is strongly supported as a superior method of nano-liposome production compared to other variants of SC techniques and high pressure based competitive methods that were discussed in an earlier report.5 The present configuration of the SC-GAS set-up facilitates nano-liposomes with a mean size of 72 nm and an EE of about 75%, which is 40% less than other SC methods and 70–90% less than high pressure homogenization and micro-fluidizer based techniques. This is the first study to report the production of stabilized superior liposomes that are less than 100 nm with a narrow range size distribution for the encapsulation of water soluble compounds from C. sinensis CS1197 via a SC-GAS method.
Thermal analysis of the nano-liposomes. The DSC method is a tool used to measure the temperature and energy variation involved in phase transitions, i.e., the gel to liquid-crystalline phase transitions of phospholipid bilayers, which reflects the degree of crystallinity of free C. sinensis CS1197 WE and extract entrapped in liposomes. DSC thermograms for free C. sinensis CS1197 and CS liposomes are shown in Fig. 6A. Empty liposomes (liposomes without extract) showed a melting endotherm at 137.94 °C, CS nano-liposomes showed this at 139.50 °C, and C. sinensis CS1197 WE does not show any melting endotherm. The melting endotherms are the result of crystallinity, and no peak was detected for free C. sinensis CS1197 WE; this may be due to the lack of crystalline forms and/or higher purity and an amorphous nature. The results were comparable to an earlier report.9 A liquid crystalline (LC) nature is very important for drug delivery, and LC based systems can offer the specific advantages of thermodynamic stability, high solubilization levels, improved bioavailability, protection against oxidation and controlled release properties to pharmaceuticals.28 In the present study, crystallinity was attributed to free C. sinensis CS1197 WE due to liposomal encapsulation, which helps to enhance the performance of the formulation for drug delivery.
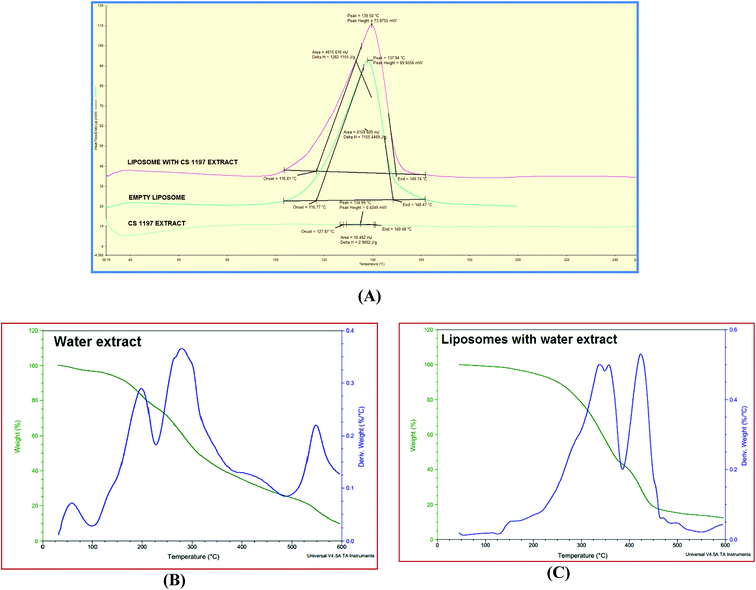 |
| Fig. 6 DSC and TGA thermograms. DSC thermograms of CS1197 WE, empty liposomes (To −116.77 °C, Tm −137.94 °C, Tc −148.47 °C, and ΔH −1155 J g−1) and CS nano-liposomes (To −116.81 °C, Tm −139.50 °C, Tc −149.74 °C and ΔH −1282 J g−1) (A); and TGA thermograms of CS1197 WE (B) and CS nano-liposomes (C). | |
TGA was used to measure physical and chemical changes in compounds as a function of temperature. TGA curves for C. sinensis CS1197 WE and CS nano-liposomes are shown in Fig. 6B and C, respectively. During heating from 30 to 600 °C, about 90% wt loss was observed at a temperature of 198 °C for free C. sinensis CS1197 WE and 290 °C for the CS nano-liposomes. This wt loss may be due to a loss of moisture and/or a change in the molecular structure causing phase transitions (as shown by the secondary Y-axis). Liposomal encapsulation has improved the thermal stability of the extract.
FTIR analysis. FTIR spectra of C. sinensis CS1197 WE, empty liposomes and CS nano-liposomes were studied in order to understand the association between C. sinensis CS1197 water soluble compounds and phospholipids (Fig. 7). The FTIR spectrum of free C. sinensis CS1197 WE contains principle bands at 3402, 2931, 2360, 1627, 1404, 1080, and 1033 (Fig. 7A), and that of empty liposomes contains bands at 3410, 2924, 2854, 1735, 1651, 1465 and 1095 (Fig. 7B). The FTIR spectrum of CS liposomes (Fig. 7C) contains similar bands to those contained in both free extract and empty liposome spectra, indicating that the liposomal encapsulation of water soluble compounds did not result in the formation of new linkages, thus ensuring efficient drug unloading at the target sites.
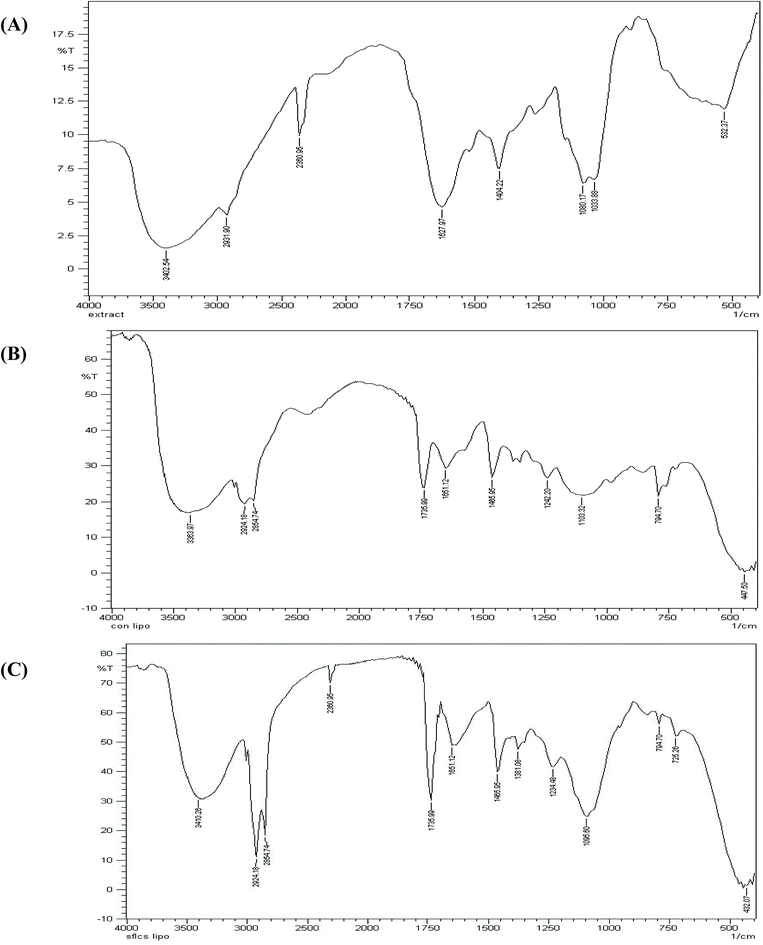 |
| Fig. 7 FTIR spectra of: CS1197 water extract (A); empty liposomes (B); and CS liposomes (C) prepared via the SCCO2-GAS technique. | |
XRD analysis. XRD has been widely used in the characterization of nanoparticles for detailing critical features, such as crystal structure, crystallite size, and strain. The broadening of diffraction peaks is due to disordered states of crystals. This has been attributed to the absence of total constructive and destructive interferences of X-rays in a finite sized lattice.29 The XRD pattern for CS nano-liposomes was recorded over a 2θ range of 0 to 80°, as shown in Fig. 8B. Sharp characteristic peaks appeared at 2θ = 28° and 41°, exhibiting a polymorphic nature. These diffraction peaks are due to an ordered lipid bi-layer, which is a superior characteristic of nano-liposomes produced via the SC-GAS method. However, no peak or broadened diffraction peak at 2θ = 21° was seen in the case of free C. sinensis CS1197 WE (Fig. 8A) which is due to its amorphous nature/lack of purity, or due to it being composed of highly disordered crystals. So, due to liposomal encapsulation, a highly ordered crystalline nature was attributed to C. sinensis CS1197 WE, which is important for enhanced performance in drug delivery.
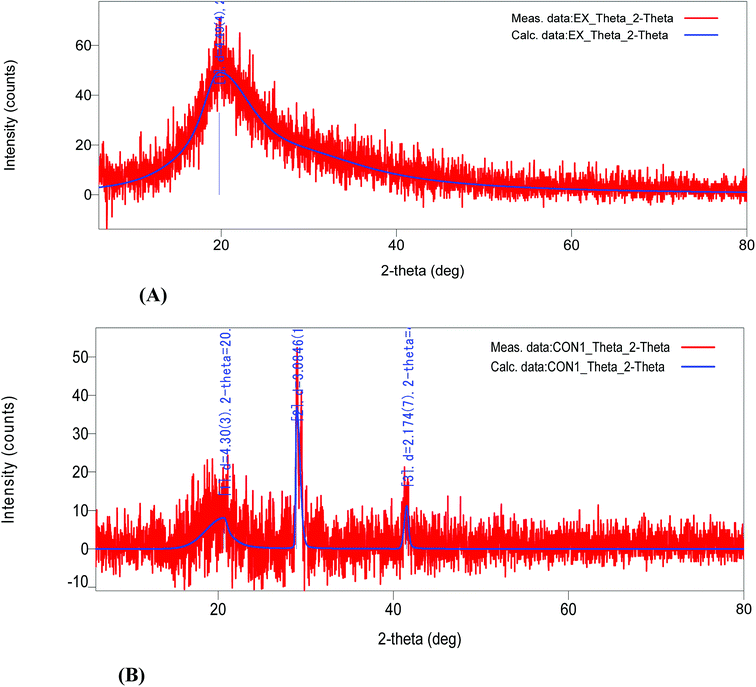 |
| Fig. 8 X-ray diffraction spectra of: CS1197 WE (A); and CS nano-liposomes (B). | |
Storage studies. Storage stability is an important index of any drug delivery system. Physical instability can lead to drug leakage and the aggregation or fusion of vesicles.30 Liposomes prepared via the SC-GAS method were assessed after different time intervals of storage at 4, 25 and 37 °C and pH values of 2, 4, 7 and 10 (Table 1).
Table 1 The effects of storage temperature and pH on the particle size, coefficient of uniformity and viscosity of CS nano-liposomes
Time (weeks) |
Mean particle size (μm) |
Coefficient of uniformity (Cu) |
Viscosity (mPa s) |
Temperature effect |
4 °C |
25 °C |
37 °C |
4 °C |
25 °C |
37 °C |
4 °C |
25 °C |
37 °C |
0 |
0.072 ± 0.002 |
|
|
1.101 ± 0.010 |
|
|
1.781 ± 0.01 |
|
|
1 |
0.072 ± 0.001 |
0.073 ± 0.001 |
0.072 ± 0.003 |
1.102 ± 0.081 |
1.111 ± 0.013 |
1.110 ± 0.013 |
1.779 ± 0.02 |
1.782 ± 0.02 |
1.778 ± 0.01 |
2 |
0.073 ± 0.001 |
0.073 ± 0.003 |
0.074 ± 0.002 |
1.120 ± 0.013 |
1.119 ± 0.019 |
1.121 ± 0.018 |
1.778 ± 0.01 |
1.779 ± 0.01 |
1.777 ± 0.02 |
3 |
0.073 ± 0.001 |
0.074 ± 0.001 |
0.075 ± 0.001 |
1.126 ± 0.012 |
1.125 ± 0.018 |
1.126 ± 0.018 |
1.779 ± 0.02 |
1.777 ± 0.02 |
1.777 ± 0.02 |
4 |
0.075 ± 0.003 |
0.076 ± 0.001 |
0.079 ± 0.002 |
1.125 ± 0.112 |
1.126 ± 0.021 |
1.130 ± 0.013 |
1.776 ± 0.01 |
1.775 ± 0.03 |
1.759 ± 0.03 |
6 |
0.076 ± 0.001 |
0.077 ± 0.003 |
0.082 ± 0.004 |
1.130 ± 0.011 |
1.131 ± 0.011 |
1.139 ± 0.019 |
1.759 ± 0.01 |
1.750 ± 0.04 |
1.750 ± 0.01 |
8 |
0.076 ± 0.001 |
0.077 ± 0.005 |
0.089 ± 0.003 |
1.202 ± 0.018 |
1.139 ± 0.026 |
1.149 ± 0.010 |
1.758 ± 0.02 |
1.749 ± 0.02 |
1.733 ± 0.01 |
Time (weeks) |
pH effect |
2 |
4 |
7 |
10 |
2 |
4 |
7 |
10 |
2 |
4 |
7 |
10 |
0 |
0.072 ± 0.002 |
|
|
|
|
|
|
|
|
|
|
|
1 |
0.072 ± 0.002 |
0.072 ± 0.002 |
0.072 ± 0.004 |
0.073 ± 0.003 |
1.110 ± 0.013 |
1.109 ± 0.013 |
1.111 ± 0.013 |
1.120 ± 0.013 |
1.779 ± 0.02 |
1.781 ± 0.02 |
1.782 ± 0.02 |
1.780 ± 0.02 |
2 |
0.078 ± 0.003 |
0.076 ± 0.001 |
0.073 ± 0.003 |
0.077 ± 0.002 |
1.131 ± 0.019 |
1.121 ± 0.019 |
1.119 ± 0.019 |
1.124 ± 0.019 |
1.763 ± 0.01 |
1.773 ± 0.01 |
1.779 ± 0.01 |
1.779 ± 0.01 |
3 |
0.081 ± 0.002 |
0.078 ± 0.004 |
0.074 ± 0.001 |
0.082 ± 0.001 |
1.170 ± 0.018 |
1.130 ± 0.018 |
1.125 ± 0.018 |
1.155 ± 0.018 |
1.729 ± 0.02 |
1.769 ± 0.02 |
1.777 ± 0.02 |
1.759 ± 0.02 |
4 |
0.080 ± 0.001 |
0.077 ± 0.003 |
0.076 ± 0.002 |
0.087 ± 0.004 |
1.174 ± 0.021 |
1.154 ± 0.021 |
1.126 ± 0.021 |
1.163 ± 0.021 |
1.665 ± 0.03 |
1.765 ± 0.03 |
1.775 ± 0.03 |
1.565 ± 0.03 |
6 |
0.090 ± 0.004 |
0.080 ± 0.002 |
0.077 ± 0.003 |
0.108 ± 0.004 |
1.271 ± 0.011 |
1.201 ± 0.011 |
1.131 ± 0.011 |
1.298 ± 0.011 |
1.557 ± 0.04 |
1.757 ± 0.04 |
1.750 ± 0.04 |
1.367 ± 0.04 |
8 |
0.108 ± 0.002 |
0.087 ± 0.003 |
0.077 ± 0.005 |
0.127 ± 0.005 |
1.340 ± 0.026 |
1.289 ± 0.026 |
1.139 ± 0.026 |
1.450 ± 0.026 |
1.510 ± 0.02 |
1.740 ± 0.02 |
1.749 ± 0.02 |
1.240 ± 0.02 |
The nano-liposomes prepared via the SC-GAS method stored at 4, 25 and 37 °C were highly stable for up to 2 months (Table 1) with no significant changes (p > 0.05) in mean size, Cu and viscosity. This is due to the fact that uniformly distributed nano-liposomes possess a uniform charge density around the surface, attaining a thermodynamically stabilized state. Liposomes prepared via the SC-GAS and Bangham methods and stored at different temperatures were observed under a phase contrast microscope (Fig. 9). Liposomes prepared via the SC-GAS method showed no aggregation, but liposomes prepared via the Bangham method showed fast aggregation after 2 months. Large variations in the mean size caused an unequal distribution of the charge density i.e., large particles possessed a larger charge density compared to smaller particle, and as a result larger particles had the tendency to show dominance over smaller particles because the large potential difference caused aggregation. Fig. 10A–C shows the effects of storage temperature on the EE over two months. The EEs of liposomes for adenosine, cordycepin and polysaccharides decreased by less than 2.3% at 4 °C, 4.4% at 25 °C and 9.8% at 37 °C after one month, and less than 3.2% at 4 °C, 7.3% at 25 °C and 20.3% at 37 °C after two months. The study suggests that liposome storage at 4 and 25 °C favors minimal compound leakage, assuring 92–97% drug retention in the nano-liposomes, whereas 79–81% drug retention can be assured for liposomes stored at 37 °C (Table 2).
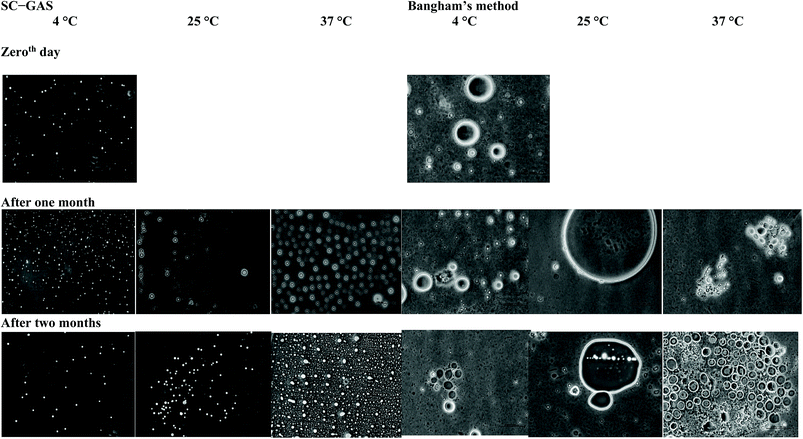 |
| Fig. 9 Phase contrast micrographs of liposomes prepared via the SC-GAS and Bangham methods, stored at different temperatures. | |
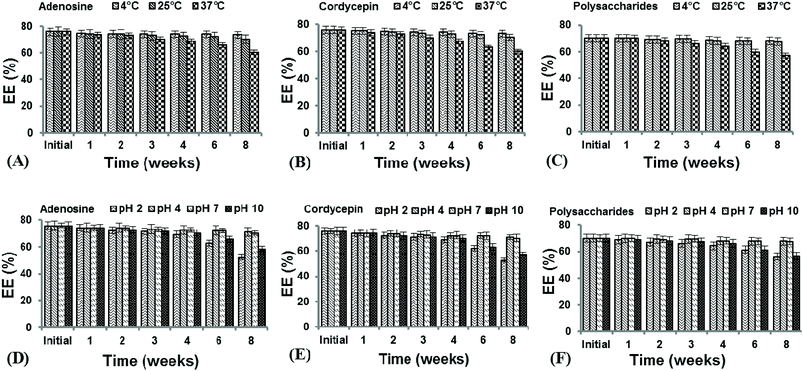 |
| Fig. 10 The effects of storage time, temperature and pH on the encapsulation efficiencies of CS nano-liposomes. | |
Table 2 The effects of storage temperature and pH on the percentage retention of water soluble compounds from CS1197 in the nano-liposomes
Parameter |
|
Retention after 8 weeks (%) |
Adenosine |
Cordycepin |
Polysaccharides |
Temperature (°C) |
4 |
97.07 |
96.26 |
96.79 |
25 |
92.65 |
92.83 |
96.51 |
37 |
79.6 |
78.82 |
81.17 |
pH |
2 |
68.79 |
69.42 |
79.9 |
4 |
93.64 |
93.44 |
96.66 |
7 |
92.65 |
92.83 |
96.51 |
10 |
76.96 |
75.07 |
80.69 |
The CS nano-liposomes stored at different pH values of 2, 4, 7 and 10 were assessed for their EEs (Fig. 10D–F), mean diameters, Cu values and viscosities over two months (Table 1). The nano-liposomes stored at pH 4 and 7 were stable for up to 2 months with no significant changes (p > 0.05) in mean size, Cu and viscosity. This is due to the fact that a pH value of 4 to 7 favored the maximum retention of surfactant molecules on the surfaces of the liposomes, thus arresting aggregation. However, nano-liposomes stored under extreme pH conditions, i.e., 2 and 10, were stable until the 3rd week and were later destabilized due to fast aggregation. Under extreme pH conditions, higher ionic strength caused a lowering of the surfactant charge density over the liposome surfaces, resulting in fast aggregation. This study suggests that liposome storage at pH 4 and 7 favors minimal compound leakage, assuring 92–96% drug retention in nano-liposomes, whereas 68–80% drug retention can be assured for liposomes stored at pH 2 and 10 (Table 2).
Oxidative stability of the CS nano-liposomes. Nano-liposomes prepared via the SC-GAS method were evaluated for their oxidative stability at different temperatures and pH values over 8 weeks (Fig. 11). Liposomes stored at 4 and 25 °C showed increasing PVs for the first 4 weeks and were later found to stabilize. The maximum PVs for liposomes at 4 and 25 °C were found to be 1.15 and 1.53 meq O2/kg of sample after 8 weeks. However, liposomes stored at 37 °C exhibited the highest PV, i.e., 4.88 meq O2/kg of sample. It can be concluded that liposome storage at 4 and 25 °C can ensure minimum lipid oxidation. In addition, the oxidative status of nano-liposomes at different pH values was evaluated over 8 weeks (Fig. 11). The liposomes stored at pH 4 and 7 showed minimal PVs, i.e., 0.32, 1.30, and 1.58 meq O2 kg of sample. Storage at pH 2 showed the minimum PV, but the storage of liposomes at pH 2 cannot be recommended because of the increased particle size and decreased EE at a low pH value of 2. Liposome storage at a higher pH value of 10 resulted in accelerated lipid oxidation, and the highest PV was 2.02 meq O2/kg of sample. So from the results, it can be concluded that extreme pH conditions are not recommended, and pH values of 4 and 7 favor minimal lipid oxidation.
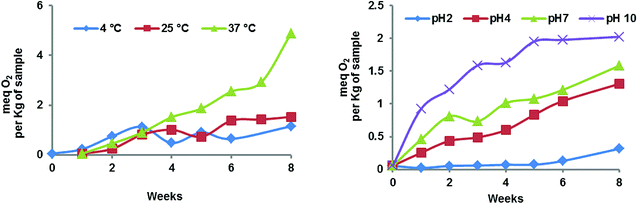 |
| Fig. 11 Peroxide values (PVs) for CS nano-liposomes observed for 8 weeks under accelerated conditions of temperature and pH. | |
In vitro release studies. In vitro drug release studies are important to help understand the in vivo performance of the dosage form. Drug release studies help in the evaluation of sustained and prolonged release dispersion systems.31 In the present study, in vitro release studies of adenosine, cordycepin and polysaccharides were carried over 48 h through a dialysis membrane. Fig. 12 shows that C. sinensis CS1197 WE in its free form resulted in a fast release profile, i.e., release values of about 81, 83, and 51% for adenosine, cordycepin, and polysaccharides, respectively, were observed over 4 h. But in the case of the nano-liposomes, release values of about 40, 46 and 27% for adenosine, cordycepin and polysaccharides, respectively (Fig. 12) were observed over 4 h. It is suggested that the drug release from the liposomes characteristically exhibited a bi-phasic fashion, i.e., an initial fast drug loss followed by diffusion through the membrane.32 The initial fast rate of release is commonly due to compound detachment from the liposomal surface, while the later slow release results from sustained drug release from the inner lamellae. C. sinensis CS1197 WE is mainly entrapped inside the bilayer lipid structure of the liposomes and the in vitro release studies showed that compound transport out of the liposomes was driven mainly by a diffusion-controlled mechanism. Since prolonged delivery through nano-liposomes prepared via the SC-GAS method undoubtedly reflects a longer residence time in circulation, this could help bio-active compounds to reach target sites.
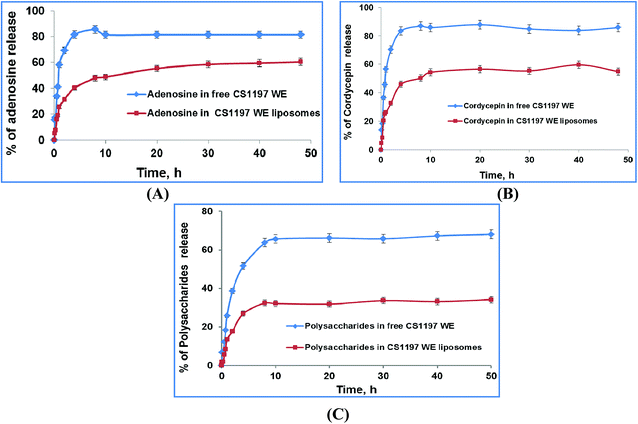 |
| Fig. 12 The in vitro kinetic release profiles of CS nano-liposome dispersions prepared via the SC-GAS method in pH 6.8 hydration medium at 37 °C. | |
The mechanism of the release phenomenon through the nano-liposomes was studied via applying two kinetic models. The kinetic data for the two models is shown in Table 3 and the regression of the kinetic models to the in vitro kinetic release data from the CS nano-liposome formulations is shown in Fig. S3.† A first order model fits the in vitro experimental release data of free C. sinensis CS1197 WE well (R2 = 0.927–0.943), where a fast release pattern is adopted. But the release of C. sinensis CS1197 WE through the nano-liposomes followed the Higuchi model (R2 = 0.931–0.957), proving that release through the liposomes involves a diffusion-controlled mechanism.
Table 3 The parameters of the kinetic models for in vitro release by the CS nano-liposome formulations
|
|
First order |
Higuchi model |
K1 |
R2 |
K2 |
R2 |
Free CS1197 WE |
Adenosine |
0.1794 |
0.927 |
26.622 |
0.833 |
Cordycepin |
0.191 |
0.943 |
27.594 |
0.847 |
Polysaccharides |
0.017 |
0.929 |
22.512 |
0.969 |
CS liposomes |
Adenosine |
0.027 |
0.849 |
17.877 |
0.953 |
Cordycepin |
0.031 |
0.844 |
17.274 |
0.931 |
Polysaccharides |
0.047 |
0.869 |
11.593 |
0.957 |
Conclusions
The SC-GAS method provides a simpler way to prepare liposomes with a characteristic nano-size and better morphology (i.e., a uniform size and shape). The current configuration of the SC-GAS mediated liposome production unit can be operated in fed-batch mode, with the additional feature of ensuring reproducibility for the encapsulation of both hydrophilic and hydrophobic active compounds. CS nano-liposome production under optimized conditions, i.e., 180 bar, 50 °C and 0.75% Tween 80, offers stabilized liposomes exhibiting good LSI and rheological properties and achieving about 75% EE. DSC and TGA studies reveal that encapsulation within a lipid bilayer improves the thermal stability of C. sinensis water soluble compounds. FTIR analysis proved that the liposomal encapsulation of C. sinensis WE ensures the efficient unloading of active ingredients at target sites. The ordered structure of the lipid bi-layer was attributed to crystallinity, which was confirmed via XRD analysis. Storage and oxidative stability studies suggest that maintaining the CS nano-liposomes at 4 and 25 °C ensures maximum drug retention and minimum lipid oxidation. In vitro kinetic release studies show that the active compounds are delivered through liposomes via a diffusion controlled mechanism. The proposed model can be successfully implemented on a pilot scale, indicating that supercritical processes can provide promising technical and economic conditions for large scale production. Supercritical technology shows advantages over conventional preparation methods and offers great potential for the encapsulation of valuable compounds relevant to nutraceutical, functional food and pharmaceutical applications.
Conflicts of interest
The authors report no conflicts of interest. The authors alone are responsible for the content and writing of this paper.
Acknowledgements
The author (SMG) acknowledges encouragement from the Director of the CSIR – Central Food Technological Research Institute, Mysore, India.
References
- P. LI, H. JI, T. Tina and W. Karl, Chin. J. Pharm. Anal., 2001, 21, 77–78 Search PubMed.
- M. Shashidhar, P. Giridhar, K. U. Sankar and B. Manohar, J. Funct. Foods, 2013, 5, 1013–1030 CrossRef CAS.
- G. Gregoriadis, N. Engl. J. Med., 1976, 295, 704–710 CrossRef CAS PubMed.
- S.-L. Huang, Adv. Drug Delivery Rev., 2008, 60, 1167–1176 CrossRef CAS PubMed.
- G. Shashidhar and P. Gadkari, RSC Adv., 2016, 6, 57739–57750 RSC.
- S. Varona, Á. Martín and M. a. J. Cocero, Ind. Eng. Chem. Res., 2011, 50, 2088–2097 CrossRef CAS.
- A. S. Zarena and K. U. Sankar, Ther. Delivery, 2011, 2, 259–277 CrossRef CAS.
- U. S. Kadimi, D. R. Balasubramanian, U. R. Ganni, M. Balaraman and V. Govindarajulu, Nanomedicine, 2007, 3, 273–280 CrossRef CAS PubMed.
- S. M. Ghatnur, R. S. Sonale, M. Balaraman and U. S. Kadimi, J. Liposome Res., 2012, 22, 215–223 CrossRef CAS PubMed.
- L. Zhao and F. Temelli, J. Supercrit. Fluids, 2015, 100, 110–120 CrossRef CAS.
- L. Zhao and F. Temelli, J. Food Eng., 2015, 158, 104–112 CrossRef CAS.
- L. Zhao, F. Temelli, J. M. Curtis and L. Chen, Food Res. Int., 2015, 77, 63–72 CrossRef CAS.
- I. E. Santo, R. Campardelli, E. C. Albuquerque, S. V. de Melo, G. Della Porta and E. Reverchon, Chem. Eng. J., 2014, 249, 153–159 CrossRef CAS.
- S. M. Ghatnur, G. Parvatam and M. Balaraman, Pharmacogn. Mag., 2015, 11, 448 CrossRef CAS PubMed.
- A. Bangham, M. M. Standish and J. Watkins, J. Mol. Biol., 1965, 13, 238–IN227 CrossRef CAS PubMed.
- R. F. Craig, Craig's soil mechanics, CRC Press, 2004 Search PubMed.
- K. N. Pearce and J. E. Kinsella, J. Agric. Food Chem., 1978, 26, 716–723 CrossRef CAS.
- P. V. Gadkari and M. Balaraman, J. Food Eng., 2015, 147, 14–23 CrossRef CAS.
- R. Wrolstad, T. Acree, E. Decker, M. Penner, D. Reid, S. Schwartz, C. Shoemaker, D. Smith and P. Sporns, Lipids, and Carbohydrates. Wiley, New Jersey, 2005 Search PubMed.
- T. P. Castor and L. Chu, US Pat., US5776486A, 1998.
- T. Castor, WO9427581, 1994.
- R. Campardelli and E. Reverchon, J. Food Eng., 2015, 149, 131–136 CrossRef CAS.
- G. D. Bothun, B. L. Knutson, H. J. Strobel and S. E. Nokes, Langmuir, 2005, 21, 530–536 CrossRef CAS PubMed.
- L. Lesoin, O. Boutin, C. Crampon and E. Badens, Colloids Surf., A, 2011, 377, 1–14 CrossRef CAS.
- C. T. Lee, P. A. Psathas, K. P. Johnston, J. deGrazia and T. W. Randolph, Langmuir, 1999, 15, 6781–6791 CrossRef CAS.
- A. Zarena, S. Bhattacharya and U. S. Kadimi, Food Bioprocess Technol., 2012, 5, 3007–3013 CrossRef.
- J. Rao and D. J. McClements, J. Agric. Food Chem., 2011, 59, 5026–5035 CrossRef CAS PubMed.
- P. P. Gaikwad and T. Desai Maya, Int. J. Pharma Res. Rev., 2013, 2, 40–52 Search PubMed.
- B. Akbari, M. P. Tavandashti and M. Zandrahimi, Iran. J. Mater. Sci. Eng., 2011, 8, 48–56 CAS.
- W. Zhou, W. Liu, L. Zou, W. Liu, C. Liu, R. Liang and J. Chen, Colloids Surf., B, 2014, 117, 330–337 CrossRef CAS PubMed.
- V. Venkateswarlu and K. Manjunath, J. Controlled Release, 2004, 95, 627–638 CrossRef CAS PubMed.
- P. Panwar, B. Pandey, P. Lakhera and K. Singh, Int. J. Nanomed., 2010, 5, 101–108 CAS.
Footnote |
† Electronic supplementary information (ESI) available. See DOI: 10.1039/c8ra07601d |
|
This journal is © The Royal Society of Chemistry 2018 |
Click here to see how this site uses Cookies. View our privacy policy here.