DOI:
10.1039/C8RA06505E
(Paper)
RSC Adv., 2018,
8, 35474-35484
Quantification and cytoprotection by vanillin, 4-methylguaiacol and 4-ethylguaiacol against AAPH-induced abnormal oxidative stress in HepG2 cells†
Received
2nd August 2018
, Accepted 30th September 2018
First published on 16th October 2018
Abstract
Vanillin, 4-methylguaiacol, and 4-ethylguaiacol, three phenolic compounds in Gujinggong (GJG) Chinese baijiu (Chinese liquor), were quantified by liquid–liquid extraction (LLE) combined with gas chromatography-mass spectrometry (GC-MS) and evaluated for their possible cytoprotective effects by AAPH-induced HepG2 cell model. To confirm whether vanillin, 4-methylguaiacol, and 4-ethylguaiacol protected HepG2 cells against AAPH-induced abnormal oxidative stress via motivating the Keap1–Nrf2 pathway, the gene and protein expression of Nrf2, Keap1, SOD, CAT, and GPx from the Keap1–Nrf2 pathway were measured with real-time PCR and western blot. Three levels of treatment doses (1000, 500, and 100 mg L−1) were applied. Results showed that vanillin, 4-methylguaiacol, and 4-ethylguaiacol exhibited potent cytoprotective effect in a dose-dependent manner, greatly alleviating or reversing the increased oxidative stress induced by AAPH through up-regulating the mRNA and protein expression levels of Nrf2, SOD, CAT, and GPx, and thereby, significantly improving the intracellular antioxidant defense system in HepG2 cells (p < 0.05). Based on these findings, it was confirmed that vanillin, 4-methylguaiacol, and 4-ethylguaiacol, natural components of Chinese baijiu, were able to modulate the expression of Nrf2 and its downstream antioxidative enzymes (i.e., SOD, CAT, and GPx) against AAPH-induced abnormal oxidative stress. Further, this study lays the foundation for better illustrating the health benefits of Chinese baijiu.
Introduction
Recently, many chronic illnesses, including cancer, heart disease, neurodegenerative diseases, diabetes, obesity, cardiovascular diseases and aging,1–3 have been related to oxidative stress. The critical role of reactive oxygen species (ROS) has also been noted.2 Excessive ROS can cause intracellular damage to DNA, proteins, or lipids, and such damage is detectable even in healthy young animals, suggesting that the intrinsic antioxidant system is not sufficient to completely eliminate ROS.4,5 Thus, in consideration of health and safety, finding bioactive components from daily foods and beverages that enhance intracellular antioxidant defense system is thought to be important for protecting cells against oxidative stress stimulated by excessive free radicals, such as ROS.
Phenolic compounds have been widely recognized as health-promoting components mainly due to their ability to alleviate oxidative stress, and are natural components of many foods and beverages.6–15 Particularly, many researches have demonstrated that phenolic compounds in alcoholic beverages possessed the capacity to scavenge free radical in vitro chemical assay (i.e., DPPH, ABTS, ORAC, and FRAP).10–13,16 According to recent studies, phenolic compounds have been widely detected in different types of Chinese baijiu and confirmed as important aroma compounds.17,18 Specifically, vanillin (VA), 4-methylguaiacol (4-MG), and 4-ethylguaiacol (4-EG) (chemical structures shown in Fig. 1) were observed to have significant contributions to the aroma of Gujinggong (GJG) Chinese baijiu (a brand of Luzhou-aroma or officially the strong-aroma type of Chinese baijiu).17 Additionally, the potent ability of VA, 4-MG, and 4-EG to scavenge free radicals has been verified by DPPH, ABTS, ORAC, and reducing power assays.19 Recently, research has confirmed that phenolic compounds attenuated oxidative stress not only by acting as an antioxidant itself, but also by improving intracellular antioxidative activity, such as glutathione peroxidase (GPx), catalase (CAT) and superoxide dismutase (SOD).5 In our previous study, the above conclusions were also observed.19 VA, 4-MG, and 4-EG were able to protect HepG2 cell against AAPH-induced oxidative stress via inhibiting the formation of ROS, malondialdehyde (MDA) and oxidized glutathione (GSSG), enhancing the activities of CAT, SOD and GPx, and inducing the formation of reduced glutathione (GSH).19 However, the exact antioxidant mechanism of VA, 4-MG, and 4-EG is still unknown.
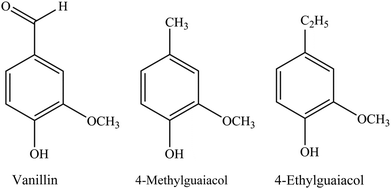 |
| Fig. 1 Chemical structures of vanillin, 4-methylguaiacol, and 4-ethylguaiacol. | |
Further, the hypothesis that phenolic compounds could activate the Kelch-like ECH-associated protein 1 (Keap1) – nuclear factor erythroid 2-related factor 2 (Nrf2) pathway and thereby induce the expression of intracellular antioxidant enzyme against ROS has been verified.20–23 Under normal conditions, Nrf2, the most critical transcription factor in the antioxidant defense system, is constantly degraded in a Keap1-dependent manner via the ubiquitin–proteasome pathway.24–26 However, oxidative stress can cause the dissociation of Nrf2/Keap1 complex, upon which Nrf2 migrates to the nucleus, binds to antioxidant response elements (AREs), and finally, activates cytoprotective genes and accelerates the expression of phase II detoxifying/antioxidant enzymes, such as SOD, CAT, and GPx, which contribute to the protection of cells against oxidative stress.27–30
Despite the overwhelming evidence suggesting the cytoprotection of phenolic compounds,31,32 information is still limited on the cytoprotective effect of vanillin (VA), 4-methylguaiacol (4-MG), and 4-ethylguaiacol (4-EG), phenolic compounds in Chinese baijiu, against oxidative stress in cells via activation of the Keap1–Nrf2 pathway. Therefore, in this study, VA, 4-MG, and 4-EG were studied to determine their potentials of ameliorating the oxidative damage through activation of the Keap1–Nrf2 pathway in 2,2′-azobis(2-methylpropionamide)-dihydrochloride (AAPH)-induced HepG2 cells, a useful model for evaluating the cytoprotective effect of natural antioxidants.31,33,34 Specifically, the effects of VA, 4-MG, and 4-EG on AAPH-induced changes in the expression of Nrf2, Keap1, SOD, CAT, and GPx were monitored. Moreover, to investigate whether VA, 4-MG, and 4-EG widely existed in GJG Chinese baijiu and to determine their concentrations in GJG Chinese baijiu, quantification by liquid–liquid extraction (LLE) combined with gas chromatography-mass spectrometry (GC-MS) was conducted.
Materials and methods
Chinese baijiu samples
Eight Gujinggong (GJG) Chinese Baijiu (CB) samples (labelled as GJGCB-1, 45% vol. of alcohol; GJGCB-2, 45% vol. of alcohol; GJGCB-3, 45% vol. of alcohol; GJGCB-4, 45% vol. of alcohol; GJGCB-5, 45% vol. of alcohol; GJGCB-6, 50% vol. of alcohol; GJGCB-7, 42% vol. of alcohol; GJGCB-8, 65% vol. of alcohol) were provided by Anhui Gujing Distillery Co., Ltd. (Anhui province, P. R. China). All samples were made from sorghum combined with wheat, corn, rice and sticky rice, and were stored at 4 °C until analysis. Here, the mention of brand name is only for research purposes, rather than for advertising.
Materials and reagents
2,2′-Azobis(2-methylpropionamide)-dihydrochloride (AAPH) was obtained from Acros (Geel, Belgium). Absolute ethanol, dichloromethane, and sodium chloride (NaCl) were purchased from Sinopharm Chemical Reagent Co., Ltd. (Beijing, China). Vanillin, 4-methylguaiacol, and 4-ethylguaiacol (Food Grade) were obtained from Ruiyuan Spice Co., Ltd. (Zaozhuang, China). 6-Hydroxy-2,5,7,8-tetramethylchroman-2-carboxylic acid (Trolox) was obtained from Sigma-Aldrich (St. Louis, USA). Other chemicals and reagents were indicated in specific methods.
Quantification of vanillin, 4-methylguaiacol, and 4-ethylguaiacol
Concentrations of vanillin, 4-methylguaiacol, and 4-ethylguaiacol in GJG samples were determined by an Agilent 7890B gas chromatograph, equipped with an Agilent 5977A mass-selective detector (Agilent Technologies, USA) as per previous method17 with a slight modification. Every GJG sample (2 mL) was diluted to 15% ethanol by volume with boiled Milli-Q water, saturated with NaCl, and extracted 3 times with freshly distilled dichloromethane (3 mL each time) by Vortex 2 (IKA, Germany). After centrifugation at 6000 rpm for 10 min, the combined extract was separated and concentrated to 500 μL, and immediately tested by gas chromatography-mass spectrometry (GC-MS) analysis.
Each concentrated extract (1 μL) was injected in splitless mode and analyzed on a DB-FFAP column (60 m × 0.25 mm i.d., 0.25 μm film thickness, J&W Scientific, USA). Helium was used as the carrier gas at a constant flow rate of 1 mL min−1. The injector temperature was set as at 250 °C. The temperature program of the oven was as follows: oven temperature was held at 40 °C at first, then raised to 50 °C at a rate of 10 °C min−1 and held for 20 min, then raised at 1 °C min−1 up to 70 °C and held for 10 min, finally raised at 3 °C min−1 up to 250 °C and held for 15 min.
MS was operated in electron ionization (EI) mode at 70 eV. Temperatures of the interface and the ion source were set at 250 and 230 °C, respectively. Identification of vanillin, 4-methylguaiacol, and 4-ethylguaiacol was conducted in a full scan mode with the mass range set from 45 to 350 amu. Selective ion monitoring (SIM) was used to quantify the analytes, and selected ions were listed in Table 1.
Table 1 Concentrations (μg L−1) of vanillin, 4-methylguaiacol, and 4-ethylguaiacol in GJG
Compound |
Vanillin |
4-Methylguaiacol |
4-Ethylguaiacol |
Totalb (μg L−1) |
The concentrations of compounds were presented as mean value of triplicate samples ± standard deviation (mean ± SD). The total concentrations of vanillin, 4-methylguaiacol, and 4-ethylguaiacol in each GJG sample. |
Quantified ion |
151 |
138 |
91 |
|
R2 |
0.997 |
0.997 |
0.999 |
|
LOD (μg L−1) |
10.9 |
3.1 |
4.7 |
|
Recovery (%) |
102 |
94 |
98 |
|
Concentrationa (μg L−1) |
GJGCB-1 |
209 ± 3 |
1062 ± 5 |
1405 ± 31 |
2675 ± 36 |
GJGCB-2 |
284 ± 7 |
1405 ± 17 |
1506 ± 16 |
3196 ± 34 |
GJGCB-3 |
214 ± 6 |
1092 ± 10 |
1397 ± 12 |
2703 ± 26 |
GJGCB-4 |
209 ± 3 |
705 ± 7 |
1708 ± 9 |
2622 ± 8 |
GJGCB-5 |
214 ± 4 |
694 ± 5 |
905 ± 16 |
1813 ± 14 |
GJGCB-6 |
120 ± 6 |
126 ± 4 |
129 ± 4 |
375 ± 12 |
GJGCB-7 |
103 ± 4 |
174 ± 5 |
149 ± 8 |
427 ± 8 |
GJGCB-8 |
156 ± 3 |
1580 ± 20 |
1013 ± 12 |
2749 ± 31 |
For standards, stock solutions of vanillin, 4-methylguaiacol, and 4-ethylguaiacol were prepared in absolute ethanol. Then, a series of working standard solutions (i.e., 10
000, 5000, 2000, 1000, 500, 200, 100, 50, 20, 10, and 1 μg L−1) were prepared from the stock solution with model solution (i.e., 45% ethanol by volume with Milli-Q water). Afterwards, each working standard solution (2 mL) was pretreated and analysed using the same extraction method and GC-MS analysis conditions as for GJG sample.
Calibration curves were drawn by plotting the response of target compounds against corresponding concentrations. Concentrations of target compounds were calculated based on the calibration curves. Analytical limits of detection (LOD) of target compounds were obtained from lowest concentrations of the analyte standard solutions based on a signal-to-noise ratio of 3. All analyses were repeated in triplicate.
Cell culture
Human hepatic cell line (HepG2) was provided by National Institute for Communicable Disease Control and Prevention, Chinese Center for Disease Control and Prevention (Beijing, China). Cells were grown and maintained in a humidified incubator at 37 °C and 5% CO2 using Dulbecco's Modified Eagle's Medium (Mediatech, Manassas, VA, USA) supplemented with 10% (v/v) fetal bovine serum, which was obtained from Gibco (Carlsbad, CA, USA). All studies were performed using 80% confluent cells before treatment.
CCK-8 assay
HepG2 cells (5 × 105 mL−1) were grown on a 96-well microplate. After treatment with various contents of sample (vanillin, 4-methylguaiacol, and 4-ethylguaiacol), cell viability was monitored by cell counting kit (CCK-8) assay (Dojindo, Kumamoto, Japan). After designed treatments, 10 μL of CCK-8 solution was added to the cells cultured in 96-well plates and OD values at 450 nm were measured with a spectrophotometer (Bio-Tek Instruments, Winooski, VT, USA). Cell viability was represented as a percentage of the absorbance observed for untreated control cells.
Sample treatment
HepG2 cells (1 × 106/well) were seeded into a 6-well microplate. After incubation for 24 h at 37 °C (5% CO2), the growth medium was removed and the wells were washed two times with PBS (Gibco, Carlsbad, CA, USA). Then, the cells were incubated with specific concentrations of vanillin, 4-methylguaiacol, and 4-ethylguaiacol (1000, 500, and 100 mg L−1) in 2 mL serum-free medium for 24 h at 37 °C (5% CO2). Afterwards, the medium was removed and the wells were washed two times with PBS. Then, 2 mL serum-free medium containing 200 μM AAPH was added, and the cells were cultured for 3 h at 37 °C (5% CO2). The cells treated with vanillin, 4-methylguaiacol, and 4-ethylguaiacol were determined as the sample group. Trolox (500 mg L−1) was used as the standard antioxidant and cell treatment with Trolox was conducted as described above. The cells treated with AAPH alone were determined as the AAPH group, and cells with no sample and AAPH treatment were determined as the control group.
RNA isolation and reverse transcription
After incubation with AAPH for 3 h, the medium was removed and the wells were washed two times with PBS. Then, the total cellular RNA was extracted with Trizol reagent (Life Technologies, Shanghai, China) according to the manufacturer's instructions. Afterwards, RNA samples were treated with DNAse and reverse transcribed into cDNA using a M-MLV Reverse Transcriptase Kit (Promega, Madison, MI, USA) using the manufacturer's protocol.
Real-time PCR
Expression of target genes (see list of genes below) in the samples were determined using real-time PCR. Real-time PCR was performed with a CFX96 Real-Time PCR detection system (Bio-Rad, CA, USA) by employing SYBR Green I. Primers used (forward; reverse) were: SOD: 5′-TGGAGATAATACAGCAGGCT-3′; 5′-AGTCACATTGCCCAAGTCTC-3′, CAT: 5′-CCTTCGACCCAAGCAA-3′; 5′-CGATGGCGGTGAGTGT-3′, GPx1: 5′-AGAAGTGCGAGGTGAACGGT-3′; 5′-CCCACCAGGAACTTCTCAAA-3′, Nrf2: 5′-AGTGTG GAGAGGTATGAGCC-3′; 5′-CGTTCCTCTCTGGGTAGTAA-3′, Keap1: 5′-AGAGCGGGATGAGTGGCA-3′; 5′-GCTGAATTAAGGCGGTTTGTC-3′, GAPDH: 5′-GGTGGTCTCCTCTGACTTCAACA-3′; 5′-GTTGCTGTAGCCAAATTCGTTGC-3′. Relative gene expression was calculated using the 2−ΔΔCt method, and GAPDH was selected as the reference gene. Results were presented as fold expression change relative to the control group.
Protein extraction
After incubation with AAPH for 3 h, the medium was removed and the wells were washed two times with PBS. Then, the cells of control group, AAPH group and groups treated with 500 mg L−1 VA, 4-MG, 4-EG or Trolox were digested with trypsin (Gibco, Carlsbad, CA, USA), lysed with RIPA lysis buffer (Solarbio Life Sciences, Beijing, China) and homogenized by passing the cells through syringe needles. The homogenates were centrifuged at 14
000g for 15 min at 4 °C, and the supernatants were decanted for analysis.
The total protein content of cell lysates was measured by a BCA protein assay kit (Nanjing Jiancheng Institute of Biotechnology, Nanjing, Jiangsu, China).
Western blot analysis
Western blot analysis was carried out according to a previous method35 with some modifications. Briefly, protein samples (20 μg per well) were resolved by SDS-PAGE (10%) and transferred onto nitrocellulose membranes (Millipore, Bedford, MA, USA). The membranes were soaked in a blocking solution of 5% BSA (Sigma-Aldrich, St. Louis, MO, USA) in tris-buffered saline (TBS, Sigma-Aldrich, St. Louis, MO, USA) containing 0.05% (v/v) Tween 20 (Amresco, Houston, TX, USA) for 50 min with gentle shaking. Then, they were incubated with 5 mL primary antibodies at a dilution of 1
:
2000 (v/v) overnight at 4 °C. Rabbit anti-SOD2/MnSOD antibody, anti-Nrf2 antibody, anti-GPX8 antibody, anti-Keap1 antibody, anti-GAPDH antibody (Abcam, Cambridge, UK) and anti-CAT antibody (GeneTex, Irvine, CA, USA) were used as primary antibodies. Afterwards, the membranes were washed five times with TBS containing 0.05% Tween 20 and incubated with the secondary antibody at a dilution of 1
:
5000 (v/v) for 40 min with gentle shaking. HRP-conjugated goat anti-rabbit IgG antibody (Beijing ComWin Biotech, Beijing, China) was used as the secondary antibody. Then, the membranes were washed six times with TBS containing 0.05% Tween 20. Finally, detection was carried out using ECL Western Blotting Detection Reagent (Millipore, Bedford, MA, USA). Western blot results were quantified using Quantity One software. Expression levels of proteins were determined via normalization to that of GAPDH. Relative expression level was determined as the ratio of intensity of the protein band to that of the corresponding GAPDH band, and finally expressed as a fold increase over the control group.
Statistical analysis
Two independent experiments were performed at least in triplicates. Data obtained from the GC-MS analysis, CCK-8 assay, real-time PCR analysis, and western blot analysis were expressed as mean ± standard deviation (SD). Statistical differences between groups were carried out using one-way ANOVA test with a post hoc Tukey test by SPSS22.0 statistical program (IBM Inc., New York, NY, USA). p < 0.05 was accepted as statistically significant.
Results
Quantification of vanillin, 4-methylguaiacol, and 4-ethylguaiacol in GJG
Concentrations of vanillin (VA), 4-methylguaiacol (4-MG), and 4-ethylguaiacol (4-EG) in GJG were determined by liquid–liquid extraction (LLE) combined with gas chromatography-mass spectrometry (GC-MS). Based on the result (as shown in Table 1), vanillin, 4-methylguaiacol, and 4-ethylguaiacol were present in all GJG samples. The concentration of vanillin ranged from 103 ± 4 μg L−1 to 284 ± 7 μg L−1, which was lower than those of 4-methylguaiacol (126 ± 4–1580 ± 20 μg L−1) and 4-ethylguaiacol (129 ± 4–1708 ± 9 μg L−1). In addition, the total concentration of vanillin, 4-methylguaiacol, and 4-ethylguaiacol was from 375 ± 12 μg L−1 to 3196 ± 34 μg L−1.
Cell viability
To investigate their effect on cell viability and determine the treatment doses, HepG2 cells were exposed for 24 h to various concentrations of VA, 4-MG, and 4-EG. As shown in Fig. 2, the cell viability decreased with an increase in treatment concentration of VA, 4-MG, and 4-EG, especially at 1500 and 2000 mg L−1, from which significant changes to cell viability were obtained (p < 0.05). For the same treatment concentrations, 4-MG had the greatest cytotoxic effect, especially at higher contents (1500 and 2000 mg L−1), followed by VA and then, 4-EG. Meanwhile lower treatment concentrations of VA, 4-MG and 4-EG caused less impact on HepG2 cells, and cell viability was above 80% when the treatment concentrations of VA, 4-MG and 4-EG were below 1500 mg L−1. Thus, three levels of treatment concentrations (i.e., high-level: 1000 mg L−1, medium-level: 500 mg L−1, and low-level: 100 mg L−1) were selected for real-time PCR analysis.
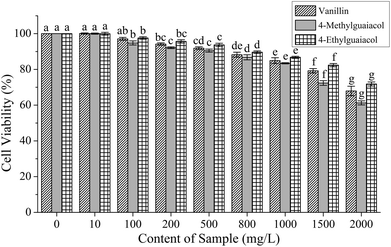 |
| Fig. 2 Cell viability of HepG2 cells treated with various doses of vanillin, 4-methylguaiacol, and 4-ethylguaiacol. Data are presented as the mean ± SD from three independent experiments. Different letters represent the significant difference between different contents of the same sample at p < 0.05. | |
Effect of vanillin, 4-methylguaiacol, and 4-ethylguaiacol on gene expressions in Keap1–Nrf2 pathway in AAPH-induced HepG2 cells
To confirm whether VA, 4-MG, and 4-EG protect HepG2 cells against AAPH-induced oxidative stress via activation of the Keap1–Nrf2 pathway, the gene and protein expressions in Keap1–Nrf2 pathway were measured with real-time PCR and western blot. The results of real-time PCR analysis were shown in Table S1 (ESI†) and in Fig. 3.
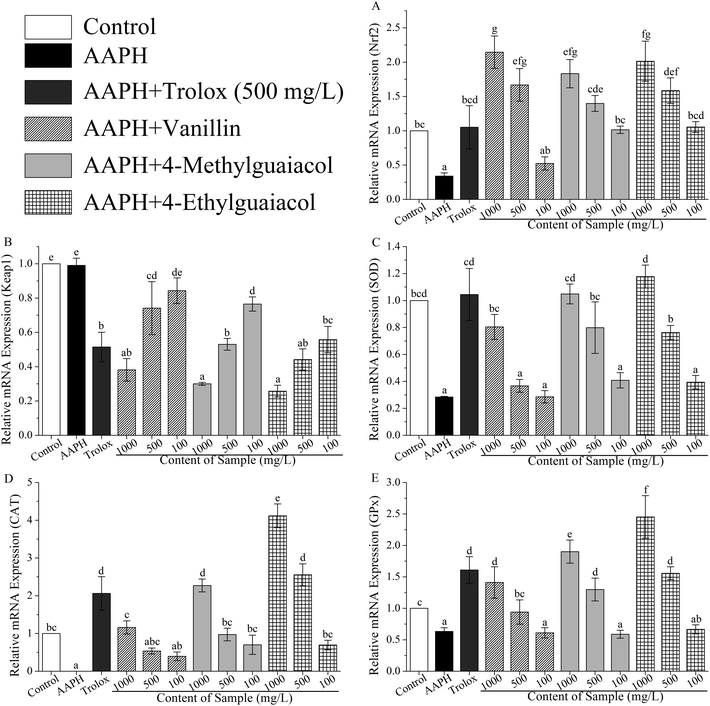 |
| Fig. 3 The effects of vanillin, 4-methylguaiacol, and 4-ethylguaiacol on AAPH-induced changes in mRNA expression levels of Nrf2 (A), Keap1 (B), SOD (C), CAT (D), and GPx (E) in HepG2 cells. Trolox is used as the positive standard. The results are expressed as relative values versus the control group. Data are shown as mean ± SD from three independent experiments. Different letters represent significant difference at p < 0.05. | |
As shown in Fig. 3A, mRNA expression of Nrf2 was significantly inhibited by AAPH (p < 0.05), and the level of Nrf2 in AAPH group was just 34% of the control group. VA, 4-MG, and 4-EG could remarkably up-regulate the mRNA expression level of Nrf2 compared with that of AAPH group, except for the low-level treatment group (100 mg L−1) of VA (p < 0.05). The level of Nrf2 in sample groups (i.e., samples treated with VA, 4-MG, and 4-EG) were increased by 54–531% times compared with that in AAPH group. In comparison with the control group, high-level (1000 mg L−1) of VA, 4-MG, and 4-EG critically enhanced the mRNA expression level of Nrf2 by 114, 83, and 101%, respectively (p < 0.05). As for medium-level (500 mg L−1), just VA and 4-EG could significantly induce mRNA expression of Nrf2 by 67 and 58%, respectively, as compared to the control group (p < 0.05). Although medium-level of 4-MG increased the Nrf2 mRNA level by 40% compared to the control group, no significant change was obtained. For low-level treatment, no significant difference was found between the sample group and the control group. In addition, high-level and medium-level of VA were interestingly more effective than 4-EG and 4-MG on induction of Nrf2 mRNA expression. However, at low-level treatment, 4-EG had the strongest upregulation ability, followed by 4-MG and VA, although no significant difference was observed for any compound. In contrast with Trolox, VA sharply enhanced the Nrf2 mRNA level by 59% at the same treatment concentration (500 mg L−1, p < 0.05), while for 4-MG and 4-EG, no significant change was obtained, although the Nrf2 mRNA level for 4-MG and 4-EG were increased by 33 and 51%, respectively. Overall, the protective effects of VA, 4-MG, and 4-EG on AAPH-induced changes in Nrf2 mRNA level were exhibited in a dose-dependent manner.
As for the mRNA expression of Keap1, in comparison with Nrf2, the results were quite different. As Fig. 3B showed, no significant change in Keap1 mRNA level was found between the AAPH and control groups. However, apart from the low-level of VA, Keap1 mRNA level was markedly down-regulated by VA, 4-MG, and 4-EG with respect to the AAPH and control groups (p < 0.05), where the level was decreased by 23–74% compared with AAPH group and control group. As for high-level treatment doses, 4-EG was observed to have the strongest downregulation ability, followed by 4-MG and VA, but no significant difference was found. While VA exerted the weakest ability at medium-level treatment with a remarkable difference (p < 0.05), and 4-EG exhibited the strongest ability at the low-level with significant difference (p < 0.05), followed by 4-MG and VA. As the same treatment level (500 mg L−1), the downregulation ability of Trolox was higher than that of 4-MG and lower than that of 4-EG, although no remarkable difference was observed. Meanwhile, the Keap1 mRNA level of VA treated sample (500 mg L−1) was significantly higher than that of Trolox treated one (p < 0.05). Also noted was that VA, 4-MG, and 4-EG exhibited a dose-dependent regulation of Keap1 mRNA level.
As shown in Fig. 3C, AAPH critically suppressed the SOD mRNA expression compared with the control group, as it decreased by 72% (p < 0.05), while VA, 4-MG, and 4-EG exhibited upregulation ability on SOD mRNA level in a dose-dependent manner. Among them, high-levels of VA, 4-MG, and 4-EG significantly promoted the SOD mRNA level by 183, 269, and 315%, respectively, with respect to the AAPH group (p < 0.05). Meanwhile, medium-levels of 4-MG and 4-EG could remarkably enhance the SOD mRNA level by 181 and 168% in comparison with the AAPH group (p < 0.05). There was no significant difference obtained among medium-level of VA, low-levels of VA, 4-MG, and 4-EG, and AAPH group. In addition, although the level of SOD in control group was higher than high-level of VA and medium-levels of 4-MG and 4-EG, and lower than high-levels of 4-MG and 4-EG, no remarkable difference was found. Notably, at medium- or low-levels, 4-MG had the strongest upregulation ability, followed by 4-EG and VA with no significant difference, whereas 4-EG was most effective in increasing the SOD mRNA level at high-level, followed by 4-MG and VA with no significant difference. At the same level, Trolox could significantly induce the SOD mRNA expression compared with VA and 4-EG (p < 0.05). Also, the SOD mRNA level of Trolox was higher than that from medium-level of 4-MG, but no significant difference was observed.
The results of CAT mRNA expression were presented in Fig. 3D. AAPH caused downregulation of CAT mRNA level at a critical change compared to the control group (p < 0.05), with CAT mRNA level almost reduced to zero. In contrast with AAPH group, VA, 4-MG, and 4-EG significantly promoted the mRNA level of CAT, except for the medium- and low-levels of VA (p < 0.05). Moreover, apart from VA, high-levels of 4-MG and 4-EG sharply increased the CAT mRNA level by 127 and 312%, respectively, in comparison with the control group (p < 0.05), giving a similar response as the medium-level of 4-EG. However, no remarkable difference was found between other sample groups and the control group. Also, 4-EG exhibited the best upregulation activity towards CAT mRNA level in a dose-dependent manner, followed by 4-MG and VA. In addition, for the same level of concentration (500 mg L−1), only 4-EG was more effective than Trolox with no significant difference, while VA and 4-MG were extremely less effective than Trolox (p < 0.05).
As shown in Fig. 3E, in contrast with the control group, AAPH critically suppressed the expression of GPx mRNA (p < 0.05), whereas VA, 4-MG, and 4-EG extremely enhanced the GPx mRNA level by 49–288% in a dose-dependent manner, except for low-levels of sample groups (p < 0.05). Also, apart from medium-level of VA, high- and medium-levels of VA, 4-MG, and 4-EG significantly promoted the GPx mRNA level by 30–145% compared to the control group (p < 0.05). Especially, the GPx mRNA level of 4-EG treated sample was higher than those of 4-MG and VA at the same level. But in contrast with Trolox, 4-EG and 4-MG exerted lower upregulation activity with no significant difference, while VA showed extremely weaker upregulation ability at the same level (500 mg L−1, p < 0.05).
Effect of vanillin, 4-methylguaiacol, and 4-ethylguaiacol on protein expressions in Keap1–Nrf2 pathway in AAPH-induced HepG2 cells
The protein expressions of Keap1–Nrf2 pathway were measured by western blot, and results were shown in Table S2 (ESI†) and in Fig. 4.
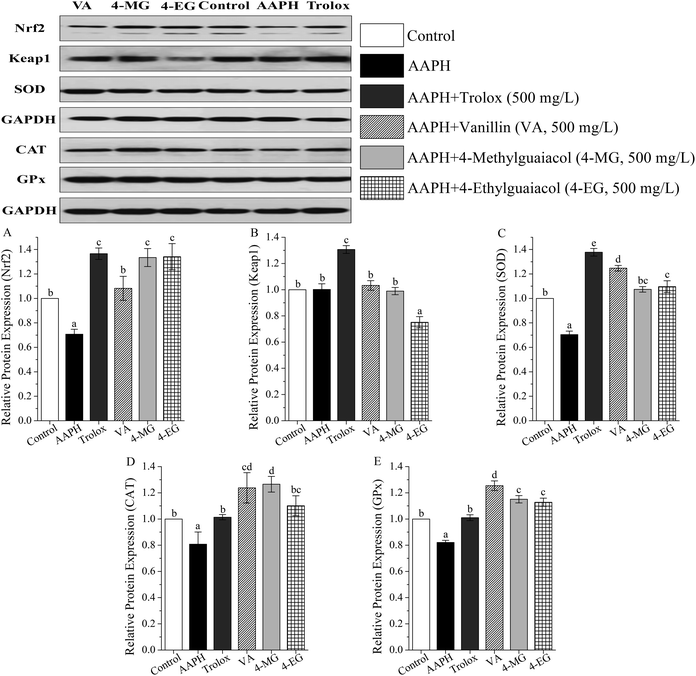 |
| Fig. 4 The effects of vanillin (VA), 4-methylguaiacol (4-MG), and 4-ethylguaiacol (4-EG) on AAPH-induced changes in protein expression levels of Nrf2 (A), Keap1 (B), SOD (C), CAT (D), and GPx (E) in HepG2 cells. Trolox is used as the positive standard. The results are expressed as relative values against the control group. Data are shown as mean ± SD from three independent experiments. Different letters represent significant difference at p < 0.05. | |
As Fig. 4 showed, in contrast to the control group, AAPH markedly suppressed protein expression of Nrf2, SOD, CAT, and GPx by 29, 30, 19, and 18%, respectively (p < 0.05), and no significant change in Keap1 protein level was obtained. Meanwhile, VA, 4-MG, and 4-EG exhibited upregulation of Nrf2 (53–90%), SOD (53–77%), CAT (36–57%), and GPx protein level (37–53%) with respect to the AAPH group (p < 0.05). Interestingly, only 4-EG could down-regulate the protein expression level of Keap1 by 25% in comparison with the AAPH group (p < 0.05). Compared with the control group, VA promoted the levels of Nrf2, SOD, CAT, and GPx by 8 (p > 0.05), 25 (p < 0.05), 24 (p < 0.05), and 25% (p < 0.05), respectively. 4-MG extremely enhanced the levels of Nrf2, CAT, and GPx by 33, 27, and 15%, respectively (p < 0.05), whereas no significant change in the level of SOD was observed. 4-EG markedly increased the levels of Nrf2, SOD, and GPx by 34, 10, and 13%, respectively (p < 0.05), however, no significant change in the level of CAT was obtained. Also noted was that among VA, 4-MG, and 4-EG, VA exhibited the strongest upregulation ability for SOD and GPx and the weakest upregulation ability for Nrf2 with a remarkable difference (p < 0.05), 4-EG showed the strongest downregulation ability on Keap1 with a remarkable difference (p < 0.05), while no significant difference in CAT level was found. In addition, VA, 4-MG, and 4-EG showed larger changes for CAT and GPx than Trolox, and smaller changes for Nrf2, Keap1, and SOD than Trolox.
Discussion
Recently, it has been widely confirmed that oxidative stress induced by excessive amounts of reactive oxygen species (ROS) can cause genomic instability, thereby leading to a variety of chronic diseases.36 Although humans possess enzymatic antioxidant defenses (SOD, CAT, and GPx, etc.), and repair systems to protect themselves against oxidative stress,37 these native antioxidant systems are generally unable to completely prevent oxidative damage in living organisms caused by oxidative stress, as evidenced by the rising incidences of chronic diseases associated with oxidative stress. Meanwhile, due to health and safety concerns, natural bioactive components in foods and beverages have been greatly addressed by people. Especially, phenolic compounds have received a great deal of attention due to their possible beneficial effects on human health. There has been increasing evidence that phenolic compounds may protect cell constituents against oxidative damage and therefore prevent or delay various chronic diseases associated with oxidative stress.12,38–40 However, available information on the cytoprotective effect of phenolic compounds in Chinese baijiu is scarce. Therefore, the aim of present study was to determine whether vanillin (VA), 4-methylguaiacol (4-MG), and 4-ethylguaiacol (4-EG) were widely present in Gujinggong (GJG) Chinese baijiu and whether these compounds could protect HepG2 cells against oxidative stress via activating the Nrf2 pathway, and to lay the foundation for better illustrating the healthy, protective properties of Chinese baijiu. Consequently, based on above analyses by GC-MS, real-time PCR and western blot, VA, 4-MG, and 4-EG were confirmed to be present in all GJG samples and indeed affected the Keap1–Nrf2 pathway—distinctly elevating the mRNA and protein expressions of Nrf2 and its downstream antioxidative enzymes (SOD, CAT, and GPx), thereby protecting HepG2 cells against AAPH-induced oxidative stress (shown in Fig. 5).
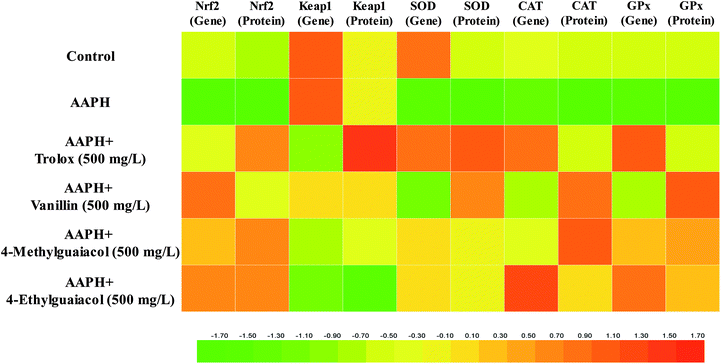 |
| Fig. 5 Effect of vanillin (VA), 4-methylguaiacol (4-MG), and 4-ethylguaiacol (4-EG) on gene and protein expression profiles of Nrf2, Keap1, SOD, CAT, and GPx in AAPH-induced HepG2 cells. Fold changes in the expression of gene and protein are color-coded according to the legend at the bottom. The color scale from green to red indicates the expression levels from low to high. | |
Nrf2 is well known for its critical role in the antioxidant defense system and is normally bound in the cytosol by Keap1.26 Moderate oxidative stress can cause the dissociation of Nrf2/Keap1 complex, releasing Nrf2 from Keap1, allowing Nrf2 to translocate into the nucleus and bind to the antioxidant response elements (AREs), and finally activating cytoprotective genes and accelerating the expression of numerous phase II detoxifying/antioxidant enzymes, such as SOD, CAT, and GPx, which contribute to protect cells from oxidative damage. However, excessive oxidative stress can wreak havoc on the antioxidant defense system, evidence of which was investigated in this study.
As shown in this research, in contrast with the control group, AAPH extremely suppressed the mRNA expression of Nrf2, SOD, CAT, and GPx (p < 0.05). As an important member of intracellular antioxidant defense system, SOD is responsible for faster dismutation of superoxide to hydrogen peroxide (H2O2), after which CAT transforms H2O2 into water and oxygen. GPx catalyzes the reaction of scavenging and destroying free radicals of GSH.41 Therefore, AAPH induced a great oxidative stress and destroyed the antioxidant defense system in HepG2 cells. However, VA, 4-MG, and 4-EG significantly promoted the mRNA levels of Nrf2, SOD, CAT, and GPx down-regulated by AAPH (p < 0.05). It has been widely demonstrated that phenolic compounds possess direct antioxidant activity, especially polyphenols.21,42 It is worthwhile to note that the direct antioxidant activity of polyphenols appears to be ineffective in vivo, because of low bioavailability and kinetic constraints.42 In addition, polyphenols are also confirmed to protect cells from oxidative damage by indirect antioxidant capacity.43 However, polyphenols must be metabolized into small molecular electrophilic compounds before exerting indirect antioxidant capacity (i.e., activating Nrf2 pathway and thus regulating the expression of phase II detoxifying/antioxidant enzymes).25 VA, 4-MG, and 4-EG, micromolecules which are generated during the grain fermentation process, seem to exert an antioxidant effect more directly than macromolecules, such as polyphenols. Of note also is that the mRNA expression level of CAT was more sensitive to oxidative stress caused by AAPH in comparison with to Nrf2, SOD, and GPx. In contrast with the control group, the mRNA levels of Nrf2, SOD, and GPx in the AAPH group were critically decreased by 66, 72, and 37%, respectively (p < 0.05). Meanwhile, the mRNA expression level of CAT was easily affected and almost decreased by 100% (p < 0.05), which concurred with a previous study.19 CAT activity was extremely decreased due to the excessive oxidative stress induced by AAPH and was near zero in HepG2 cells.19
While for the protein expression levels, oxidative stress induced by AAPH produced remarkable decrease in the expression of Nrf2, SOD, CAT, and GPx compared with the control group (p < 0.05). However, VA, 4-MG, and 4-EG was able to reverse the effect caused by AAPH, returning the expression levels of Nrf2, SOD, CAT, and GPx to or above control values. It has been well documented that phenolic compounds could modulate the protein expression levels of Nrf2 and its downstream antioxidative enzymes against oxidative stress.44,45 For instance, the expression level of SOD could be up-regulated by carnosol.46 Likewise, quercetin has been demonstrated to be effective in up-regulating the expression levels of Nrf2, SOD, and CAT against oxidative stress in albino rats treated with indomethacin.47 Also, curcumin has been indicated to ameliorate AAPH-induced oxidative stress in HepG2 cells by activating Nrf2,23 although the potential modulation effect of curcumin on antioxidative enzymes has not been verified in the study.
It is worth noticing that although VA, 4-MG, and 4-EG have similar chemical structures (one oxidizable phenolic hydroxyl group with a methoxy group in ortho position to it, as shown in Fig. 1), different effects on the expression of Nrf2 and antioxidative enzymes were observed for the three compounds. Specifically, analogous induction effect of VA, 4-MG, and 4-EG on Nrf2 mRNA was obtained as no significant was found at the same level, however, the weakest upregulation of Nrf2 was observed for VA with a significant difference (p < 0.05). 4-EG exhibited the most potent upregulation ability for SOD (p > 0.05), CAT (p < 0.05), and GPx mRNA levels (p < 0.05), followed by 4-MG and VA, whereas the lowest protein levels of CAT (p > 0.05) and GPx (p < 0.05) were also obtained from 4-EG. Moreover, VA had the strongest modulation effect on Nrf2 mRNA, although no remarkable difference among VA, 4-MG, and 4-EG was obtained. However, VA also exhibited the weakest modulation effect on mRNA of downstream antioxidative enzymes of Nrf2, but it exhibited the strongest upregulation of SOD and GPx (p < 0.05) at the same time. According to the above results, we thought that the difference in substitution of the aromatic compound might impact their combination with receptors for activating the Keap1–Nrf2 pathway.
In addition, the impact of VA, 4-MG, and 4-EG on the expression of mRNA did not parallel protein level changes. Comparing the relative expression levels of mRNA with that of correspondent proteins, higher relative expression levels were obtained for mRNA of Nrf2 and GPx, except in case of VA (mRNA level of GPx only), and for protein levels of SOD and CAT, except in case of 4-EG (protein level of CAT only). A previous study reported that VA, 4-MG, and 4-EG had strong direct antioxidant activities and could protect HepG2 cells against AAPH-induced oxidative stress via enhancing the activities of CAT, SOD and GPx.19 Thus, we inferred that VA, 4-MG, and 4-EG could induce the mRNA and protein expression of antioxidative enzymes in case of increasing oxidative stress caused by AAPH. However, when VA, 4-MG, and 4-EG or their induced antioxidative enzymes (SOD, CAT, and GPx) were enough to eliminate the free radicals (thereby suppressing oxidative stress, and maintaining the intracellular antioxidant defense system in HepG2 cells at a normal level) the mRNA expression of antioxidant enzymes was partially inhibited as secretion of more antioxidative enzymes was unnecessary. Therefore, the expression of mRNA and proteins was inconsistent. To sum up, according to the results of previous study and this study, VA, 4-MG, and 4-EG could prevent HepG2 cells from AAPH-induced oxidative stress by acting as antioxidants themselves by modulating genes and proteins expressions in the Keap1–Nrf2 pathway and improving the endogenous antioxidant enzymatic defense system.
Conclusions
In conclusion, this study determined the concentrations and cytoprotective effects of vanillin, 4-methylguaiacol, and 4-ethylguaiacol, three phenolic compounds of Chinese baijiu, by GC-MS and the AAPH-induced HepG2 cell model, respectively. Based on the analyses by GC-MS, real-time PCR and western blot, vanillin, 4-methylguaiacol, and 4-ethylguaiacol were detected in all GJG samples and were able to attenuate or reverse the increased oxidative stress resulting from AAPH via activating the Keap1-Nrf2 pathway, enhancing the mRNA and protein expressions of Nrf2 and its downstream antioxidative enzymes (SOD, CAT, and GPx), and thus, promoting the capacity of the intracellular antioxidant system.
Of note, this study provides a better illustration of the beneficial effects of Chinese baijiu on human health, but whether the concentrations of vanillin, 4-methylguaiacol, and 4-ethylguaiacol in Chinese baijiu are high enough to ameliorate AAPH-induced oxidative stress in HepG2 cells by activating Nrf2 is still unknown. Hence, further research, including (1) investigation of whether the concentrations of vanillin, 4-methylguaiacol, and 4-ethylguaiacol in Chinese baijiu are able to exhibit the property to protect cells against abnormal oxidative stress, (2) establishing an approach to increase the concentrations of vanillin, 4-methylguaiacol, and 4-ethylguaiacol in Chinese baijiu without affecting the aroma profile, (3) characterization of the effects of vanillin, 4-methylguaiacol, and 4-ethylguaiacol on other signaling kinases (e.g., mitogen-activated protein kinases (MAPK), protein kinase C (PKC), and phosphatidylinositol-3-kinase (PI3K), etc.) and phase II antioxidant enzymes (e.g., heme oxygenase 1 (HO-1) and NAD(P)H-quinone: oxidoreductase 1 (NQO1), etc.), and (4) evaluation of other potential bioactivities of vanillin, 4-methylguaiacol, and 4-ethylguaiacol needs to be carried out. To date, 1874 components in Chinese baijiu have been reported according to our studies.48 Therefore, additional data is needed to uncover the relationship between these numerous components in Chinese baijiu and human health.
Conflicts of interest
No potential conflict of interest was reported by the authors.
Abbreviations
ROS | Reactive oxygen species |
GPx | Glutathione peroxidase |
CAT | Catalase |
SOD | Superoxide dismutase |
VA | Vanillin |
4-MG | 4-Methylguaiacol |
4-EG | 4-Ethylguaiacol |
MDA | Malondialdehyde |
GSSG | Oxidized glutathione |
GSH | Reduced glutathione |
Keap1 | Kelch-like ECH-associated protein 1 |
Nrf2 | Nuclear factor erythroid 2-related factor 2 |
AREs | Antioxidant response elements |
AAPH | 2,2′-Azobis(2-methylpropionamide)-dihydrochloride |
HepG2 | Human hepatic cell line |
GJG | Gujinggong (one of the famous CB) |
CB | Chinese baijiu |
LLE | Liquid–liquid extraction |
GC-MS | Gas chromatography-mass spectrometry |
LOD | Limits of detection |
CCK-8 | Cell counting kit |
SD | Standard deviation |
Acknowledgements
This work was supported by the National Key R&D Program of China [2017YFC1600401] and the National Natural Science Foundation of China [31601556 and 31701567]. We deeply thank associate research fellow Xuelian Luo from Chinese Center for Disease Control and Prevention for her help with the cells culture aspect of this study.
Notes and references
- K. Ganesan and B. Xu, A Critical Review on Polyphenols and Health Benefits of Black Soybeans, Nutrients, 2017, 9, 455–471 CrossRef PubMed.
- S. Akhtar, A. Rauf, M. Imran, M. Qamar, M. Riaz and M. S. Mubarak, Black carrot (Daucus carota L.), dietary and health promoting perspectives of its polyphenols: A review, Trends Food Sci. Technol., 2017, 66, 36–47 CrossRef CAS.
- Y. Sang, F. Zhang, H. Wang, J. Yao, R. Chen, Z. Zhou, K. Yang, Y. Xie, T. Wan and H. Ding, Apigenin exhibits protective effects in a mouse model of D-galactose-induced aging via activating the Nrf2 pathway, Food Funct., 2017, 8, 2331–2340 RSC.
- R. S. Sohal, R. J. Mockett and W. C. Orr, Mechanisms of aging: An appraisal of the oxidative stress hypothesis, Free Radical Biol. Med., 2002, 33, 575–586 CrossRef CAS PubMed.
- S. Joko, M. Watanabe, H. Fuda, S. Takeda, T. Furukawa, S.-P. Hui, R. Shrestha and H. Chiba, Comparison of chemical structures and cytoprotection abilities between direct and indirect antioxidants, J. Funct. Foods, 2017, 35, 245–255 CrossRef CAS.
- M. Zhao, D. Zhu, D. Sun-Waterhouse, G. Su, L. Lin, X. Wang and Y. Dong, In Vitro and In Vivo Studies on Adlay-Derived Seed Extracts: Phenolic Profiles, Antioxidant Activities, Serum Uric Acid Suppression, and Xanthine Oxidase Inhibitory Effects, J. Agric. Food Chem., 2014, 62, 7771–7778 CrossRef CAS PubMed.
- M. Slavin, W. Kenworthy and L. Yu, Antioxidant Properties, Phytochemical Composition, and Antiproliferative Activity of Maryland-Grown Soybeans with Colored Seed Coats, J. Agric. Food Chem., 2009, 57, 11174–11185 CrossRef CAS PubMed.
- K. Zhou, L. Su and L. Yu, Phytochemicals and Antioxidant Properties in Wheat Bran, J. Agric. Food Chem., 2004, 52, 6108–6114 CrossRef CAS PubMed.
- Y. Chen, G. Chen, X. Fu and R.-H. Liu, Phytochemical Profiles and Antioxidant Activity of Different Varieties of Adinandra Tea (Adinandra Jack), J. Agric. Food Chem., 2015, 63, 169–176 CrossRef CAS PubMed.
- A. Ljevar, N. Curko, M. Tomasevic, K. Radosevic, V. G. Srcek and K. K. Ganic, Phenolic Composition, Antioxidant Capacity and in vitro Cytotoxicity Assessment of Fruit Wines, Food Technol. Biotechnol., 2016, 54, 145–155 CAS.
- C. I. G. Tuberoso, G. Serreli, F. Congiu, P. Montoro and M. A. Fenu, Characterization, phenolic profile, nitrogen compounds and antioxidant activity of Carignano wines, J. Food Compos. Anal., 2017, 58, 60–68 CrossRef CAS.
- X. Wang, K. Xie, H. Zhuang, R. Ye, Z. Fang and T. Feng, Volatile flavor compounds, total polyphenolic contents and antioxidant activities of a China gingko wine, Food Chem., 2015, 182, 41–46 CrossRef CAS PubMed.
- Z. Wu, E. Xu, J. Long, X. Pan, X. Xu, Z. Jin and A. Jiao, Comparison between ATR-IR, Raman, concatenated ATR-IR and Raman spectroscopy for the determination of total antioxidant capacity and total phenolic content of Chinese rice wine, Food Chem., 2016, 194, 671–679 CrossRef CAS PubMed.
- N. R. Mihailovic, V. B. Mihailovic, S. Kreft, A. R. Ciric, L. G. Joksovic and P. T. Djurdjevic, Analysis of phenolics in the peel and pulp of wild apples (Malus sylvestris (L.) Mill.), J. Food Compos. Anal., 2018, 67, 1–9 CrossRef CAS.
- N. Auzanneau, P. Weber, A. Kosinska-Cagnazzo and W. Andlauer, Bioactive compounds and antioxidant capacity of Lonicera caerulea berries: Comparison of seven cultivars over three harvesting years, J. Food Compos. Anal., 2018, 66, 81–89 CrossRef CAS.
- J. R. Oliveira Neto, T. Sardinha de Oliveira, P. C. Ghedini, B. G. Vaz and E. d. S. Gil, Antioxidant and vasodilatory activity of commercial
beers, J. Funct. Foods, 2017, 34, 130–138 CrossRef CAS.
- D. Zhao, D. Shi, J. Sun, A. Li, B. Sun, M. Zhao, F. Chen, X. Sun, H. Li, M. Huang and F. Zheng, Characterization of key aroma compounds in Gujinggong Chinese baijiu by gas chromatography-olfactometry, quantitative measurements, and sensory evaluation, Food Res. Int., 2018, 105, 616–627 CrossRef CAS PubMed.
- Y. Zheng, B. Sun, M. Zhao, F. Zheng, M. Huang, J. Sun, X. Sun and H. Li, Characterization of the Key Odorants in Chinese Zhima Aroma-Type Baijiu by Gas Chromatography-Olfactometry, Quantitative Measurements, Aroma Recombination, and Omission Studies, J. Agric. Food Chem., 2016, 64, 5367–5374 CrossRef CAS PubMed.
- D. Zhao, J. Sun, B. Sun, M. Zhao, F. Zheng, M. Huang, X. Sun and H. Li, Intracellular antioxidant effect of vanillin, 4-methylguaiacol and 4-ethylguaiacol: three components in Chinese baijiu, RSC Adv., 2017, 7, 46395–46405 RSC.
- T. Suzuki and M. Yamamoto, Molecular basis of the Keap1–Nrf2 system, Free Radical Biol. Med., 2015, 88, 93–100 CrossRef CAS PubMed.
- R. Mattera, M. Benvenuto, M. G. Giganti, I. Tresoldi, V. Manzari, A. Modesti, R. Bei, F. R. Pluchinotta, S. Bergante, G. Tettamanti, L. Masuelli, A. Modesti and R. Bei, Effects of Polyphenols on Oxidative Stress-Mediated Injury in Cardiomyocytes, Nutrients, 2017, 9, 523–565 CrossRef PubMed.
- H. Teng, T. Fang, Q. Lin, H. Song, B. Liu and L. Chen, Red raspberry and its anthocyanins: Bioactivity beyond antioxidant capacity, Trends Food Sci. Technol., 2017, 66, 153–165 CrossRef CAS.
- G.-N. Kim, Y.-J. Lee, J.-H. Song and H.-D. Jang, Curcumin ameliorates AAPH-induced oxidative stress in HepG2 cells by activating Nrf2, Food Sci. Biotechnol., 2013, 22, 241–247 CrossRef CAS.
- T. H. Rushmore, M. R. Morton and C. B. Pickett, The antioxidant responsive element. Activation by oxidative stress and identification of the DNA consensus sequence required for functional activity, J. Biol. Chem., 1991, 266, 11632–11639 CAS.
- T. Nguyen, P. Nioi and C. B. Pickett, The Nrf2-Antioxidant Response Element Signaling Pathway and Its Activation by Oxidative Stress, J. Biol. Chem., 2009, 284, 13291–13295 CrossRef CAS PubMed.
- K. R. Sekhar, X. X. Yan and M. L. Freeman, Nrf2 degradation by the ubiquitin proteasome pathway is inhibited by KIAA0132, the human homolog to INrf2, Oncogene, 2002, 21, 6829–6834 CrossRef CAS PubMed.
- H. Zhang, J. Wang, Y. Liu, L. Gong and B. Sun, Wheat bran feruloyl oligosaccharides ameliorate AAPH-induced oxidative stress in HepG2 cells via Nrf2 signalling, J. Funct. Foods, 2016, 25, 333–340 CrossRef CAS.
- H. Zhang, S. Zhang, J. Wang and B. Sun, Wheat bran feruloyl oligosaccharides protect against AAPH-induced oxidative injury via p38MAPK/PI3K-Nrf2/Keap1-MafK pathway, J. Funct. Foods, 2017, 29, 53–59 CrossRef CAS.
- S. Salla, R. Sunkara, S. Ogutu, L. T. Walker and M. Verghese, Antioxidant activity of papaya seed extracts against H2O2 induced oxidative stress in HepG2 cells, LWT--Food Sci. Technol., 2016, 66, 293–297 CrossRef CAS.
- C. Michiels, M. Raes, O. Toussaint and J. Remacle, Importance of Se-glutathione peroxidase, catalase, and Cu/Zn-SOD for cell survival against oxidative stress, Free Radical Biol. Med., 1994, 17, 235–248 CrossRef CAS PubMed.
- S. Magesh, Y. Chen and L. Hu, Small Molecule Modulators of Keap1–Nrf2–ARE Pathway as Potential Preventive and Therapeutic Agents, Med. Res. Rev., 2012, 32, 687–726 CrossRef CAS PubMed.
- X.-L. Jin, K. Wang, Q.-Q. Li, W.-L. Tian, X.-F. Xue, L.-M. Wu and F.-L. Hu, Antioxidant and anti-inflammatory effects of Chinese propolis during palmitic acid-induced lipotoxicity in cultured hepatocytes, J. Funct. Foods, 2017, 34, 216–223 CrossRef CAS.
- B. Svobodova, L. Barros, T. Sopik, R. C. Calhelha, S. Heleno, M. J. Alves, S. Walcott, V. Kuban and I. C. F. R. Ferreira, Non-edible parts of Solanum stramoniifolium Jacq. - a new potent source of bioactive extracts rich in phenolic compounds for functional foods, Food Funct., 2017, 8, 2013–2021 RSC.
- M. A. Martin, S. Ramos, R. Mateos, A. B. Granado Serrano, M. Izquierdo-Pulido, L. Bravo and L. Goya, Protection of Human HepG2 Cells against Oxidative Stress by Cocoa Phenolic Extract, J. Agric. Food Chem., 2008, 56, 7765–7772 CrossRef CAS PubMed.
- H. Zhang, H. Yousef, R. Liu, C. Yang, Y. Sun, R. Tsao and J. Renaud, Bioaccessibility, bioavailability and anti-inflammatory effects of anthocyanins from purple root vegetables using mono- and co-culture cell models, Mol. Nutr. Food Res., 2017, 61, 1600928–1600942 CrossRef PubMed.
- Y. Wang, Y. Wu, Y. Wang, H. Xu, X. Mei, D. Yu, Y. Wang and W. Li, Antioxidant Properties of Probiotic Bacteria, Nutrients, 2017, 9, 521–535 CrossRef PubMed.
- V. Mishra, C. Shah, N. Mokashe, R. Chavan, H. Yadav and J. Prajapati, Probiotics as Potential Antioxidants: A Systematic Review, J. Agric. Food Chem., 2015, 63, 3615–3626 CrossRef CAS PubMed.
- H.-J. Jung, Y. S. Song, K. Kim, C.-J. Lim and E.-H. Park, Assessment of the anti-angiogenic, anti-inflammatory and antinociceptive properties of ethyl vanillin, Arch. Pharmacal Res., 2010, 33, 309–316 CrossRef CAS PubMed.
- E. M. T. Padhi, R. Liu, M. Hernandez, R. Tsao and D. D. Ramdath, Total polyphenol content, carotenoid, tocopherol and fatty acid composition of commonly consumed Canadian pulses and their contribution to antioxidant activity, J. Funct. Foods, 2016, 38, 602–611 CrossRef.
- R. H. Soliman, O. A. Ismail, M. S. Badr and S. M. Nasr, Resveratrol ameliorates oxidative stress and organ dysfunction in Schistosoma mansoni infected mice, Exp. Parasitol., 2017, 174, 52–58 CrossRef CAS PubMed.
- G. M. M. Cunha, V. M. A. Silva, K. D. G. Bessa, M. A. O. Bitencourt, U. B. O. Macedo, F. P. Freire-Neto, R. R. Martins, C. F. Assis, T. M. A. M. Lemos, M. G. Almeida and A. C. G. Freire, Levels of oxidative stress markers: correlation with hepatic function and worm burden patients with schistosomiasis, Acta Parasitol., 2012, 57, 160–166 CAS.
- H. J. Forman, K. J. A. Davies and F. Ursini, How do nutritional antioxidants really work: Nucleophilic tone and para-hormesis versus free radical scavenging in vivo, Free Radical Biol. Med., 2014, 66, 24–35 CrossRef CAS PubMed.
- L. F. M. F. Cardozo, M. B. Stockler-Pinto and D. Mafra, Brazil nut consumption modulates Nrf2 expression in hemodialysis patients: A pilot study, Mol. Nutr. Food Res., 2016, 60, 1719–1724 CrossRef CAS PubMed.
- A. L. Eggler, K. A. Gay and A. D. Mesecar, Molecular mechanisms of natural products in chemoprevention: induction of cytoprotective enzymes by Nrf2, Mol. Nutr. Food Res., 2008, 52(suppl 1), S84–94 Search PubMed.
- J. W. Kaspar, S. K. Niture and A. K. Jaiswal, Nrf2:INrf2 (Keap1) signaling in oxidative stress, Free Radical Biol. Med., 2009, 47, 1304–1309 CrossRef CAS PubMed.
- S. Bartenbacher, C. Oestreicher and M. Pischetsrieder, Profiling of antioxidative enzyme expression induced by various food components using targeted proteome analysis, Mol. Nutr. Food Res., 2017, 61, 1600655–1600664 CrossRef PubMed.
- M. A. S. Abourehab, K. A. Khaled, H. A. A. Sarhan and O. A. A. Ahmed, Evaluation
of combined famotidine with quercetin for the treatment of peptic ulcer: in vivo animal study, Drug Des., Dev. Ther., 2015, 9, 2159–2169 CAS.
- H. Liu and B. Sun, Effect of Fermentation Processing on the Flavor of Baijiu, J. Agric. Food Chem., 2018, 66, 5425–5432 CrossRef CAS PubMed.
Footnote |
† Electronic supplementary information (ESI) available. See DOI: 10.1039/c8ra06505e |
|
This journal is © The Royal Society of Chemistry 2018 |