DOI:
10.1039/C8RA07160H
(Paper)
RSC Adv., 2018,
8, 34116-34120
Synthesis and conductive performance of polyoxometalate acid salt gel electrolytes
Received
28th August 2018
, Accepted 13th September 2018
First published on 4th October 2018
Abstract
Two vanadium-substituted polyoxometalate acid salt gel electrolytes, [PyPS]3H4SiW9V3O40 and [PyPS]5H2SiW9V3O40, have been synthesized using a 1-(3-sulfonic group) propylpyridine (PyPS) and a Keggin vanadium-substituted heteropoly acid H7SiW9V3O40 through an ionic self-assembly method, and adjusting the ratio of cation and anion. A substitution effect of the acid salt gel electrolytes has been investigated. Interestingly, when protons of the polyoxometalate acid salt gel electrolytes are substituted, both the conductivity and the phase transformation temperature increase. The fastest conductivity of these gel electrolytes was as high as 2.57 × 10−2 S cm−1 at 110 °C.
1. Introduction
Heteropoly acids (HPAs) or polyoxometalates (POMs) are a type of metal oxide cluster. It is formed by inorganic metal oxygen cluster anions that means a variety of structures and characteristics can be obtained.1,2 Therefore, polyoxometalate-based compounds show interesting electronic, magnetic, redox, medicinal and photonic properties.3–7 In particular, detailed research into conduction in these compounds will inspire the development of fuel cells and electrochemical electrolytes based on POMs.8–12 However, the machinability of POMs is poor, and the conductivity is highly related to the amount of water molecules in the POM structure. Thus, the conductivity will be decreased at high temperature.13
Ionic liquids (ILs) are a type of excellent molten salt since it remains in the liquid phase at room temperature. The preparation of the ionic liquids based on relatively large organic cations and inorganic anions through ionic self-assembly. Ionic liquids have many excellent properties in range of physicochemical, such as thermal stability and electrochemical applications.14–16 Many kinds of novel ionic liquids have been synthesized by different cations and anions with ionic self-assembly effect.17,18
Recently, many researcher found that the combination of the physical properties of POMs with those of IL salts generated a “chimera”, which exhibits a liquid-like property.19 These materials show a quasi-solid state at room temperature, and it is changed to a liquid state when being heated to about 100 °C.20 Consequently, the quasi-solid state materials exhibit higher ionic conductivity than that of other solid conductors and have advantages over both a liquid electrolyte and a solid state electrolyte, which makes this new class of materials potential candidates for electrolyte in advanced electrochemical devices.21–25 However, many researcher focus on the normal salts of the polyoxometalates ionic liquids (POM-ILs) and there are few articles reported about the acid salts (POM-ASs) of POM-ILs.
Generally, Keggin-type heteropoly acids have many special characteristics such as stable structure, simple preparation method and high yield. Thus, we chose a Keggin-type vanadium-substituted HPA (H7SiW9V3O40) and the pyridine class with sulfonic group functional compound, 1-(3-sulfonic group) propylpyridine (PyPS) to synthesize a series of POM-based ionic liquids through the ionic self-assembly method. The phase transformation, structure and conductive performance of two POM-ASs compounds were characterized.
2. Experimental section
2.1 Instrument and reagent
Elemental analysis was determined by inductively coupled plasma (ICP-MS) analysis on a Shimadzu V-1012 ICP-MS spectrometer. Infrared (IR) spectrum was conducted on a NICOLET NEXUS 470 FT/IR spectrometer during the wavenumber range 400–4000 cm−1 using KBr pellet. X-ray powder diffraction (XRD) pattern was conducted on a BRUKER D8 ADVANCE X-ray diffractometer using a Cu tube at the conditions: at 40 kV and 40 mA in the range of 2θ = 4–40° at a rate of 0.02°·s−1. The thermal stability of these samples was reported on a SHIMADZU thermal analyzer. Conductivity was measured through a DDS-11A conductivity meter using a Shanghai DJS-1 and DJS-10 electrode. The UV absorption spectra were monitored by a Specord TU-1901 UV-vis spectrophotometer.
All the chemicals were of analytical grade and used without further purification.
2.2. Synthesis of the POM-ASs
1-(3-Sulfonic group) propylpyridine (PyPS) was synthesized according to the literature.23 H7SiW9V3O40 was synthesized as follow, Na10[α-SiW9O34] was synthesized by a method according to recent literature. A 10 mL aqueous solution of sodium metavanadate (1.71 g NaVO3) was added to a 30 mL aqueous solution of Na10[α-SiW9O34] (10 g Na10[α-SiW9O34]). The mixture was adjusted to pH = 1.5. And then, the mixture was added into the H+ type cation exchange resin column at room temperature, until the pH of the mixture is less than 1. The mixture was dried at 40 °C and a orange power was obtained.
The pre-synthesized PyPS and H7SiW9V3O40 were taken in 3
:
1 and 5
:
1 mole ratios to give one mole of [PyPS]3H4SiW9V3O40 and [PyPS]5H2SiW9V3O40. PyPS was added into an aqueous solution of H7SiW9V3O40. The mixture was reacted under ultrasound for 10 minutes at room temperature, and then collected bottom layer liquid. The liquid evaporated at room temperature and then the product as oily gel-type was obtained.
3. Results and discussion
The detail IR spectra of the compounds at 1100–700 cm−1 is shown in the Fig. 1 and Table 1. As shown in the Fig. 1 and Table 1, we can clearly find that these feature frequencise, all compounds, fall in the stretching sequence of νas(Si–Oa), νas(M–Od), νas(M–Ob–M) and νas(M–Oc–M), (M = W, V), which is referred to POM character bands at 1100–700 cm−1. We also find the shift of characteristic peaks of POM-ASs in the Fig. 1 and Table 1 when compared with POM. This phenomenon can be explained by the vibrations frequency change result in the influence of the anion–anion interactions among polyoxoanions.26,27 In spite of the shift of characteristic peaks of POM-ASs, the peaks still exists, which can find at a red zone in the Fig. 1. The result infer that these compounds still maintain POM structure without decomposition, when PyPS added.
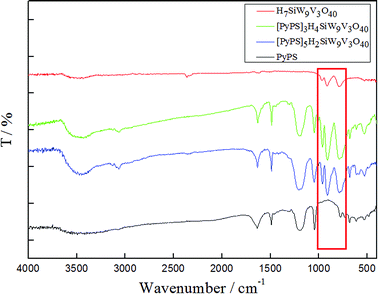 |
| Fig. 1 IR spectra of the compounds. | |
Table 1 The detail IR spectra of compounds at 1100–700 cm−1
Vibrations/cm−1 |
SiW9V3 |
[PyPS]3H4SiW9V3 |
[PyPS]5H2SiW9V3 |
M–Od stretching |
970 |
960 |
962 |
Si–Oa stretching |
914 |
911 |
908 |
M–Oc–M stretching |
785 |
790 |
788 |
The POM in non-reduced state is generally characterized by charge transfer bands of oxygen-to-metal, which can be observed by UV-vis spectrophotometer in the UV region below 400 nm. The UV absorption spectrum of the compounds is shown in the Fig. 2. As shown in the Fig. 2, we can find that the absorption bands of H7SiW9V3O40, [PyPS]3H4SiW9V3O40 and [PyPS]5H2SiW9V3O40 appear at about 260 nm, 259 nm, 260 nm, respectively. The absorption spectrum of POM-ILs display a moderately intense peak nearly 259 nm (O–M) and the peak is blue-shifted from 260 nm which is referred to the pure POM.28 This phenomenon can provide an evidence for intermolecular interactions between PyPS cations and Keggin-type anion units.
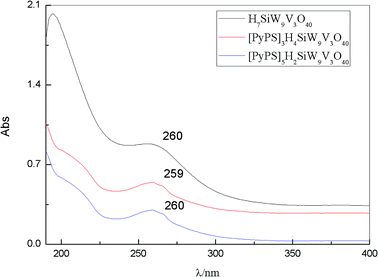 |
| Fig. 2 The UV absorption spectra of the HPA and POM-ASs. | |
Wide-angle XRD patterns of POM and POM-ASs are shown in the Fig. 4. The intensity is very strong peaks in the large region of the XRD patterns of H7SiW9V3O40. The Keggin-type of the polyoxometalates which exhibited typical peaks in the range of 2θ = 7–11° in the XRD patterns was found. From that phenomenon, it is clear that the POM compound was successfully prepared. Furthermore, we also found a broad diffraction peaks in the region of 2θ = 20–35° of POM-ASs, which indicates the distinction between the POM-ASs and their parent HPA.29 [PyPS]3H4SiW9V3O40 and [PyPS]5H2SiW9V3O40 present similar XRD pattern. This phenomenon infers that the parent HPA is changes into amorphous structure after the acidic protons (PyPS) were added.
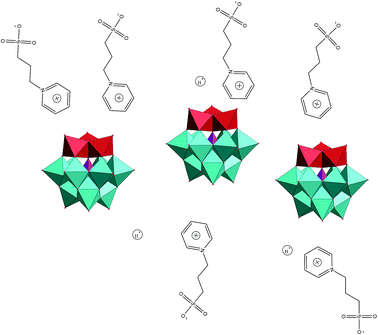 |
| Fig. 3 Schematic illustration of potential weak connections in these compounds among PyPS, protons and POM anion. | |
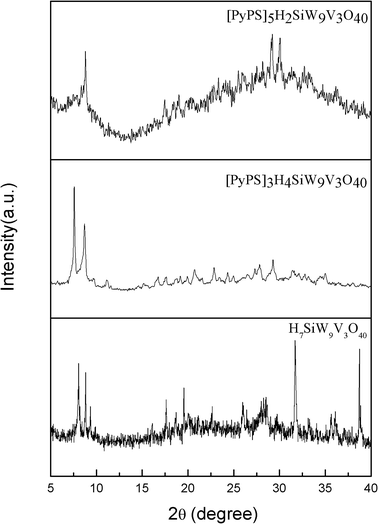 |
| Fig. 4 Wide-angle XRD patterns of the HPA and POM-ASs. | |
These POM-ASs exhibit a characteristic of reversible gel–liquid phase transformation. Fig. 5 shows the POM-ASs phase transform between gel state and liquid state at 100 °C. Since the weak connection among PyPS cation, POM anion and protons, the gel state of POM-ASs exhibit amorphous state, which can be proved by XRD patterns, and the schematic is shown in Fig. 3.
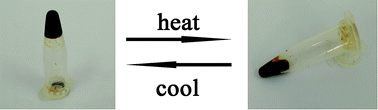 |
| Fig. 5 Photographs of the [PyPS]3H4SiW9V3O40 reversible gel–liquid phase transformation at room temperature and 100 °C. | |
The variation of the conductivity of these compounds can be observed with heating. The results of variation of conductivity show in the Fig. 6. As shown in the Fig. 6, it can find that there is a broad endothermic peak at about 85 °C for [PyPS]3H4SiW9V3O40 and at about 70 °C for [PyPS]5H2SiW9V3O40. That phenomenon can be attributed to a phase transformation from a solid gel phase to an isotropic liquid phase.30 It can also find that the conductivity of these compounds increase with heating, especially at the time that these compounds at the temperature of phase transformation from gel to liquid, the phenomenon of increasing conductivity is obvious. Concretely, at 110 °C the conductivity of [PyPS]3H4SiW9V3O40 and [PyPS]5H2SiW9V3O40 is 2.57 × 10−2 S cm−1 and 2.18 × 10−2 S cm−1, respectively. The phenomenon can be resulted in an increase in the migration of particles in the compound.
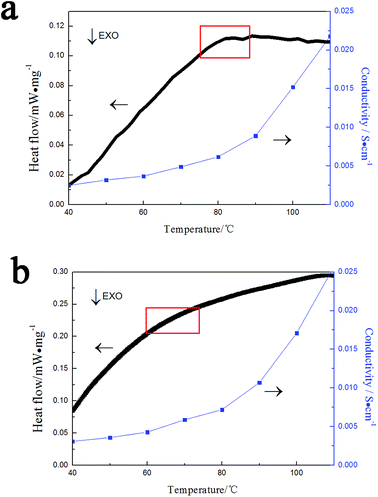 |
| Fig. 6 Conductivity–temperature and heat flow curves of (a) [PyPS]3H4SiW9V3O40 and (b) [PyPS]5H2SiW9V3O40. | |
Fig. 7 shows conductivity of different amount of PyPS substitution at different temperature. Obviously, when the number of protons in the compound increase, the conductivity of these compounds enhance accordingly. This phenomenon can be explained as that the protons in the compounds migrate much faster than PyPS. Thus it is found that the higher conductivity can result from more protons in the compound. Additionally, when many protons have substituted the PyPS, the conductivity of the compounds also increase. So it is infer that more protons in the compounds will result in higher conductivity. Furthermore, this result also can be confirmed by conductive activation energy.31,32
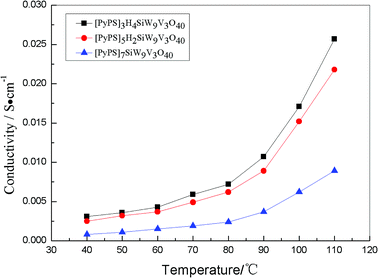 |
| Fig. 7 Conductivity–temperature plots of [PyPS]3H4SiW9V3O40, [PyPS]5H2SiW9V3O40 and [PyPS]7SiW9V3O40. | |
The conductive activation energy Ea can be evaluated as follow
where
σ is conductive,
σ0 is the pre-exponential factor,
Ea is the conductive activation energy,
R is the gas constant and
T is the absolute temperature.
Fig. 8 shows the conductive Arrhenius plots of [PyPS]
3H
4SiW
9V
3O
40, [PyPS]
5H
2SiW
9V
3O
40 and [PyPS]
7SiW
9V
3O
40 at the same condition. The values of
Ea of these compounds can be obtained by linear fit and the values of
Ea for [PyPS]
3H
4SiW
9V
3O
40, [PyPS]
5H
2SiW
9V
3O
40 and [PyPS]
7SiW
9V
3O
40 is 33.4 kJ mol
−1, 33.7 kJ mol
−1 and 35.7 kJ mol
−1, respectively. In this case, it is found that number of protons have an obvious influence on conductivity of the compounds.
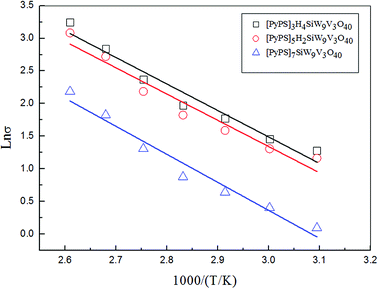 |
| Fig. 8 The conductive Arrhenius plots of [PyPS]3H4SiW9V3O40, [PyPS]5H2SiW9V3O40 and [PyPS]7SiW9V3O40 at the same condition. | |
4. Conclusions
In this paper, we have reported two vanadium-substituted polyoxometalate acid salts gel electrolytes, [PyPS]3H4SiW9V3O40 and [PyPS]5H2SiW9V3O40. These electrolytes exhibit reversible gel–liquid phase transformation. A relationship among the conductivity, substituted protons and temperature can be observed by heat flow and conductivity plots. The results indicate that the conductivity and phase transformation temperature improve with increasing the number of protons in the compounds. Thus these materials maybe provide both liquid state electrolytes and solid state electrolytes for supercapacitors.
Conflicts of interest
There are no conflicts to declare.
Acknowledgements
This work was supported by the Liaoning Provincial Natural Science Foundation of China (201602404), the Zhejiang Provincial Natural Science Foundation of China (LY18B010001) and the Scientific Research Foundation of Liaoning Institute of Science and Technology (RXYJ2015001).
References
- M. Shiddiq, D. Komijani, D. Yan, A. Gaitaariño, E. Coronado and S. Hill, Nature, 2016, 531, 348–351 CrossRef CAS PubMed.
- Z. Chen, W. B. Bu, D. L. Ni, C. J. Zuo, C. Chao, Q. Li, L. L. Zhang, W. Zheng and J. L. Shi, J. Am. Chem. Soc., 2016, 138, 8156–8164 CrossRef PubMed.
- A. Rubinstein, P. Jiménezlozanao, J. J. Carbó, J. M. Poblet and R. Neumann, J. Am. Chem. Soc., 2014, 136, 10941–10948 CrossRef CAS PubMed.
- T. Yoshida, T. Murayama, N. Sakaguchi, M. Okumura, T. Ishida and M. Haruta, Angew. Chem., Int. Ed., 2018, 57, 1523–1527 CrossRef CAS PubMed.
- J. J. Chen, M. D. Symes, S. C. Fan, M. S. Zheng, H. N. Miras, Q. F. Dong and L. Cronin, Adv. Mater., 2017, 27, 4649–4654 CrossRef PubMed.
- Y. W. Liu, S. M. Liu, X. Y. Lai, J. Miao, D. F. He, N. Li, F. Luo, Z. Shi and S. X. Liu, Adv. Funct. Mater., 2015, 25, 4480–4485 CrossRef CAS.
- X. Tong, N. Q. Tian, W. Wu, W. M Zhu, Q. Y. Wu, F. H. Cao, W. F. Yan and A. B. Yaroslavtsev, J. Phys. Chem. C, 2013, 117, 3258–3263 CrossRef CAS.
- J. J. Chen, J. C. Ye, X. G. Zhang, M. D. Symes, S. C. Fan, D. L. Long, M. S. Zheng, D. Y. Wu, L. Cronin and Q. F. Dong, Adv. Energy Mater., 2018, 8, 1701021 CrossRef.
- M. H. Yang, B. G. Choi, S. C. Jung, Y. K. Han, Y. S. Huh and S. B. Lee, Adv. Funct. Mater., 2015, 24, 7301–7309 CrossRef.
- H. Gao, A. Virya and K. Lian, J. Mater. Chem. A, 2015, 3, 21511–21517 RSC.
- M. Tountas, Y. Topal, A. Verykios, A. Soultati, A. Kaltzoglou, T. A. Papadopoulos, F. Auras, K. Seintis, M. Fakis and L. C. Palilis, J. Mater. Chem. C, 2018, 6, 1459–1469 RSC.
- X. F. Wu, W. Wu, Q. Y. Wu and W. F. Yan, Langmuir, 2017, 33, 4242–4249 CrossRef CAS PubMed.
- W. B. Kim, T. Voitl, G. J. Rodriguezrivera and J. A. Dumesic, Science, 2004, 305, 1280–1283 CrossRef CAS PubMed.
- W. L. Zhou, J. Peng, Z. Y. Zhang, Z. Y. Shi, S. U. Khan and H. S. Liu, RSC Adv., 2015, 5, 35753–35759 RSC.
- E. Rafiee, S. Eavani, X. Cai, X. F. Wu, R. S. Anaredy and S. K. Shaw, RSC Adv., 2016, 6, 46433–46466 RSC.
- R. Hayes, G. G. Warr and R. Atkin, Chem. Rev., 2015, 115, 6357–6426 CrossRef CAS PubMed.
- E. Elfassy, Y. Mastai, D. Pontoni and M. Deutsch, Langmuir, 2016, 32, 3164–3173 CrossRef CAS PubMed.
- Q. Y. Wu, X. Tong and X. F. Wu, J. Xuzhou Inst. Technol., Nat. Sci. Ed., 2011, 26, 1–8 Search PubMed.
- J. Azizullah, M. Al-Rashida, A. Haider, U. Kortz, S. A. Joshi and J. Iqbal, ChemistrySelect, 2018, 3, 1472–1479 CrossRef.
- A. B. Bourlinos, K. Raman, R. Herrera, Q. Zhang, L. A. Archer and E. P. Giannelis, J. Am. Chem. Soc., 2004, 126, 15358–15359 CrossRef CAS PubMed.
- S. Akbani, M. T. Hamed Mosavian, F. Moosavi and A. Ahmadpour, RSC Adv., 2017, 7, 44537–44546 RSC.
- A. R. Neale, C. Schütter, P. Wilde, P. Goodrich, C. Hardacre, S. Passerini, A. Balducci and J. Jacquemin, J. Chem. Eng. Data, 2017, 62, 376–390 CrossRef CAS.
- Y. Leng, J. Wang, D. R. Zhu, X. Q. Ren, H. Q. Ge and L. Shen, Angew. Chem., Int. Ed., 2010, 48, 168–171 CrossRef PubMed.
- X. F. Wu, X. H. Zhou, Q. Y. Wu and W. F. Yan, New J. Chem., 2016, 40, 7923–7927 RSC.
- Y. Y. Li, X. F. Wu, Q. Y. Wu, H. Ding and W. F. Yan, Dalton Trans., 2014, 43, 13591–13595 RSC.
- Z. K. Zhao, Y. T. Dai, T. Bao, R. Z. Li and G. R. Wang, J. Catal., 2012, 288, 44–53 CrossRef CAS.
- T. P Huang, N. Q. Tian, Q. Y. Wu and W. F. Yan, Soft Matter, 2015, 11, 4481–4486 RSC.
- X. F. Wu, X. Tong, Q. Y. Wu, H. Ding and W. F. Yan, J. Mater. Chem. A, 2014, 2, 5780–5784 RSC.
- Z. R. Xie, Q. Y. Wu and L. M. Ai, Funct. Mater. Lett., 2018, 11, 1850059 CrossRef CAS.
- T. L. Greaves and C. J. Drummond, Chem. Rev., 2008, 108, 206–237 CrossRef CAS PubMed.
- M. A. Hickner, H. Ghassemi, S. K. Yu, B. R. Einsla and J. E. Mcgrath, Chem. Rev., 2004, 104, 4587–4611 CrossRef CAS PubMed.
- Z. R. Xie, H. Wu, Q. Y. Wu and L. M. Ai, RSC Adv., 2018, 8, 13984–13988 RSC.
|
This journal is © The Royal Society of Chemistry 2018 |
Click here to see how this site uses Cookies. View our privacy policy here.