DOI:
10.1039/C8RA06260A
(Paper)
RSC Adv., 2018,
8, 30512-30519
Enhanced production of optical (S)-acetoin by a recombinant Escherichia coli whole-cell biocatalyst with NADH regeneration†
Received
24th July 2018
, Accepted 21st August 2018
First published on 29th August 2018
Abstract
Acetoin is an important platform chemical with a variety of applications in foods, cosmetics, chemical synthesis, and especially in the asymmetric synthesis of optically active pharmaceuticals. It is also a useful breath biomarker for early lung cancer diagnosis. In order to enhance production of optical (S)-acetoin and facilitate this building block for a series of chiral pharmaceuticals derivatives, we have developed a systematic approach using in situ-NADH regeneration systems and promising diacetyl reductase. Under optimal conditions, we have obtained 52.9 g L−1 of (S)-acetoin with an enantiomeric purity of 99.5% and a productivity of 6.2 g (L h)−1. The results reported in this study demonstrated that the production of (S)-acetoin could be effectively improved through the engineering of cofactor regeneration with promising diacetyl reductase. The systematic approach developed in this study could also be applied to synthesize other optically active α-hydroxy ketones, which may provide valuable benefits for the study of drug development.
1. Introduction
Enantiomeric α-hydroxy ketones are important pharmaceutical intermediates, widely applied in antifungal agents, antitumor antibiotics, antidepressants, farnesyl transferase inhibitors and selective inhibitors of amyloid-β protein production.1–3 Acetoin (3-hydroxy-2-butanone), the smallest natural chiral α-hydroxy ketone, is a popular flavor additive, preservative, plant growth promoter and breath biomarker of early lung cancer, and is identified as one of the top 30 potential building blocks.4–7 This four-carbon compound has one chiral center in the molecule and therefore exists as two stereoisomers, (R)-acetoin and (S)-acetoin.8,9 With FEMA no. 2008, acetoin is a substance generally recognized as safe (visit https://ntp.niehs.nih.gov/ for toxicity and related information). Acetoin and its isomers are widely applied in foods, cosmetics, chemical synthesis, especially in asymmetric synthesis of optically active pharmaceuticals and liquid crystals.10,11 Its derivate, 4-chloro-4,5-dimethyl-1,3-dioxolan-2-one, has been extensively utilized in modifying antibiotics to synthesize optically active drugs, which could enhance drug targeting properties and reduce side effects.12,13
Native microorganisms generally produce acetoin as a mixture of two stereoisomers, in which (R)-acetoin is predominant and (S)-acetoin exists as a minor by-product, leading to very low yield of (S)-acetoin and high cost of chiral separation.14–16 Liu et al. developed an engineered Lactococcus lactis to produce (S)-acetoin from glucose.17 However, the nonenzymatic oxidative decarboxylation of α-acetolactate to diacetyl occurred spontaneously and inefficiently, the engineered strain could only produce 5.8 g L−1 of (S)-acetoin with a productivity of 0.19 g (L h)−1. Several attempts to produce (S)-acetoin by using purified enzymes have been reported previously. According to Loschonsky et al., cyclohexane-1,2-dione hydrolase formed (S)-acetoin with a 95% enantiomeric excess from 23 mM substrate by homocoupling of acetaldehyde.18 In 2013, Gao et al. developed a two-enzymes coupling system, in which NADPH-dependent carbonyl reductase reduced diacetyl to produce (S)-acetoin, and glucose dehydrogenase was used to regenerate NADPH. Under optimal conditions, 12.2 g L−1 of (S)-acetoin (enantiomeric purity: 96.9%) with a productivity of 9.8 g (L h)−1 was produced.19
Recently, recombinant whole-cells have emerged as biocatalysts for (S)-acetoin production. He et al.20 described a whole-cell biocatalytic process with a high yield by recombinant E. coli cells co-expressing (2R,3R)-2,3-butanediol dehydrogenase (R,R-BDH), NADH oxidase and hemoglobin. The recombinant strain produced 72.4 g L−1 (S)-acetoin from a mixtures of 2,3-butanediol isomers. However, the enantiomeric purity of the product was only 94.7% for the generation of (R)-acetoin. Since (S)-acetoin can be used to provide chiral groups in high-value pharmaceuticals or for liquid crystals, high optical purity (i.e., over 98.0%) is particularly required. Gao et al. developed a whole-cell biocatalytic process for (S)-acetoin production by over-expressing diacetyl reductase (DAR) in E. coli.12 When diacetyl and glucose were used as substrate and co-substrate respectively, the recombinant whole-cells could produce 39.4 g L−1 of (S)-acetoin in 20 h. Since diacetyl is a prochiral compound that can be produced by chemical synthesis or microbial fermentation, it is a preferred substrate for optical (S)-acetoin.12,19,21,22 However, two problems still need to be solved. First, due to the high concentration of intracellular DAR, the endogenous cofactor regeneration system of E. coli is typically not sufficiently efficient,23,24 limiting the titer and productivity of (S)-acetoin; second, organic acids (e.g. acetic acid and lactic acid) produced during glucose metabolism make product recovery difficult and increase glucose consumption.25
In this study, an efficient and economical process was developed for optical (S)-acetoin production from diacetyl. As shown in Fig. 1, two in situ-NADH regeneration systems were introduced into E. coli and determined their effectiveness in (S)-acetoin production. DAR genes from several species were codon-optimized and over-expressed to screen for a promising DAR with high stereoselectivity, specific activity and stability. Finally, a series of whole-cell biocatalytic reactions were performed to achieve high (S)-acetoin production.
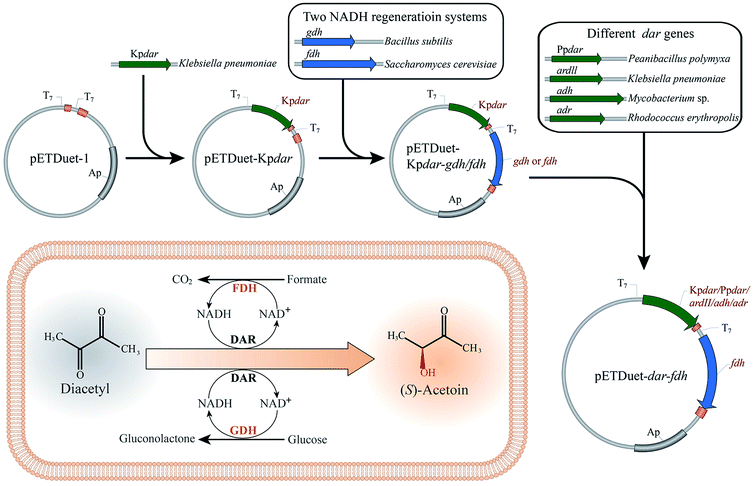 |
| Fig. 1 Scheme for (S)-acetoin production from diacetyl using recombinant whole-cell biocatalysts with NADH regeneration systems. dar, the gene encoding diacetyl reductase (DAR); gdh, the gene encoding glucose dehydrogenase (GDH); fdh, the gene encoding formate dehydrogenase (FDH); Ap: the gene encoding ampicillin resistance. | |
2. Materials and methods
2.1 Chemicals
(2S,3S)-2,3-Butanediol (99.0%) and meso-2,3-butanediol (98.0%) were obtained from ACROS (Geel, Belgium). (2R,3R)-2,3-Butanediol (97.0%), acetoin (99.0%), NADH, and NAD+ were obtained from Sigma (St Louis, USA). Diacetyl (99.0%) was obtained from Aladdin (Shanghai, China). PCR primers were synthesized by Sangon (Shanghai, China) and DNA sequencing was performed by Genscript (Nanjing, China). Yeast extract and peptone were obtained from Oxoid (Hampshire, UK).
2.2 Bacterial strains and vectors
The strains and vectors used in the present study are listed in Table 1. E. coli DH5α and E. coli BL21(DE3) were used for gene cloning and protein expressing, respectively. The pET-28a(+) and pETDuet-1 were used for gene expression. Luria–Bertani medium (10 g L−1 peptone, 5 g L−1 yeast extract, and 10 g L−1 NaCl at pH 7.0) was used for E. coli cultivation and solidified with 1.5% agar, if necessary. Kanamycin and ampicillin were used at concentrations of 30 μg mL−1 and 100 μg mL−1, respectively.
Table 1 Strains and plasmids used in this study
Strain and plasmid |
Genotype and properties |
Source or reference |
Strain |
E.coli DH5α |
supE44△lacU169 (φ80 lacZ△M15) hsdR17 recA1 endA1 gyrA96 thi-1 relA1 |
Novagen |
E.coli BL21(DE3) |
F− ompT hsdSB (rB−mB−) gal (λ c I 857 ind1 Sam7 nin5 lacUV5 T7gene1) dcm (DE3) |
Novagen |
![[thin space (1/6-em)]](https://www.rsc.org/images/entities/char_2009.gif) |
Plasmid |
pET-28a(+) |
Overexpression vector; Kanr |
Novagen |
pETDuet-1 |
Overexpression vector; Ampr |
Novagen |
pET-28a(+)-Kpdar |
Kpdar in pET-28a(+) |
This work |
pETDuet-fdh |
fdh in pETDuet-1 |
This work |
pETDuet-Kpdar |
Kpdar in pETDuet-1 |
This work |
pETDuet-Kpdar-gdh |
Kpdar and gdh in pETDuet-1 |
This work |
pETDuet-Kpdar-fdh |
Kpdar and fdh in pETDuet-1 |
This work |
pETDuet-Ppdar-fdh |
Ppdar and fdh in pETDuet-1 |
This work |
pETDuet-ard II-fdh |
ard II and fdh in pETDuet-1 |
This work |
pETDuet-adh-fdh |
adh and fdh in pETDuet-1 |
This work |
pETDuet-adr-fdh |
adr and fdh in pETDuet-1 |
This work |
2.3 Synthesis of DAR genes and construction of co-expression systems
DNA manipulations were carried out using standard protocols.26 The codons for the DAR genes, Kpdar from K. pneumoniae CICC 10011 (GenBank accession no. HM113375.1), Ppdar from P. polymyxa DSM 365 (GenBank accession no. KT717931), ard II from K. pneumoniae 342 (GenBank accession no. CP000964.1), adh from Mycobacterium sp. B-009 (GenBank accession no. AB905197.1), and adr from R. erythropolis WZ010 (GenBank accession no. KC508606.1), were optimized and synthesized by Genscript (Nanjing, China), and then inserted into pETDuet-1 between the Nco I and Sac I sites to yield expression vectors. The formate dehydrogenase (FDH) gene fdh from S. cerevisiae (NCBI reference sequence NM_001183808.1), and Glucose dehydrogenase (GDH) gene gdh from B. subtilis (GenBank accession no. CP019663.1), were also synthesized with codon optimization. The fdh and gdh fragments were digested with Nde I and Kpn I, respectively. Then the fragments were ligated into the expression vectors that had been digested with the same restriction endonucleases. The co-expression plasmids were designated pETDuet-Kpdar-gdh, pETDuet-Kpdar-fdh, pETDuet-Ppdar-fdh, pETDuet-ard II-fdh, pETDuet-adh-fdh, and pETDuet-adr-fdh, respectively.
2.4 Biocatalyst preparation and bioconversion conditions
The recombinant E. coli strains were cultivated at 37 °C and 180 rpm to OD600 of 0.6. According to preliminary experiments, recombinant genes expression was induced with 0.2 mM IPTG at 25 °C for 12 h. The cells were harvested by centrifugation at 6000 rpm and then washed twice with 0.85% NaCl. The cell pellet was resuspended in 100 mM phosphate buffer (pH 7.5) at a concentration of 20 g(DCW) L−1 and maintained at 4 °C. The dry cell weight (DCW) of E. coli was determined by converting the OD600 value with a coefficient of 0.275 g(DCW) per (LOD600).27
A typical bioconversion reaction was carried out with 5.5 g(DCW) L−1 whole-cell biocatalyst in 50 mL flasks. A total of 10 mL of the mixture was reacted in 100 mM phosphate buffer (pH 7.5) at 32 °C and 200 rpm for 2 h. (S)-Acetoin production by whole-cells co-expressing fdh with DAR genes was carried out using 15.0 g L−1 of diacetyl and 12.0 g L−1 of formate (only account molecular mass of formate ion). For (S)-acetoin production by whole-cells co-expressing Kpdar with gdh, 15.0 g L−1 of diacetyl and 30.0 g L−1 of glucose were required.
2.5 Optimization of the reaction conditions
To achieve a high conversion efficiency, the pH (5.5, 6.0, 6.5, 7.0, 7.5, or 8.0), temperature (28 °C, 32 °C, 36 °C, 40 °C, or 45 °C), molar ratio of formate to diacetyl (0.6, 0.8, 1.0, 1.2, 1.6, or 2.0), and shaking speed (75 rpm, 100 rpm, 125 rpm, 150 rpm, or 175 rpm) were investigated in turn. An initial bioconversion was reacted in 100 mM phosphate buffer at 32 °C and 200 rpm for 2 h. The concentrations of cell, diacetyl and formate in the reaction was 5.5 g(DCW) L−1, 15 g L−1 and 12 g L−1, respectively.
2.6 Fed-batch biotransformation
Fed-batch biotransformation was carried out in a 10 mL mixture at 40 °C and 125 rpm. The initial concentrations of diacetyl and whole-cell biocatalyst were 15.0 g L−1 and 5.5 g(DCW) L−1, respectively. The ratio of formate to diacetyl was 1.2, and the pH was 6.5. During bioconversion, diacetyl was fed at intervals to maintain its concentration at 5.0–15.0 g L−1, and pH was adjusted to 6.5 by formic acid. At the same time, small amounts of whole cell biocatalyst were added at the interval time, until the whole cell concentration reached to 11.0 g(DCW) L−1.
2.7 Purification of KpDAR
E. coli BL21(DE3) carrying plasmid pET-28a (+)-Kpdar was cultivated at 37 °C and 200 rpm to OD600 of 0.6, and then 0.4 mM IPTG was added to the culture at 20 °C for 12 h. The culture was centrifuged at 6000 rpm, and the cell pellet was resuspended in buffer (pH 7.4; 20 mM potassium phosphate, 500 mM sodium chloride, 20 mM imidazole, 1 mM DTT, 0.1 mM PMSF, and 10% glycerol). Cells were disrupted by sonication in an ice bath and centrifuged at 12
000 rpm for 30 min at 4 °C. The Kpdar was purified by HisTrap HP column (GE Healthcare) and detected by SDS-PAGE.
2.8 Assays for DARs, GDH and FDH activities
Crude proteins were extracted from recombinant E. coli using BugBuster Master Mix reagent (Novagen). Enzyme activities were determined by monitoring the change in optical density at 340 nm corresponding to the reduction of NAD+ or oxidation of NADH (ε340 = 6.22/mM cm) using a UV/visible spectrophotometer (DU800, Beckman). One unit of DAR activity was defined as the amount of enzyme that consumed 1 μmol of NADH per min. The reaction mixture contained 5 mM diacetyl and 0.2 mM NADH in 100 mM phosphate buffer (pH 6.5). One unit of GDH or FDH activity was defined as the amount of enzyme that produced 1 μmol of NADH per min. The reaction mixture contained 5 mM substrate (glucose or formate) and 0.2 mM NAD+ in 100 mM phosphate buffer (pH 6.5). The reaction solution for the KpDAR reduction assay contained 5 mM of ketone substrate and 0.2 mM of NADH in 100 mM phosphate buffer and for KpDAR oxidation contained 5 mM of alcohol substrate and 0.2 mM of NAD+ in 100 mM phosphate buffer. Protein concentrations were determined via the Bradford method using bovine serum albumin as a standard.
2.9 Analytical methods
The concentrations of diacetyl, acetoin, and 2,3-butanediol in the supernatant were determined using a gas chromatography (GC) system (Agilent 7890A) equipped with a polar column (Phenomenex ZB-WAX plus, 0.32 mm × 0.25 μm × 30 m). The injector and detector temperatures were both 250 °C. The column oven temperature was maintained at 100 °C for 1 min, then increased to 180 °C at a rate of 20 °C min−1, finally maintained at 180 °C for 3 min. (R)-Acetoin and (S)-acetoin in the supernatant were extracted using ethyl acetate and then measured by the GC system (Agilent 7890A) equipped with a chiral column (Agilent Cyclosil-B, 0.32 mm × 0.25 μm × 30 m). The temperatures of injector and detector were both 240 °C. The column oven temperature was maintained at 100 °C for 1 min and increased to 120 °C at a rate of 10 °C min−1. Then, it was increased to 130 °C at a rate of 6 °C min−1 and finally to 230 °C at a rate of 20 °C min−1. The enantiomeric purity of (S)-acetoin was defined as [S]/([S] + [R]) × 100%, where [R] and [S] represented the concentrations of (R)-acetoin and (S)-acetoin, respectively. Formate and other organic acids concentration were analyzed using high-performance liquid chromatography (HPLC) system (Dionex UltiMate 3000) equipped with a Carbomix H-NP column (Sepax, 7.8 × 300 mm). The analysis was conducted at 55 °C with a mobile phase of 2.5 mM H2SO4 at a flow rate of 0.6 mL min−1.
3. Results and discussion
3.1 Construction of different whole-cell biocatalysts by cofactor engineering
Redox balance plays an important role for cell growth and cellular metabolism.28 When heterogeneous oxidoreductases are overexpressed, the endogenous cofactor regeneration system is typically not sufficiently efficient.23 GDH and FDH are widely used for in situ-cofactor regeneration.25,29–31 To investigate their effect on (S)-acetoin production, gdh gene from Bacillus subtilis32 and fdh gene from Saccharomyces cerevisiae33 were synthesized after codon optimization (see ESI Table S1†) by GenScript (Nanjing, China) and co-expressed with DAR gene in E. coli BL21(DE3). To obtain a promising DAR, five DAR genes from several species were codon-optimized (see ESI Table S1†) and co-expressed with fdh or gdh to compare for (S)-acetoin synthesis. Recombinant DARs were detected by SDS-PAGE (see ESI Fig. S1†).
Batch biotransformation was conducted to evaluate the performance of two NADH regeneration systems with formate or glucose as co-substrate. As shown in Table 2, the conversion rate was low, obtained by E. coli BL21/pETDuet-Kpdar with single expression of KpDAR, and the final titer of (S)-acetoin was only 0.8 g L−1 with an enantiomeric purity of 89.3% and productivity of 0.4 g (L h)−1. The crude extract enzyme of E. coli BL21/pETDuet-Kpdar-gdh showed a KpDAR activity of 33.4 U mg−1 and a GDH activity of 16.3 U mg−1. When introducing the GDH/glucose system, the titer of (S)-acetoin increased to 4.3 g L−1 with an enantiomeric purity of 94.4% and productivity of 2.2 g (L h)−1 by E. coli BL21/pETDuet-Kpdar-gdh. Introduction of the FDH/formate system resulted in a higher (S)-acetoin titer, productivity and enantiomeric purity. 6.7 g L−1 of (S)-acetoin was obtained with an enantiomeric purity of 99.6% and productivity of 3.4 g (L h)−1 by E. coli BL21/pETDuet-Kpdar-fdh. Crude extract of this recombinant strain showed a KpDAR activity of 34.9 U mg−1 and a FDH activity of 0.45 U mg−1, while E. coli BL21/pETDuet-Kpdar showed a KpDAR activity of 79.0 U mg−1.
Table 2 The products of batch bioconversion with different DARs and cofactor regeneration systems
Strain |
(S)-Acetoin (g L−1) |
Productivity (g (L h)−1) |
Enantiomeric purity (%) |
E. coli BL21/pETDuet-Kpdar |
0.8 ± 0.01 |
0.4 |
89.3 ± 0.97 |
E. coli BL21/pETDuet-Kpdar-gdh |
4.3 ± 0.12 |
2.2 |
94.4 ± 0.79 |
E. coli BL21/pETDuet-Kpdar-fdh |
6.7 ± 0.08 |
3.4 |
99.6 ± 0.08 |
E. coli BL21/pETDuet-Ppdar-fdh |
3.2 ± 0.04 |
1.6 |
95.9 ± 0.77 |
E. coli BL21/pETDuet-ard II-fdh |
1.2 ± 0.02 |
0.6 |
54.5 ± 0.07 |
E. coli BL21/pETDuet-adh-fdh |
1.8 ± 0.03 |
0.4 |
90.2 ± 0.09 |
E. coli BL21/pETDuet-adr-fdh |
5.5 ± 0.15 |
2.8 |
98.5 ± 0.19 |
Acetoin biosynthesis initiates with the condensation of two pyruvate molecules to yield one α-acetolactate, then yield (R)-acetoin by decarboxylases or diacetyl upon spontaneous decarboxylation.24,34,35 Diacetyl can be further converted into (R)-acetoin or (S)-acetoin by DAR or BDH. Liang et al. reported that E. coli has an endogenous acetoin/2,3-butanediol pathway and glucose can be metabolized to acetoin by diacetyl-dependent endogenous system.24 In our study, the enantiomeric purity of (S)-acetoin produced by E. coli BL21/pETDuet-Kpdar-gdh with glucose as co-substrate was low (94.4%), indicating that (R)-acetoin was produced by the intrinstic acetoin/2,3-butanediol pathway of E. coli. While in FDH/formate system, only a trace amount of (R)-acetoin was detected for the absence of glucose, and the enantiomeric purity of (S)-acetoin can reach to 99.6% by E. coli BL21/pETDuet-Kpdar-fdh.
Low-molecular-weight formate has similar price with glucose. Regeneration of 1 mol of NADH requires only 45 g of formate comparing 172 g of glucose, indicating that the reducing equivalent capacity of formate is approximately four-fold higher than that of glucose.30 In addition, glucose can be metabolized to acetic acid, lactic acid by E. coli, further increasing glucose consumption.25 FDH catalyses the conversion of formate to CO2 with no organic acid products, reducing the cost associated with the co-substrate and downstream processing.30,31,36 Considering the higher enantiomeric purity and titter, lower cost of the co-substrate, and easier purification, the FDH/formate system would significantly reduce total cost of (S)-acetoin production.
When co-expressing fdh with other DAR genes including Ppdar, ard II, adr, or adh, all resulting biocatalysts produced (S)-acetoin, but the titer and enantiomeric purity of (S)-acetoin varied widely (Table 2). Because E. coli BL21/pETDuet-Kpdar-fdh showed the best performance in terms of both titer and enantiomeric purity among all recombinant strains, this strain together with KpDAR was used for subsequent analysis.
3.2 Purification and characterization of KpDAR
KpDAR was purified by Ni-affinity chromatography and confirmed by SDS-PAGE. The purified KpDAR showed single bands about 28 kDa on the SDS-PAGE (see ESI Fig. S2†). Oxidation and reduction reactions were conducted to investigate the substrate specificities of KpDAR (see ESI Table S2†). KpDAR had clear activities towards diacetyl, (R)/(S)-acetoin and meso-2,3-butanediol with NADH/NAD+ as the cofactor. Diacetyl was the best substrate in the ketone reduction reactions. The specific activity of purified KpDAR towards diacetyl was up to 2887.6 U mg−1. meso-2,3-Butanediol was the best substrate in the alcohol oxidation reactions, while very low activity was observed with (R)/(S)-acetoin, (2S,3S)-2,3-butanediol and (2R,3R)-2,3-butanediol.
3.3 Optimization of the reaction conditions
The reaction conditions for (S)-acetoin production were optimized to increase the efficiency of the whole-cell biocatalysis. Because reaction pH and temperature are two important parameters that strongly influence enzyme activities and cellular maintenance, the effects of pH and temperature on whole-cell biocatalysis were first investigated. As shown in Fig. 2a, the titer (9.19 g L−1) and yield (59.9%) of (S)-acetoin was high at pH 6.5. Under more acidic or alkaline conditions, the yield of the transformation decreased. As shown in Fig. 2b, the optimal temperature was found at 40 °C, and the yield of (S)-acetoin increased to 67.8%.
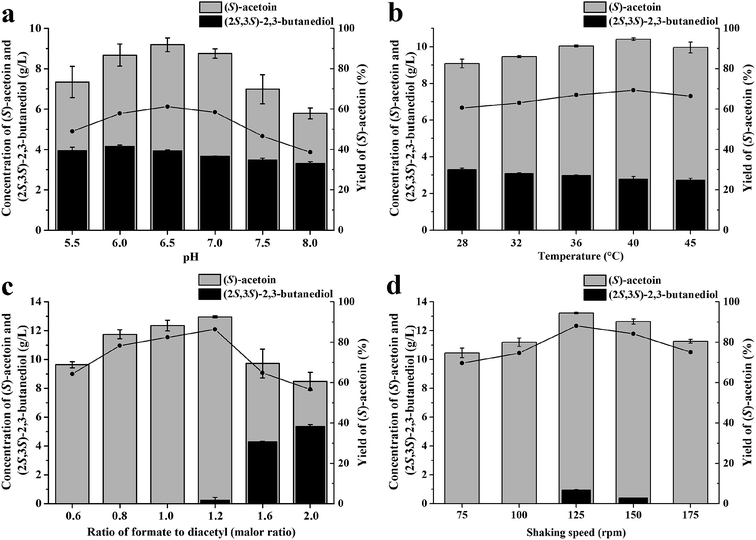 |
| Fig. 2 Optimization of biocatalysis conditions. (a) pH; (b) temperature; (c) ratio of formate to diacetyl; (d) shaking speed. (●) Yield of (S)-acetoin. Error bars indicate standard deviations (n = 3). | |
The substrate specificities of KpDAR indicated that KpDAR not only showed a high catalytic efficiency toward diacetyl, but also exhibited acetoin reductase activity (see ESI Table S2 and Fig. S3†). Both reductions of diacetyl and (S)-acetoin are accomplished with stoichiometric amounts of NADH consumption. Through manipulating the level of NADH/NAD+, production of (S)-acetoin can be improved.25 Thus, to enhance (S)-acetoin production, the concentration of formate should be optimized. As shown in Fig. 2c, when the molar ratio of formate to diacetyl was low, increasing the concentration of formate effectively increased the efficiency of (S)-acetoin production. When the molar ratio was 1.2, (S)-acetoin production reached its maximum level (12.96 g L−1) with a yield of 84.4%. Further increasing formate concentration to provide more NADH resulted in accumulation of (2S,3S)-2,3-butanediol, thus decreased the final yield of (S)-acetoin.
Oxygen supply can significantly affect the ratio of NADH/NAD+. Different oxygen supplies were investigated by varying the shaking speeds, and the optimal shaking speed was 125 rpm, as shown in Fig. 2d. Under optimal conditions, 13.2 g L−1 of (S)-acetoin was accumulated from 15.0 g L−1 of diacetyl with a productivity of 6.6 g (L h)−1 (Fig. 2d). The yield of (S)-acetoin was 86.0% and the enantiomeric purity was 99.6% (Fig. 3a and b).
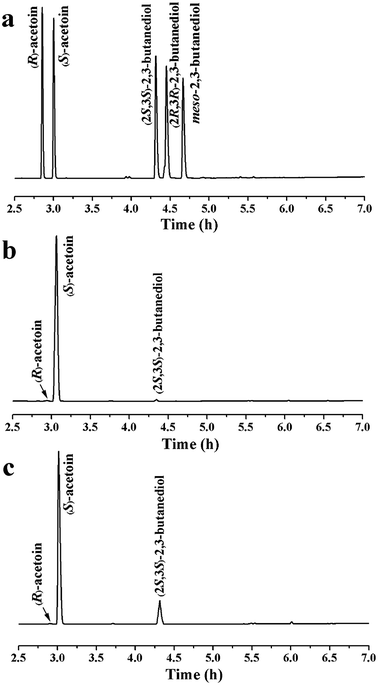 |
| Fig. 3 Chiral-column GC analyses of products produced by whole-cell of E. coli BL21/pETDuet-Kpdar-fdh. (a) Standard samples of pure stereoisomers; (b) sample from batch bioconversion by E. coli BL21/pETDuet-Kpdar-fdh; (c) sample from fed-batch bioconversion by E. coli BL21/pETDuet-Kpdar-fdh. Samples were extracted using ethyl acetate and isoamyl alcohol was used as the internal standard. | |
3.4 Fed-batch biotransformation
Diacetyl is toxic to recombinant E. coli,12,32 and therefore fed-batch processes should be performed to avoid substrate inhibition and achieve high product concentration.37 In this study, fed-batch biotransformation was conducted with an initial diacetyl concentration of 15.0 g L−1, while feeding small amounts of diacetyl and formate (ratio of formate to diacetyl approximately 1.2) at 1 h intervals. After 8.5 h of reaction, 52.9 g L−1 of (S)-acetoin was accumulated from 81.4 g L−1 of diacetyl with an enantiomeric purity of 99.5% (Fig. 3a and c) and productivity of 6.2 g (L h)−1 (Fig. 4). The titer and productivity were new records on high optical (S)-acetoin (enantiomeric purity ≥ 98.0%) production to our knowledge. In addition, 15.6 g L−1 of (2S,3S)-2,3-butanediol was obtained (Fig. 4). It should be pointed out that this optical active compound is also a building block in the chiral synthesis,20 and could be separated from (S)-acetoin by distillation for their different boiling points. No meso-2,3-butanediol, (2R,3R)-2,3-butanediol or organic acids were detected.
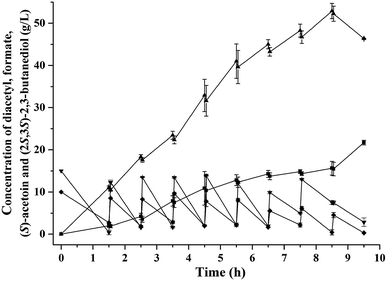 |
| Fig. 4 Production of optical (S)-acetoin by E. coli BL21/pETDuet-Kpdar-fdh in fed-batch bioconversion. (▲) (S)-Acetoin, (■) (2S,3S)-2,3-butanediol, (▼) diacetyl, (◆) formate. Error bars indicate standard deviations (n = 3). | |
According to Liu et al., (S)-acetoin was effectively produced by biocatalytic resolution of the mixture of meso-2,3-butanediol and (2S,3S)-2,3-butanediol by the resting cells of B. subtilis 168, in which meso-2,3-butanediol was converted to (S)-acetoin.38 However, the enantiomeric purity of the product (S)-acetoin was low (96.2%) for the generation of (R)-acetoin, which may be due to the complex metabolic network of B. subtilis. Gao et al. reported that whole-cells of recombinant E. coli over-expressing DAR could be used to produced relatively high concentration of (S)-acetoin (39.4 g L−1) from diacetyl.12 However, the enantioselective reduction of one diacetyl molecule to form (S)-acetoin consumes one NADH/NADPH molecule as an electron donor, while generating NAD+/NADP+.19,39 Once the DAR level is no longer limiting, the NADH/NADPH levels become limiting. Thus, it is particularly important to construct an efficient cofactor regeneration system to further increase the productivity.
In the present study, DARs from several species were codon-optimized and compared for (S)-acetoin synthesis, and two typical in situ-cofactor regeneration systems were introduced into recombinant E. coli. In batch biotransformation, co-expressing KpDAR with FDH resulted in 8.4-fold increase in titer, with enantiomeric purity of 99.6%. Compared with the results achieved previously using glucose as a co-substrate,12 co-expressing KpDAR with FDH improved the (S)-acetoin concentration from 39.4 g L−1 to 52.9 g L−1 and productivity from 2.0 g (L h)−1 to 6.2 g (L h)−1 in fed-batch biotransformation, indicating that the NADH level of E. coli was maintained appropriately by introduced FDH regeneration system. Thus, engineering of cofactor regeneration, together with a promising diacetyl reductase, can effectively improve the production of (S)-acetoin.
4. Conclusions
In this study, we developed an efficient and economical process for high-value chiral (S)-acetoin production from diacetyl. Two in situ-NADH regeneration systems were applied to improve the production of (S)-acetoin, and five DARs from several species were also compared for (S)-acetoin synthesis. Under the optimal conditions, 52.9 g L−1 of (S)-acetoin was produced with an enantiomeric purity of 99.5% and a productivity of 6.2 g (L h)−1; the titer and productivity were new records on high optical (S)-acetoin (enantiomeric purity ≥ 98.0%) production to our knowledge. The systematic approach developed in this study could also be applied to synthesize other optically active α-hydroxy ketones.
Conflicts of interest
There are no conflicts to declare.
Acknowledgements
This work was supported by the National Natural Science Foundation of China [grant numbers 21466007, 31460296 and 31360207]; and the Guangxi Science and Technology Development Project [grant number 14125008-2-22]. We appreciate the professional comments and the constructive suggestions of the anonymous reviewers and the editor in improving the manuscript.
References
- T. Tanaka, M. Kawase and S. Tani, Bioorg. Med. Chem., 2004, 12, 501–505 CrossRef PubMed.
- O. B. Wallace, D. W. Smith, M. S. Deshpande, C. Polson and K. M. Felsenstein, Bioorg. Med. Chem., 2003, 13, 1203–1206 CrossRef.
- Q. K. Fang, Z. X. Han, P. Grover, D. Kessler, C. H. Senanayake and S. A. Wald, Tetrahedron: Asymmetry, 2000, 11, 3659–3663 CrossRef.
- G. Song, T. Qin, H. Liu, G. B. Xu, Y. Y. Pan, F. X. Xiong, K. S. Gu, G. P. Sun and Z. D. Chen, Lung Cancer, 2010, 67, 227–231 CrossRef PubMed.
- X. A. Fu, M. X. Li, R. J. Knipp, M. H. Nantz and M. Bousamra, Cancer Med., 2014, 3, 174–181 CrossRef PubMed.
- T. A. Werpy and G. Petersen, Top value added chemicals from biomass: volume I - results of screening for potential candidates from sugars and synthesis gas, US Department of Energy, 2004 Search PubMed.
- Z. J. Xiao and J. R. Lu, Biotechnol. Adv., 2014, 32, 492–503 CrossRef PubMed.
- X. Ji, H. Huang and P. Ouyang, Biotechnol. Adv., 2011, 29, 351–364 CrossRef PubMed.
- T. W. Yang, Z. M. Rao, X. Zhang, M. J. Xu, Z. H. Xu and S. T. Yang, Crit. Rev. Biotechnol., 2017, 37, 990–1005 CrossRef PubMed.
- Q. Xu, L. Xie, Y. Li, H. Lin, S. Sun, X. Guan, K. Hu, Y. Shen and L. Zhang, J. Chem. Technol. Biotechnol., 2015, 90, 93–100 CrossRef.
- S. Saito, H. Inoue and K. Ohno, EP0409234, EP Patent, 1991.
- J. Gao, Y. Y. Xu, F. W. Li and G. Ding, Lett. Appl. Microbiol., 2013, 57, 274–281 CrossRef PubMed.
- Y. Peng and S. Z. Chen, Fine Chem. Intermed., 2002, 32, 20–21 Search PubMed.
- S. Ui, M. Odagiri, A. Mimura, H. Kanai, T. Kobayashi and T. Kudo, J. Ferment. Bioeng., 1996, 81, 386–389 CrossRef.
- Z. J. Xiao, P. H. Liu, J. Y. Qin and P. Xu, Appl. Microbiol. Biotechnol., 2007, 74, 61–68 CrossRef PubMed.
- Z. Z. Cui, Y. F. Mao, Y. J. Zhao, C. Chen, Y. J. Tang, T. Chen, H. W. Ma and Z. W. Wang, J. Chem. Technol. Biotechnol., 2018 DOI:10.1002/jctb.5702.
- J. Liu, C. Solem and P. R. Jensen, Biotechnol. Bioeng., 2016, 113, 2744–2748 CrossRef PubMed.
- S. Loschonsky, S. Waltzer, B. Volker and P. M. Müller, Chemcatchem, 2014, 6, 969–972 CrossRef.
- C. Gao, L. Zhang, Y. Xie, C. Hu, Y. Zhang, L. Li, Y. Wang, C. Ma and P. Xu, Bioresour. Technol., 2013, 137, 111–115 CrossRef PubMed.
- Y. He, F. Chen, M. Sun, H. Gao, Z. Guo, H. Lin, J. Chen, W. Jin, Y. Yang, L. Zhang and J. Yuan, Molecules, 2018, 23, 619–634 CrossRef PubMed.
- L. Li, Y. Wang, L. Zhang, C. Ma, A. Wang, F. Tao and P. Xu, Bioresour. Technol., 2012, 115, 111–116 CrossRef PubMed.
- S. Ui, A. Mimura, M. Ohkuma and T. Kudo, Lett. Appl. Microbiol., 1999, 28, 457–460 CrossRef PubMed.
- K. Goldberg, K. Schroer, S. Lütz and A. Liese, Appl. Microbiol. Biotechnol., 2007, 76, 249–255 CrossRef PubMed.
- K. Liang and C. R. Shen, Metab. Eng., 2017, 39, 181–191 CrossRef PubMed.
- Y. Wang, L. Li, C. Ma, C. Gao, F. Tao and P. Xu, Sci. Rep., 2013, 3, 2643 CrossRef PubMed.
- J. Sambrook and D. W. Russell, Molecular Cloning: A Laboratory Manual, Clod Spring Harbor Laboratory, 3rd edn, 2001 Search PubMed.
- Y. J. Zhou, W. Yang, L. Wang, Z. Zhu, S. Zhang and Z. K. Zhao, Microb. Cell Fact., 2013, 12, 1–12 CrossRef PubMed.
- S. J. Bae, S. Kim and J. S. Hahn, Sci. Rep., 2016, 6, 27667 CrossRef PubMed.
- L. Zhang, Y. Y. Xu, J. Gao, H. Xu, C. Cao, F. Xue, G. Ding and Y. Y. Peng, Bioresour. Technol., 2016, 201, 319–328 CrossRef PubMed.
- V. I. Tishkov and V. O. Popov, Biomol. Eng., 2006, 23, 89–110 CrossRef PubMed.
- N. Samuel, T. Bao, X. Zhang, T. W. Yang, M. J. Xu, X. Li, I. Komera, T. Philibert and Z. M. Rao, J. Chem. Technol. Biotechnol., 2017, 92, 2477–2487 CrossRef.
- R. Nina, N. Markus, L. Andreas, W. Roland, W. Andrea, E. Thorsten and H. Werner, Biotechnol. Bioeng., 2010, 106, 541–552 CrossRef PubMed.
- A. E. Serov, A. S. Popova, V. V. Fedorchuk and V. I. Tishkov, Biochem. J., 2002, 367, 841–847 CrossRef PubMed.
- J. Z. Lian, R. Chao and H. M. Zhao, Metab. Eng., 2014, 23, 92–99 CrossRef PubMed.
- L. Zhang, Y. Zhang, Q. Liu, L. Meng, M. Hu, M. Lv, K. Li, C. Gao, P. Xu and C. Ma, Sci. Rep., 2015, 5, 9033 CrossRef PubMed.
- K. Mädje, K. Schmölzer, B. Nidetzky and R. Kratzer, Microb. Cell Fact., 2012, 11, 1–8 CrossRef PubMed.
- N. Z. Xie, H. Liang, R. B. Huang and P. Xu, Biotechnol. Adv., 2014, 32, 615–622 CrossRef PubMed.
- Z. Liu, J. Qin, C. Gao, D. Hua, C. Ma, L. Li, Y. Wang and P. Xu, Bioresour. Technol., 2011, 102, 10741–10744 CrossRef PubMed.
- M. Takeda, S. Anamizu, S. Motomatsu, X. Chen and C. R. Thapa, Biosci. Biotechnol. Biochem., 2014, 78, 1879–1886 CrossRef PubMed.
Footnote |
† Electronic supplementary information (ESI) available. See DOI: 10.1039/c8ra06260a |
|
This journal is © The Royal Society of Chemistry 2018 |