DOI:
10.1039/C8RA06274A
(Paper)
RSC Adv., 2018,
8, 35386-35394
Antibacterial properties of electrospun Ti3C2Tz (MXene)/chitosan nanofibers†
Received
24th July 2018
, Accepted 1st October 2018
First published on 15th October 2018
Abstract
Electrospun natural polymeric bandages are highly desirable due to their low-cost, biodegradability, non-toxicity and antimicrobial properties. Functionalization of these nanofibrous mats with two-dimensional nanomaterials is an attractive strategy to enhance the antibacterial effects. Herein, we demonstrate an electrospinning process to produce encapsulated delaminated Ti3C2Tz (MXene) flakes within chitosan nanofibers for passive antibacterial wound dressing applications. In vitro antibacterial studies were performed on crosslinked Ti3C2Tz/chitosan composite fibers against Gram-negative Escherichia coli (E. coli) and Gram-positive Staphylococcus aureus (S. aureus) – demonstrating a 95% and 62% reduction in colony forming units, respectively, following 4 h of treatment with the 0.75 wt% Ti3C2Tz – loaded nanofibers. Cytotoxicity studies to determine biocompatibility of the nanofibers indicated the antibacterial MXene/chitosan nanofibers are non-toxic. The incorporation of Ti3C2Tz single flakes on fiber morphology was analyzed by scanning electron microscopy (SEM) and transmission electron microscopy equipped with an energy-dispersive detector (TEM-EDS). Our results suggest that the electrospun Ti3C2Tz/chitosan nanofibers are a promising candidate material in wound healing applications.
1. Introduction
With the discovery of graphene, a two-dimensional (2D) material comprised of a single atomic layer of sp2 bonded carbons, interest in 2D materials suitable for biomedical applications has grown due to their inherent antibacterial activity, impressive mechanical properties, and thermal stability.1–4 MXenes—a family of 2D transition metal layered hexagonal ternary carbides and/or nitrides—have attracted a great deal of attention for applications including supercapacitors,5,6 batteries,7–9 water purification,10–12 catalysis,13–15 and desalination.16 These materials have a Mn+1XnTz composition, where M is an early transition metal, X is carbon or nitrogen, n = 1, 2, or 3, and T is a variable surface termination (i.e. OH, F, O, Cl).6,7,17 Currently there are only a few studies on the antibacterial behavior of MXene, predominantly for use as antibiofouling agents in wastewater treatment.18,19 However, the antibacterial properties of MXene for their potential use as medical bandaging materials have yet to be studied.
As MXenes are nanoscale materials, usually manufactured in colloidal suspension, a carrier polymer is required for accurate dosage and immobilization for use as a medical bandage. Electrospun nanofibers are an ideal carrier medium, as they exhibit desirable characteristics such as high surface area and porosity, flexibility in chemical functionalization, excellent permeability and high absorption capabilities.20 Chitosan (CS), a polysaccharide derivative of chitin, was chosen as a carrier polymer due to its biocompatibility, biodegradability, and low-cost. CS is a natural polymer that has been used in numerous studies for biomedical applications including drug-delivery systems, tissue engineering, wound healing, and antibacterial effects derived from its cationic nature.21–27 While electrospinning and crosslinking of chitosan is possible,28,29 most studies of electrospun chitosan for antibacterial applications use a copolymer to increase stability and electrospinnability.30–32 In this study, Ti3C2Tz (MXene) delaminated single and few layer flakes was electrospun within chitosan nanofibers for the first time. Only recently has MXene been successfully incorporated within electrospun polymers such as poly(acrylic acid) (PAA), poly(ethylene oxide) (PEO), poly(vinyl alcohol) (PVA), and alginate/PEO.33
Electrospun polymers functionalized with two-dimensional materials (i.e. graphene oxide (GO),34–37 exfoliated nanosheets of metal–organic frameworks (MOFs),38 transition-metal dichalcogenides (TMDCs),39 and layered zeolites40) and their antibacterial activities have been extensively studied. Chitosan/poly(lactide-co-glycolide) (PLGA) functionalized with GO decorated with silver nanoparticles via a two-step process displayed excellent antibacterial activity to Gram-negative (E. coli and P. aeruginosa) (inactivation rate of 99%) and Gram-positive (S. aureus) bacteria (inactivation rate of 76%).34 Antibacterial properties of electrospun GO sheets that were blended directly with PVA/chitosan displayed clear zones of inhibition, whose mean diameter increased with increasing GO loading.35 Previous studies attribute the antibacterial mechanism of GO to oxidative and physical stresses, including production of reactive oxygen species (ROS) and penetration of the bacterial membrane by the two-dimensional sharp edges.41 Similarly, the antibacterial effects of Ti3C2Tz flakes and membranes have been suggested to be due to their sharp edges, high surface hydrophilicity and action as a reducing agent.18,19 The antibacterial activity of Ti3C2Tz incorporated within electrospun nanofibers as a bandage medium; however, has never been studied.
In this study, we report on the fabrication of glutaraldehyde-crosslinked and basified Ti3C2Tz/CS nanofibers produced by electrospinning a blend of the natural polymer CS and delaminated Ti3C2Tz single and few-layer flakes colloidal suspensions. To realize the potential bandage applications of Ti3C2Tz/CS, the antibacterial effects of the nanofibers on the Gram-negative Escherichia coli (E. coli) and the Gram-positive Staphylococcus aureus (S. aureus) were studied. This work shows that even with a low Ti3C2Tz concentration in the composite (less than 1.0 wt%), substantial antibacterial activity is achieved.
2. Results and discussion
2.1 Synthesis and characterization of Ti3C2Tz
Ti3C2Tz is synthesized via the selective etching of Al layers from the starting material, Ti3AlC2, using a LiF/HCl solution as an etchant as illustrated in (Fig. 1a). The resultant multilayered (ML) Ti3C2Tz flakes are then washed with distilled water, centrifuged, and decanted to remove any remaining reaction by-products until a certain degree of spontaneous delamination occurs. A typical SEM image of the ML structure is shown in (Fig. 1b). The ML Ti3C2Tz is sonicated in water under a flow of a protective argon gas, to produce delaminated Ti3C2Tz, or a colloidal suspension of single and few-layer thick flakes as seen in (Fig. 1c and d), using SEM and TEM, respectively. The obtained Ti3C2Tz flake sizes agreed with other studies following similar etching and delamination procedures.42
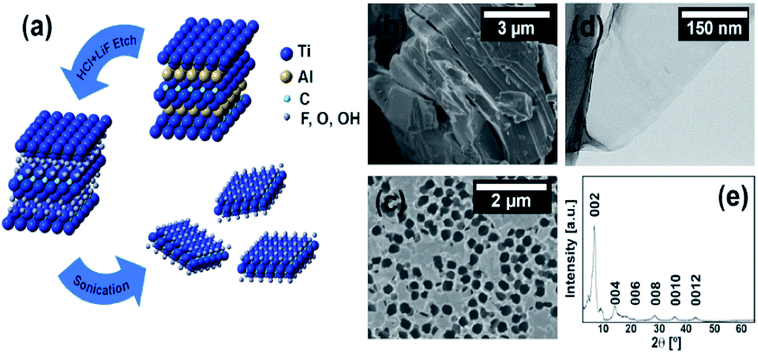 |
| Fig. 1 (a) Schematic detailing the removal of the Al layers from the Ti3AlC2 to yield ML-Ti3C2Tz that washing, and sonication then separate into individual Ti3C2Tz flakes. (b) SEM micrographs of ML-Ti3C2Tz powder and, (c) Ti3C2Tz single and few-layer thick flakes on an anodized alumina membrane. (d) TEM micrograph of a Ti3C2Tz flake. (e) XRD diffractogram of the vacuum assisted filtered Ti3C2Tz film. | |
A typical XRD pattern of a vacuum-assisted film of Ti3C2Tz flakes displays a sharp, intense (002) peak at 6.9°. This peak corresponds to (00l) basal-plane reflections with a c lattice parameter of 25 Å, indicative of the presence of water and Li cations between the negatively charged sheets.17 For this study, the antibacterial properties of delaminated Ti3C2Tz composites were investigated due to the previously demonstrated antibacterial effects of the flakes.
2.2 Morphology and structure of Ti3C2Tz/CS nanofiber mats
2.2.1. Ti3C2Tz/CS fiber morphology. SEM micrographs (Fig. 2a) show the as-spun CS mats with uniform, cylindrical fibers with an average fiber diameter of 211 ± 40 nm. Fine-tuning of electrospinning parameters led to consistent formation of uniform CS nanofibers with Ti3C2Tz loads of 0, 0.05, 0.10, 0.25, 0.50, and 0.75 wt%. These Ti3C2Tz concentrations were chosen because they consistently formed uniform, bead-free nanofibers. SEM micrographs of the Ti3C2Tz/CS nanofibers revealed the presence of dense networks of non-woven fibers of relatively uniform diameters (Fig. 2b–f). No branching of the fibers was observed, which suggests that the electrical forces and surface tension of the Ti3C2Tz/CS solution during electrospinning were properly balanced. Morphology of the Ti3C2Tz/CS nanofibers were visually comparable to those of the neat CS nanofibers. Average fiber diameters of the 0.05, 0.10, 0.25, 0.50, and 0.75 wt% Ti3C2Tz/CS were 236 ± 59, 170 ± 54, 311 ± 144, 279 ± 80, and 298 ± 76 nm respectively (Fig. S1†). The data failed Levene's test for homogeneity at a significance level of 0.05, so a Kruskal–Wallis non-parametric analysis of variance (ANOVA) was performed, finding significant differences between fiber diameters at p ≤ 0.05. Dunn's test was used for post hoc multiple comparison and indicated significant difference between the CS and the composite fibers containing 0.25, 0.50 and 0.75 wt% Ti3C2Tz. This difference in diameter may be due to the increasing water concentration in the electrospinning solutions from the increasing addition of Ti3C2Tz aqueous suspensions.
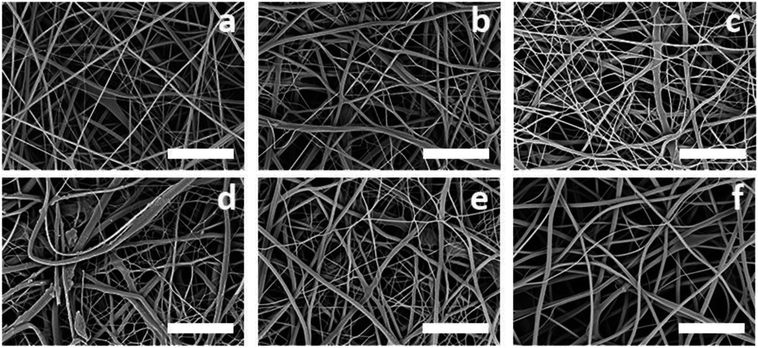 |
| Fig. 2 SEM micrographs displaying the surface of the as-spun electrospun crosslinked Ti3C2Tz/CS mats for the five different MXene loadings: (a) 0, (b) 0.05, (c) 0.10, (d) 0.25, (e) 0.50, and (f) 0.75 wt%. Scale bars represent 5 μm. | |
2.2.2. Ti3C2Tz/CS chemical functionalities. The chemical functionalities of as-spun CS and Ti3C2Tz/CS fiber mats was investigated by FT-IR spectroscopy (Fig. 3a) to ensure that the addition of Ti3C2Tz did not interfere in the crosslinking of the chitosan nanofibers. Typical CS bands at 3418 (hydroxyl group), 1203 (bridge ether oxygen), 1653 (amide I group), and 1532 cm−1 (amide II) appeared for all the nanofibrous mats (Table 1).43 The amine deformation displayed in the FT-IR spectra for both the neat and composite fibers indicates successful crosslinking, where glutaraldehyde undergoes a Schiff base reaction with the chitosan for the formation of an amine functionality and/or Michael-type adducts with terminal aldehydes to form carbonyl groups.44 As shown by the negligible differences between the spectra of the neat and composite nanofibers, the crosslinking of the chitosan was unaffected by the addition of Ti3C2Tz.
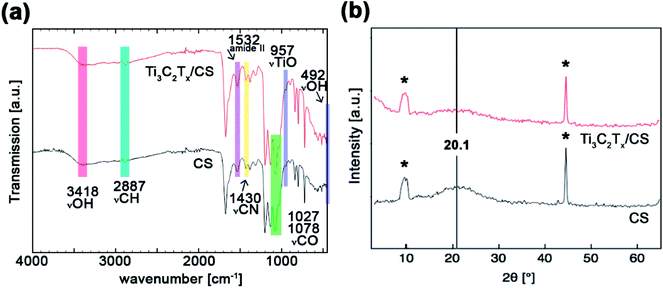 |
| Fig. 3 (a) FTIR spectra and (b) XRD patterns of the neat chitosan nanofibers and the 0.75 wt% Ti3C2Tz/CS nanofibers. Asterisks denote signal by the stage. | |
Table 1 FTIR peak annotations of the CS and Ti3C2Tz/CS nanofibers
Peak frequency, cm−1 |
Peak assignment |
References |
CS |
Ti3C2Tz/CS |
3418 |
492, 3418 |
−OH stretching |
28, 29, 46 and 62 |
— |
957 |
Ti–O stretching |
46 and 47 |
2887 |
2887 |
C–H stretching |
28, 29, 46 and 62 |
1653 |
1653 |
Amide I |
28, 29, 46 and 62 |
1532 |
1532 |
Amide II |
28, 29, 46 and 62 |
1430 |
1430 |
C–N stretching |
28, 29, 46 and 62 |
1203 |
1203 |
Bridge ether oxygen |
28, 29, 46 and 62 |
1078, 1027 |
1078, 1027 |
C–O stretching |
46 and 47 |
2.2.3. Ti3C2Tz/CS crystal structure. The effect of Ti3C2Tz on the overall crystal structure of the CS nanofibers was investigated by X-ray diffraction (Fig. 3b). The neat CS and Ti3C2Tz/CS nanofibers displayed similar XRD patterns with the typical amorphous broad reflection at 20.1°, signaling the retardation of the crystallization process of the chitosan blend.45 However, the introduction of Ti3C2Tz into chitosan nanofibers produced a somewhat broadened peak of decreased intensity, suggesting some form of interaction between the Ti3C2Tz and CS. More specifically, Ti3C2Tz single flakes may physically interfere with the semi-crystalline behavior of CS via interfacial interactions and steric hindrance.46
2.3 Characteristics of Ti3C2Tz immobilized within CS nanofibers
Two different interactions are likely to occur at the organic–inorganic interface between the negatively charged surface terminations (O, F, OH, Cl) of the Ti3C2Tz and the positively charged nitrogen-containing groups of chitosan. The first being electrostatic interactions and the second being the less dominant hydrogen bonding.47,48 Fig. 4a illustrates the orientations of Ti3C2Tz to the CS nanofibers based on these interactions.
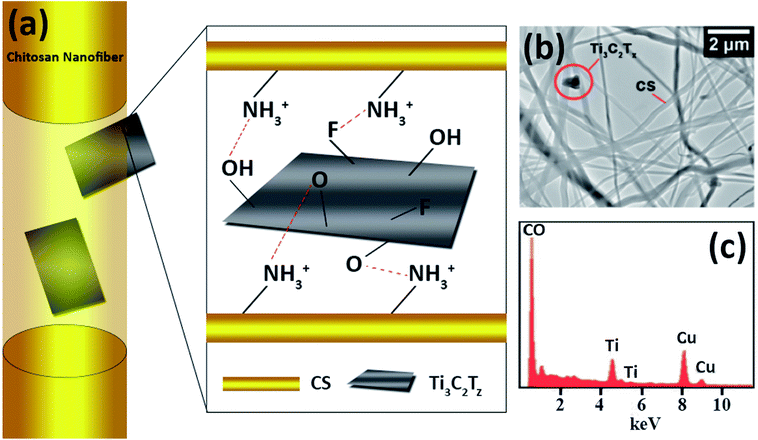 |
| Fig. 4 (a) Schematic diagram illustrating the observed orientations of Ti3C2Tz flakes within the chitosan nanofibers. (b) TEM micrograph of the Ti3C2Tz/CS nanofibers and (c) elemental analysis (the presence of copper arises from the TEM grid). | |
Due to the encapsulation of the Ti3C2Tz flakes within the chitosan nanofibers, they could not be visualized from the SEM micrographs. To identify the presence of Ti3C2Tz flakes, Ti3C2Tz/CS fiber mats were analyzed by TEM (Fig. 4b). The TEM micrographs enabled the simultaneous visualization of the chitosan nanofibers and the Ti3C2Tz flakes. Two orientations were observed: full embodiment of the Ti3C2Tz flake within the fiber and protrusion of the Ti3C2Tz flake, as suggested in Fig. 4. The TEM-EDS micrograph and results on the composite fibers shows the presence of Ti3C2Tz within the fiber (Fig. 4c). The chitosan was not doped and so the peaks for C and O are combined.
2.4 Antibacterial activity of electrospun Ti3C2Tz/CS mats
2.4.1. GA crosslinked CS and Ti3C2Tz/CS. The antibacterial activity of the glutaraldehyde-crosslinked 0.75 wt% Ti3C2Tz/CS composite nanofibers after 4 h of contact with E. coli and S. aureus is shown in (Fig. 5a and b). The glutaraldehyde-crosslinked Ti3C2Tz/CS fibers exhibited a bacterial cell reduction of over 95% for E. coli, relative to the unmodified control CS fibers. A lower cell reduction of 62% was achieved against S. aureus due to the thicker peptidoglycan cell wall of Gram-positive bacteria which may provide protection against the antibacterial effects of the Ti3C2Tz/CS composite nanofibers. Our results are in agreement with a previous study on PLGA/CS mats functionalized with silver nanoparticles, which reported higher cell reduction for E. coli than S. aureus.34 The E. coli data passes Levene's test for homogeneity of variance (significance level of 0.05), so a one-way ANOVA with post hoc Tukey test was performed. The S. aureus data also does not pass Levene's test for homogeneity of variance (significance level of 0.05), so a Kruskal–Wallis one-way ANOVA with post hoc Dunn's test at significance level 0.05 was performed.
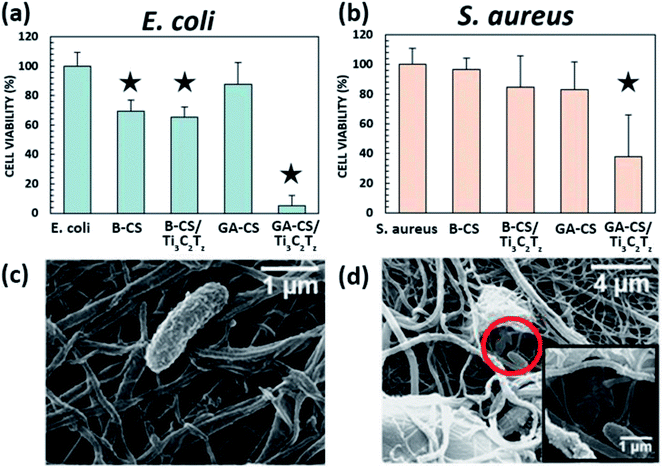 |
| Fig. 5 Antibacterial activity of, (a) E. coli and (b) S. aureus. B-X and GA-X indicate mats treated with NaOH and glutaraldehyde, respectively. SEM micrographs shows an, (c) intact and, (d) destroyed E. coli bacteria on the 0.75 wt% Ti3C2Tz/CS nanofiber mat. The star icon designates samples that are significantly different from the control, p ≤ 0.05. | |
2.4.2. Basified CS and Ti3C2Tz/CS. Two methods of crosslinking, glutaraldehyde vapor deposition and basification, were used to compare the effect on the nanofiber antibacterial efficacy as a function of their crosslinking stability. Basification remains a common method for creating aqueous insoluble CS nanofiber mats via removal of the acetate salt. The application of NaOH neutralizes the amines on the chitosan, which prevents the mat from immediately dissolving into solution, but does not prevent the mat from beginning to degrade and smoothing into a film.49The basified composite proved to be effective against E. coli but showed less antibacterial activity against S. aureus, indicating that the charged NH3 group may be required for S. aureus activity. While the mat did not disintegrate appreciably over the 4 h time period (measured via OD600, data not shown), this smoothing effect may also have covered surface MXene flakes, reducing their efficacy against the S. aureus cell wall. Both basified CS and basified MXene composite nanofiber mats were effective against more susceptible E. coli, which may be due to the inherent antibacterial activity of CS. The mechanism is proposed to be direct adsorption of dissolved water-insoluble CS onto the surface of the bacterial cell, forming an impervious layer that blocks membrane transport channels and leads to cell death.50 This is likely the same effect found in other studies of electrospun CS, as the combination of CS with a copolymer would not prevent bacteria from contacting regions of CS on the surface of the nanofibers.30–32
2.4.3. Antibacterial mechanism. Following exposure to the Ti3C2Tz/CS composite nanofibers, SEM micrographs of the attached cells were examined as shown in Fig. 5c and d. Morphological integrity was lost upon contact with the Ti3C2Tz/CS mats as seen by the flattened and burst features of the bacteria. These SEM micrographs are consistent with previous images obtained for E. coli following treatment with AgNPs.51While there are many proposed mechanisms for the antibacterial nature of Ti3C2Tz flakes,18,19 the most likely mechanisms involve direct contact of the bacteria with the MXene flakes. Direct mechanical destruction by MXene penetration through bacterial membranes has been observed by TEM,18 and is also considered to be the main cause of the antibacterial activity of two-dimensional GO.1–4,19 Additionally, the hydrophilic, negatively-charged flake surface (due to the reactive –OH, –F, and –O surface terminations) may promote agglomeration of the bacteria, a significant indicator of antimicrobial activity in GO.52 Despite being incorporated into the nanofibers, the Ti3C2Tz flakes retain a high degree of antibacterial effectiveness. Our findings compare well with other studies that tested 200 μg mL−1 Ti3C2Tz colloidal suspensions against E. coli – resulting in a 99% bacterial inhibition.18
2.5 Biocompatibility of electrospun Ti3C2Tz/CS mats
To determine the biocompatibility of Ti3C2Tz/CS nanofibers, an in vitro cytotoxicity test was performed using HeLa cells, with results evaluated using an alamarBlue® assay. As shown in Fig. 6, the average cell viabilities relative to the control were over 85% at all test concentrations. The data passed Levene's test for homogeneity (at a significance level of 0.05), so a one-way analysis of variance (ANOVA) was performed, and no difference between samples was found (p ≤ 0.05). This indicates that the mats are not cytotoxic to HeLa cells over 72 h of exposure.
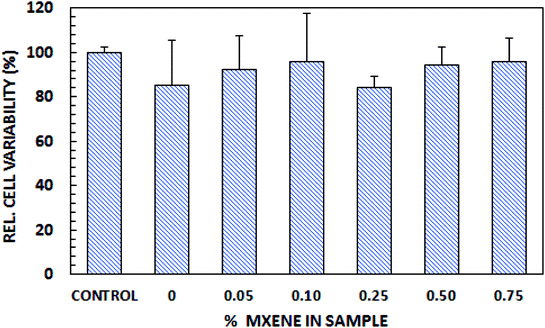 |
| Fig. 6 Biocompatibility of Ti3C2Tz/CS nanofibers. | |
2.6 Material comparison
A comprehensive literature review of electrospun materials with incorporated antibacterial two-dimensional and metal oxide nanoparticles (e.g. zinc oxide, titanium dioxide, and carbon nanotubes) clearly indicates that Ti3C2Tz/CS nanofibers are the most effective in reducing E. coli and S. aureus at incredibly low doses (e.g. 0.75 wt% Ti3C2Tz/CS). Given that the most common application method for these nanoparticles is spraycoating or dipcoating onto the surface of electrospun fibers, their effectiveness may be lowered as the particles may be washed away in aqueous environments. Electrospinning a composite nanofiber, as detailed in this paper, allows the nanoparticles to be fully or partially encapsulated, limiting surface erosion.
Though 2D materials and metal oxides display excellent antibacterial properties, it is important to consider their biocompatibility for use in wound healing dressings. For example, GO at higher doses was shown to exhibit stark cytotoxicity reflected in the protrusion into mitochondrion and cell nuclei thus leading to apoptosis.53 Carbon nanotubes have been found to promote inflammation and granuloma formation due to the formation of free radicals, release and accumulation of peroxidative products, depletion of antioxidant and oxidation of protein sulfhydryl groups.54,55 TiO2 was revealed to be toxic to the cells of major organs such as the brain and liver, causing acute necrosis. It was also demonstrated that TiO2 was capable of travelling through the placental barrier of pregnant mice and relaying neurotoxicity to their offspring.56 Despite these properties displayed by commonly studied 2D materials and metal oxides, Ti3C2Tz encapsulated by the chitosan nanofibers proved to be non-toxic. This is supported by previous studies demonstrating the high biocompatibilities of MnOx/Ti3C2 composite materials and functionalized Ti3C2 nanosheets with GdW10-based polyoxometalates (POMS) (Fig. 7).57,58
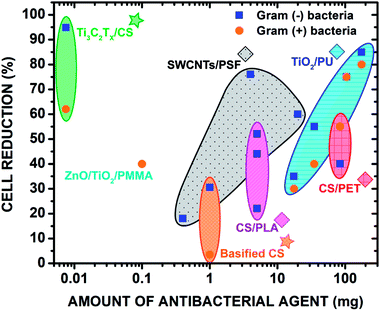 |
| Fig. 7 Comparison of the antibacterial properties with the previous literature of select polymeric nanofibers with incorporated metal oxides and 2D materials. The antibacterial effects of electrospun polymer blends were also compared. The stars denote the materials from this study. Copyright WILEY-VCH Verlag GmbH & Co. KGaA, 69469 Weinheim, Germany, 2016. | |
3. Conclusions
We present the functionalization of electrospun CS nanofiber mats with Ti3C2Tz flakes to develop flexible bandage materials with antimicrobial properties. Ti3C2Tz flakes were successfully incorporated into chitosan nanofibers without alteration to the nanofiber integrity as confirmed by SEM and TEM. Contact with the Ti3C2Tz/CS nanofiber surface imparted remarkable antibacterial activity, leading to a cell reduction rate of 95% against Gram-negative (E. coli) and 62% against Gram-positive (S. aureus) bacteria. Our findings strongly suggest the application of Ti3C2Tz/CS nanofiber mats as excellent wound dressing materials.
4. Experimental
4.1 Materials
Medium molecular weight chitosan with 77% deacetylation (MW = 190–310 kDa), glutaraldehyde solution (50%), ethanol (EtOH, 99.5%), sodium hydroxide (NaOH), phosphate buffered saline (PBS), glycine and hydrochloric acid (HCl, 37.0%) were obtained from Sigma Aldrich. Lithium fluoride (LiF, 99% trace metals basis) and trifluoroacetic acid (TFA, 99%) were obtained from Alfa Aesar. Sodium chloride (NaCl), BD Bacto™ Agar and yeast extract were purchased from Fisher Scientific and Tryptone from Oxoid. NEB® 5-alpha competent Escherichia coli was purchased from New England Biolabs and Staphylococcus aureus subsp. aureus Rosenbach was purchased from American Type Culture Collection (ATCC).
4.2 Synthesis and characterization of MXene (Ti3C2Tz)
A colloidal suspension of Ti3C2Tz flakes was obtained via sonication of multilayer Ti3C2Tz, after etching the MAX phase Ti3AlC2 in a LiF/HCl solution as described previously.42 Briefly, Ti3AlC2 (1.0 g) was gradually added to 12 M HCl solution (10 mL) containing LiF (1.0 g) and left to stir for 24 h at 35 °C. The solution was then washed five to eight times with deionized (DI) water via a procedure of agitation, centrifugation (3500 rpm/2301 × g for 2 min), and decantation, until the supernatant became dark in color. The solution was then sonicated in ice water for 1 h under flowing argon gas and centrifuged (5000 rpm/4696 × g for 1 h). Information regarding the morphological properties of Ti3C2Tz flakes was obtained through scanning electron microscopy (SEM) (Zeiss Supra 50VP SEM) and transmission electron microscopy (TEM) (JEOL JEM2100) operating at an accelerating voltage of 200 kV. Samples for the TEM, started as Ti3C2Tz flakes in a colloidal suspension, were diluted 1
:
20 in DI water, sonicated for 10 min in a water bath, and drop-cast onto copper aperture grids. Samples were allowed to fully dry before imaging. X-ray diffraction (XRD) patterns of a free-standing membrane comprised of Ti3C2Tz flakes were acquired with a Rigaku Smartlab with Cu K radiation with step size of 0.04 degrees and dwell time of 0.7 s per step.
4.3 Fabrication of Ti3C2Tz/CS nanofibers
Chitosan solutions 2.7% (w/v) were prepared in trifluoroacetic acid (TFA), and then loaded with MXene at 0, 0.05, 0.10, 0.25, 0.50 or 0.75 wt% based on the solid CS.28 The solution was rotated overnight or until uniform. The electrospinning setup was comprised of a high voltage power supply (20–25 kV), a syringe and metal needle (5 mL; 21 gauge), with a tip-to-copper collector distance of 10–14 cm. All solutions were pumped at 1 mL h−1 at room temperature (23.0 ± 0.5 °C) and under 9–15% relative humidity. The glutaraldehyde-crosslinked samples were prepared by exposing the electrospun fibers to glutaraldehyde vapor for 24 h in an airtight chamber.28,29,59 The basified samples were prepared by soaking in 1 M NaOH in EtOH for 0.5 h, then washed in changes of ethanol until the pH returned to neutral as measured via litmus paper.
4.4 Characterization of as-spun and crosslinked nanofibers
Fourier transform infrared spectroscopy (FTIR) spectra were recorded from 4000 to 400 cm−1 using a Perkin Elmer Spectrum Two FTIR spectrometer. X-ray diffraction (XRD) patterns of the fiber mats were acquired with a Rigaku Smartlab with Cu K radiation with step size of 0.04 degrees and dwell time of 0.7 s per step. Nanofiber morphology and size was examined in a SEM (Zeiss Supra 50VP) using an accelerating voltage of 3.50 kV. Average fiber diameters were calculated from fifty measurements per sample using ImageJ software.60 A TEM (JEOL JEM2100) equipped with an ultrathin window energy-dispersive detector (TEM-EDS) was used to identify Ti3C2Tz within the chitosan nanofibers using an accelerating voltage of 200 kV. Samples were prepared by placing them on a copper aperture grid. The hydrophilicity of the fiber surface was evaluated by measuring the water contact angle.
4.5 Antibacterial tests
To prepare for testing, the electrospun mats were weighed into 1.0 ± 0.1 mg samples and soaked in EtOH for 1 h to sterilize them (n = 4 per type). The EtOH was then removed, and the mats were allowed to dry completely under sterile conditions. The glutaraldehyde-crosslinked mats then had residual reactive aldehyde groups capped using a sterile saturated glycine solution. Finally, the mats were washed and conditioned in 3 changes of sterile 1× PBS. The bacterial strains were inoculated and cultured in sterilized lysogeny broth (LB) overnight in a shaking incubator at 37 °C. The cultures were then diluted to ∼3 × 108 CFU mL−1 in sterile PBS, corresponding to an OD600 of 0.375 ± 0.02 for E. coli and OD600 of 0.200 ± 0.02 for S. aureus. Both bacteria solutions were then diluted 1
:
1000 in sterile PBS to a concentration of ∼3 × 108 CFU mL−1, and 1 mL of this bacteria solution was combined with each sterile electrospun mat. All samples were prepared using sterile techniques and cultured in a shaking incubator at 37 °C for 4 h. The same volume of bacteria, without electrospun mats, was also incubated as a control. The antibacterial activity of the electrospun samples was assessed using the spread plate method.61 At 4 h incubation time, a serial dilution of each sample was plated onto LB agar plates using sterile techniques and incubated in a still incubator at 37 °C overnight. Following incubation, the colonies were counted using a Bio-Rad ChemiDoc™ MP System and Quantity One® 1-D analysis software with manual verification of each colony count. The dilution used for the spread plate method was previously determined and found to be within the statistically relevant range.61 The antibacterial effect was calculated relative to the controls containing no electrospun mats. After contact with the electrospun mats, the morphology of the attached bacteria cells was observed using a SEM. The fibers were first rinsed with sterile PBS pH 6.8 and fixed using 2.5% paraformaldehyde/2.5% glutaraldehyde diluted in 0.2 M Sorenson's buffer pH 7.2 for several hours. A series of water–ethanol treatments (50
:
50, 30
:
70, 20
:
80, 10
:
90, and 100 ethanol) for 10 min each were used to dehydrate the attached cells. The fibers were then dried at room temperature.
4.6 Cytotoxicity studies using mammalian cells
Cytotoxicity of the glutaraldehyde-crosslinked bandages was measured using HeLa cells cultured in complete Dulbecco's Modified Eagle Medium (DMEM supplemented with 10% fetal bovine serum (FBS) and 1% penicillin/streptomycin). Samples (1.0 ± 0.1 mg, n = 4 per type) had reactive aldehydes capped using a saturated glycine solution as detailed previously, and then were sterilized using EtOH and dried under UV light. Samples were added to a 24-well plate well containing 5 × 104 HeLa cells in 1.5 mL complete DMEM. The cells were then cultured for 72 h at 37 °C and 5% CO2. A negative control of the cells with no bandages present was also prepared. Cell viability was assessed using an alamarBlue® assay by first removing media and bandages from the well, adding 1.5 mL warmed complete media containing 10% alamarBlue® and incubating them for 4 h at 37 °C protected from light. The number of live cells was assessed by measuring absorbance at 570 nm, normalized to the 600 nm reading value. Cell viability was calculated relative to the control wells.
Conflicts of interest
There are no conflicts to declare.
Acknowledgements
The authors wish to acknowledge the entire Habas research lab at Temple University for assistance with bacterial studies. Furthermore, the authors would like to thank S. Kota and M. Ghidiu for their extensive and useful discussions as well as the Drexel Centralized Research Facility, S. Huang, and A. Lang for assistance with TEM imaging. Funding for this work was provided by the Swedish Research Council (621-2014-4890).
References
- D. Bitounis, H. Ali-Boucetta, B. H. Hong, D.-H. Min and K. Kostarelos, Adv. Mater., 2013, 25, 2258–2268 CrossRef CAS PubMed
. - C. Chung, Y.-K. Kim, D. Shin, S.-R. Ryoo, B. H. Hong and D.-H. Min, Acc. Chem. Res., 2013, 46, 2211–2224 CrossRef CAS PubMed
. - S. Gurunathan, J. W. Han, A. A. Dayem, V. Eppakayala and J.-H. Kim, Int. J. Nanomed., 2012, 7, 5901 CrossRef CAS PubMed
. - Y. Zhang, T. R. Nayak, H. Hong and W. Cai, Nanoscale, 2012, 4, 3833–3842 RSC
. - M. Naguib, O. Mashtalir, J. Carle, V. Presser, J. Lu, L. Hultman, Y. Gogotsi and M. W. Barsoum, ACS Nano, 2012, 6, 1322–1331 CrossRef CAS PubMed
. - M. Naguib, M. Kurtoglu, V. Presser, J. Lu, J. Niu, M. Heon, L. Hultman, Y. Gogotsi and M. W. Barsoum, Adv. Mater., 2011, 23, 4248–4253 CrossRef CAS PubMed
. - M. Naguib, J. Come, B. Dyatkin, V. Presser, P.-L. Taberna, P. Simon, M. W. Barsoum and Y. Gogotsi, Electrochem. Commun., 2012, 16, 61–64 CrossRef CAS
. - M. Naguib, J. Halim, J. Lu, K. M. Cook, L. Hultman, Y. Gogotsi and M. W. Barsoum, J. Am. Chem. Soc., 2013, 135, 15966–15969 CrossRef CAS PubMed
. - D. Er, J. Li, M. Naguib, Y. Gogotsi and V. B. Shenoy, ACS Appl. Mater. Interfaces, 2014, 6, 11173–11179 CrossRef CAS PubMed
. - Q. Peng, J. Guo, Q. Zhang, J. Xiang, B. Liu, A. Zhou, R. Liu and Y. Tian, J. Am. Chem. Soc., 2014, 136, 4113–4116 CrossRef CAS PubMed
. - Y. Ying, Y. Liu, X. Wang, Y. Mao, W. Cao, P. Hu and X. Peng, ACS Appl. Mater. Interfaces, 2015, 7, 1795–1803 CrossRef CAS PubMed
. - O. Mashtalir, K. M. Cook, V. N. Mochalin, M. Crowe, M. W. Barsoum and Y. Gogotsi, J. Mater. Chem. A, 2014, 2, 14334–14338 RSC
. - Z. W. Seh, K. D. Fredrickson, B. Anasori, J. Kibsgaard, A. L. Strickler, M. R. Lukatskaya, Y. Gogotsi, T. F. Jaramillo and A. Vojvodic, ACS Energy Lett., 2016, 1, 589–594 CrossRef CAS
. - J. Ran, G. Gao, F.-T. Li, T.-Y. Ma, A. Du and S.-Z. Qiao, Nat. Commun., 2017, 8, 13907 CrossRef CAS PubMed
. - H. Pan, Sci. Rep., 2016, 6, 32531 CrossRef CAS PubMed
. - C. E. Ren, K. B. Hatzell, M. Alhabeb, Z. Ling, K. A. Mahmoud and Y. Gogotsi, J. Phys. Chem. Lett., 2015, 6, 4026–4031 CrossRef CAS PubMed
. - M. Ghidiu, J. Halim, S. Kota, D. Bish, Y. Gogotsi and M. W. Barsoum, Chem. Mater., 2016, 28, 3507–3514 CrossRef CAS
. - K. Rasool, M. Helal, A. Ali, C. E. Ren, Y. Gogotsi and K. A. Mahmoud, ACS Nano, 2016, 10, 3674–3684 CrossRef CAS PubMed
. - K. Rasool, K. A. Mahmoud, D. J. Johnson, M. Helal, G. R. Berdiyorov and Y. Gogotsi, Sci. Rep., 2017, 7, 1598 CrossRef PubMed
. - S. Agarwal, J. H. Wendorff and A. Greiner, Polymer, 2008, 49, 5603–5621 CrossRef CAS
. - R. Jayakumar, M. Prabaharan, S. V. Nair and H. Tamura, Biotechnol. Adv., 2010, 28, 142–150 CrossRef CAS PubMed
. - H. Ueno, T. Mori and T. Fujinaga, Adv. Drug Delivery Rev., 2001, 52, 105–115 CrossRef CAS PubMed
. - T. Dai, M. Tanaka, Y.-Y. Huang and M. R. Hamblin, Expert Rev. Anti-Infect. Ther., 2011, 9, 857–879 CrossRef CAS PubMed
. - J.-K. F. Suh and H. W. Matthew, Biomaterials, 2000, 21, 2589–2598 CrossRef CAS PubMed
. - S. V. Madihally and H. W. Matthew, Biomaterials, 1999, 20, 1133–1142 CrossRef CAS PubMed
. - J. D. Schiffman and C. L. Schauer, Polym. Rev., 2008, 48, 317–352 CrossRef CAS
. - E. I. Rabea, M. E.-T. Badawy, C. V. Stevens, G. Smagghe and W. Steurbaut, Biomacromolecules, 2003, 4, 1457–1465 CrossRef CAS PubMed
. - J. D. Schiffman and C. L. Schauer, Biomacromolecules, 2007, 8, 2665–2667 CrossRef CAS PubMed
. - J. D. Schiffman and C. L. Schauer, Biomacromolecules, 2007, 8, 594–601 CrossRef CAS PubMed
. - A. Cooper, R. Oldinski, H. Ma, J. D. Bryers and M. Zhang, Carbohydr. Polym., 2013, 92, 254–259 CrossRef CAS PubMed
. - K.-H. Jung, M.-W. Huh, W. Meng, J. Yuan, S. H. Hyun, J.-S. Bae, S. M. Hudson and I.-K. Kang, J. Appl. Polym. Sci., 2007, 105, 2816–2823 CrossRef CAS
. - B. Son, B.-Y. Yeom, S. H. Song, C.-S. Lee and T. S. Hwang, J. Appl. Polym. Sci., 2009, 111, 2892–2899 CrossRef CAS
. - E. A. Mayerberger, O. Urbanek, R. M. McDaniel, R. M. Street, M. W. Barsoum and C. L. Schauer, J. Appl. Polym. Sci., 2017, 134(37), 45295 CrossRef
. - A. F. De Faria, F. Perreault, E. Shaulsky, L. H. Arias Chavez and M. Elimelech, ACS Appl. Mater. Interfaces, 2015, 7, 12751–12759 CrossRef CAS PubMed
. - Y. Liu, M. Park, H. K. Shin, B. Pant, J. Choi, Y. W. Park, J. Y. Lee, S.-J. Park and H.-Y. Kim, J. Ind. Eng. Chem., 2014, 20, 4415–4420 CrossRef CAS
. - H. R. Pant, C. H. Park, L. D. Tijing, A. Amarjargal, D.-H. Lee and C. S. Kim, Colloids Surf., A, 2012, 407, 121–125 CrossRef CAS
. - Y. Y. Qi, Z. X. Tai, D. F. Sun, J. T. Chen, H. B. Ma, X. B. Yan, B. Liu and Q. J. Xue, J. Appl. Polym. Sci., 2013, 127, 1885–1894 CrossRef CAS
. - J. Quirós, K. Boltes, S. Aguado, R. G. de Villoria, J. J. Vilatela and R. Rosal, Chem. Eng. J., 2015, 262, 189–197 CrossRef
. - S. Wu, J. Wang, L. Jin, Y. Li and Z. Wang, ACS Appl. Nano Mater., 2018, 1, 337–343 CrossRef CAS
. - K. A. Rieger, H. J. Cho, H. F. Yeung, W. Fan and J. D. Schiffman, ACS Appl. Mater. Interfaces, 2016, 8, 3032–3040 CrossRef CAS PubMed
. - O. Akhavan and E. Ghaderi, ACS Nano, 2010, 4, 5731–5736 CrossRef CAS PubMed
. - M. Ghidiu, M. R. Lukatskaya, M.-Q. Zhao, Y. Gogotsi and M. W. Barsoum, Nature, 2014, 516, 78 CAS
. - A. Pawlak and M. Mucha, Thermochim. Acta, 2003, 396, 153–166 CrossRef CAS
. - J. D. Schiffman and C. L. Schauer, Biomacromolecules, 2007, 8, 2665–2667 CrossRef CAS PubMed
. - Y.-T. Jia, J. Gong, X.-H. Gu, H.-Y. Kim, J. Dong and X.-Y. Shen, Carbohydr. Polym., 2007, 67, 403–409 CrossRef CAS
. - Y. Liu, J. Zhang, X. Zhang, Y. Li and J. Wang, ACS Appl. Mater. Interfaces, 2016, 8, 20352–20363 CrossRef CAS PubMed
. - M. Boota, M. Pasini, F. Galeotti, W. Porzio, M.-Q. Zhao, J. Halim and Y. Gogotsi, Chem. Mater., 2017, 29, 2731–2738 CrossRef CAS
. - O. Mashtalir, M. R. Lukatskaya, M.-Q. Zhao, M. W. Barsoum and Y. Gogotsi, Adv. Mater., 2015, 27, 3501–3506 CrossRef CAS PubMed
. - M. A. Kiechel and C. L. Schauer, Carbohydr. Polym., 2013, 95, 123–133 CrossRef CAS PubMed
. - C. Qin, H. Li, Q. Xiao, Y. Liu, J. Zhu and Y. Du, Carbohydr. Polym., 2006, 63, 367–374 CrossRef CAS
. - I. Sondi and B. Salopek-Sondi, J. Colloid Interface Sci., 2004, 275, 177–182 CrossRef CAS PubMed
. - S. Liu, T. H. Zeng, M. Hofmann, E. Burcombe, J. Wei, R. Jiang, J. Kong and Y. Chen, ACS Nano, 2011, 5, 6971–6980 CrossRef CAS PubMed
. - K. Wang, J. Ruan, H. Song, J. Zhang, Y. Wo, S. Guo and D. Cui, Nanoscale Res. Lett., 2011, 6, 8 Search PubMed
. - A. Shvedova, V. Castranova, E. Kisin, D. Schwegler-Berry, A. Murray, V. Gandelsman, A. Maynard and P. Baron, J. Toxicol. Environ. Health, Part A, 2003, 66, 1909–1926 CrossRef CAS PubMed
. - C. Salvador-Morales, E. Flahaut, E. Sim, J. Sloan, M. L. Green and R. B. Sim, Mol. Immunol., 2006, 43, 193–201 CrossRef CAS PubMed
. - P. Naserzadeh, F. Ghanbary, P. Ashtari, E. Seydi, K. Ashtari and M. Akbari, J. Biomed. Mater. Res., Part A, 2018, 106, 580–589 CrossRef CAS PubMed
. - C. Dai, H. Lin, G. Xu, Z. Liu, R. Wu and Y. Chen, Chem. Mater., 2017, 29, 8637–8652 CrossRef CAS
. - L. Zong, H. Wu, H. Lin and Y. Chen, Nano Res., 2018, 1–20 Search PubMed
. - A. E. Donius, M. A. Kiechel, C. L. Schauer and U. G. Wegst, J. R. Soc., Interface, 2013, 10, 20120946 CrossRef PubMed
. - W. S. Rasband, http://imagej.nih.gov/ij.
- S. Sutton, Journal of Validation Technology, 2011, 17, 42 Search PubMed
. - J. Brugnerotto, J. Lizardi, F. M. Goycoolea, W. Argüelles-Monal, J. Desbrieres and M. Rinaudo, Polymer, 2001, 42, 3569–3580 CrossRef CAS
.
Footnotes |
† Electronic supplementary information (ESI) available. See DOI: 10.1039/c8ra06274a |
‡ Indicates equal contribution. |
|
This journal is © The Royal Society of Chemistry 2018 |
Click here to see how this site uses Cookies. View our privacy policy here.