DOI:
10.1039/C8RA05649H
(Paper)
RSC Adv., 2018,
8, 32016-32021
Partial denitrification coupled with immobilization of anammox in a continuous upflow reactor
Received
2nd July 2018
, Accepted 5th September 2018
First published on 14th September 2018
Abstract
Based on the stable operation of a continuous upflow reactor, immobilized anammox coupling with partial denitrification (DEAMOX), was successfully achieved after 94 days operation with a 63.5% accumulation rate of NO2−–N and a 98.4% removal rate of NO3−–N. Moreover, the findings show that the optimum range of COD/NO3−–N ratio for the coupling reaction was 2.3–2.7. The nitrogen removal performance of the coupling reactor decreased in response to the increase of pH value to 8.0 or 8.5, which was inconsistent with previously published results. Complete denitrification was successfully coupled with DEAMOX by adding polycaprolactone (PCL) as solid carbon source. As a result, the NO3−–N produced via anaerobic ammonium oxidation could be completely removed; the removal rate of total nitrogen increased from 80.3% to 88.5%. In addition, a large number of denitrifying biofilms were attached to the surface of PCL particles.
1. Introduction
Recently, the anaerobic ammonium oxidation technique (ANAMMOX) has increasingly attracted interest and environmentalists recognize it as the most sustainable wastewater denitrification technique.1–3 However, its independent use is limited since it requires nitrite nitrogen as an electron acceptor. At present, partial nitrification is the main route to obtain NO2−–N; however, it has high control requirements, is easily destroyed,4,5 and is difficult to recover once destroyed. Therefore, new approaches are urgently required. Kalyuzhnyi et al.6 proposed a new denitrification technology DEnitrifying AMmonium OXidation (DEAMOX) based on anaerobic ammonium oxidation and heterotrophic denitrification for the conversion of nitrate to nitrite. For DEAMOX, the anaerobic ammonium oxidation and denitrification reaction are conducted simultaneously in a single reactor. The electron acceptor of the anaerobic ammonium oxidation reaction, NO2−N, was sourced from denitrification. The denitrification reaction is complex and could be divided into four steps, NO3−–N → NO2−–N → NO → N2O → N2. If the reaction could be controlled at the first step, a high concentration of NO2−–N would be obtained. This technology has been named partial denitrification.7 Du et al.8 established the DEAMOX process by seeding denitrification sludge with high nitrite nitrogen, accumulated from a SBR reactor, and anammox granular sludge from an upflow anaerobic sludge blanket reactor. This mixture has been added to two single SBR reactors, with ethanol and sodium acetate as carbon sources, respectively, which were stably operated for 180 days. Li et al.9 seeded the bottom of a single continuous upflow reactor with partial-denitrification sludge from a continuous upflow reactor and loaded anammox sludge in the upper part, thus successfully achieving the coupling of partial denitrification and anammox. The reason why the above achieved this process was that they both had successfully acclimated denitrification sludge with high nitrite nitrogen accumulation, which was directly added into the reactor. However, they neglected the exploration about how to directly start up the DEAMOX process. Moreover, they obtained partial-denitrification sludge with specific methods, which was complicated.
In this study, based on the continuous upflow reactor of immobilized anammox that had been successfully started and stably operated, glucose was used as the sole carbon source to explore both the start-up and operation of the DEAMOX process in a continuous upflow reactor.10 The anammox coupling with partial denitrification was achieved by gradually increasing the concentration of NO3−–N in the influent while constantly adjusting the COD/NO3−–N ratio. During the start-up process, the anammox could obtain more electron acceptors (NO2−–N) due to the continuous improvement of the partial denitrification efficiency. Exogenous NO2−–N was gradually decreased until the electron acceptors NO2−–N of anammox could be completely provided by the partial denitrification process. The effect of the pH on the nitrogen removal efficiency of the coupling system was also investigated. Finally, a solid carbon source was added in the coupling terminal of the coupling process to further couple the whole denitrification and to remove the nitrate nitrogen produced via anaerobic ammonia oxidation, which promoted the removal rate of total nitrogen. The long-term performance of the combination of the partial denitrification, anammox, and the complete denitrification in a single reactor was investigated. In summary, a novel DEAMOX-complete denitrification process was established in this study for the simultaneous treatment of NO3−–N containing wastewater and NH4+–N containing sewage, as a cost-saving and efficient method.
2. Materials and methods
2.1 Synthetic wastewater
For continuous feeding tests, a synthetic medium was used. The synthetic medium contained (per liter): 0.5 g KHCO3; 0.0272 g KH2PO4; 0.3 g MgSO4; 0.18 g CaCl2; and 1 ml trace element solutions A and B. Trace element solution A contained (per liter): 5 g EDTA and 5 g FeSO4; trace element solution B contained (per liter): 15 g EDTA; 0.43 g ZnSO4·7H2O; 0.24 g CoCl2·6H2O; 0.99 g MnCl2·4H2O; 0.25 g CuSO4·5H2O; 0.22 g NaMoO4·2H2O; 0.19 g NiCl2·6H2O; 0.21 g NaSeO4·10H2O; and 0.014 g H3BO4. NH4+–N, NO2−–N, and NO3−–N were prepared via NH4Cl, NaNO2, and NaNO3, respectively.
2.2 Experimental procedure
2.2.1 Continuous apparatus. The experimental apparatus consisted of a continuous upflow reactor with a height of 1 m, an inner diameter of 90 mm, and an effective volume of 6 L (Fig. 1). The outer part was equipped with a water bath layer. The immobilized anaerobic ammonium oxidation particles were supported by a braided filler within flow-separated balls, and were evenly distributed in the reactor.
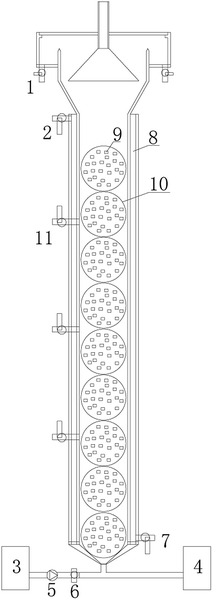 |
| Fig. 1 Partial denitrification coupled with immobilization of anammox reactor 1-outlet; 2-water jacket outlet; 3-influent (NH4+–N, NO2−–N, NO3−–N); 4-influent (COD); 5-peristaltic pump; 6-valve; 7-water jacket inlet; 8-water jacket; 9-immobilized pellets; 10-flow separated ball; 11-sampling outlet at different heights of reactor. | |
2.2.2 Start-up of the DEAMOX process. This study was based on a continuous upflow reactor of anaerobic ammonium-oxidizing bacteria, immobilized in a polyethylene glycol gel carrier, which had been successfully started up and operated stably.11 Both influent containing ammonium and nitrite at 30 and 45 mg-N L−1 were supplied to the reactor, respectively. The ratios of NO2−–N/NH4+–N and NO3−–N/NH4+–N in anammox process were about 1.25 and 0.25, which was close to the theoretical values of 1.32 and 0.26. On the 6th day, 10 mg L−1 NO3−–N was added to the influent and its impact on anaerobic ammonium oxidation was investigated. On the 11th day, the dosage of NO3−–N was increased to about 40 mg L−1 to match the need for partial denitrification coupling anammox. During phase IV, 10 mg L−1 COD was added to the influent to culture partial denitrifying bacteria. During phase V, the COD of influent increased to 60 mg L−1 to enhance the capacity of partial denitrification. During phase VI, the influent COD increased to 120 mg L−1. Furthermore, the concentration of NO2−–N in the influent decreased to 15 mg L−1 on the 50th day. During phase VIII, the concentration of NO2−–N in the influent was 0. During phase IX, the ratio of COD/NO3−–N was adjusted to explore the best conditions for coupling (Table 1).
Table 1 Operation mode of reactor
Phase |
Day |
Influent nitrogen concentration (mg L−1) |
COD concentration (mg L−1) |
COD/NO3−–N |
NH4+–N |
NO3−–N |
NO2−–N |
I |
0–5 |
30 |
— |
45 |
— |
— |
II |
6–10 |
30 |
13 |
45 |
— |
— |
III |
11–15 |
30 |
43 |
45 |
— |
— |
IV |
16–30 |
30 |
43 |
45 |
10 |
0.23 |
V |
31–41 |
30 |
43 |
45 |
60 |
1.39 |
VI |
42–49 |
30 |
43 |
45 |
110 |
2.56 |
VII |
50–56 |
30 |
43 |
15 |
110 |
2.56 |
VIII |
57–64 |
30 |
43 |
0 |
110 |
2.56 |
IX |
65–94 |
Adjust COD/NO3−–N ratio to explore the best conditions for coupling |
2.2.3 Increasing the pH to investigate the coupling performance. A previous study of anammox showed that the optimum pH range for anaerobic ammonium oxidation was 7.5–8.5.12 In this experiment, the pH of the influent was controlled to be 7.5 ± 0.1. Next, the pH was increased to 8.0 and 8.5 after a long-term operation for 94 days. In addition, the pH was increased to a reasonable range according to anammox. Its impact on the nitrite nitrogen accumulation of the partial denitrification coupling with immobilized anammox was explored.
2.2.4 Effect of PCL on the performance of DEAMOX process. A small amount of nitrate nitrogen was produced during the production stage of anaerobic ammonium oxidation, which influenced the contribution of anaerobic ammonium oxidation to the total nitrogen removal rate. Therefore, in this study, based on partial denitrification coupling and embedding anaerobic ammonium oxidation, about 100 g of the solid carbon source polycaprolactone (PCL) was added at the top of the continuous upflow reactor to remove the nitrate nitrogen produced by anammox.
2.3 Calculation method of nitrite nitrogen accumulation rate (NTR)
Eqn (1) is the anaerobic ammonium oxidation reaction equation. In theory, the consumption/production ratio of NH4+–N, NO2−–N, and NO3−–N is 1
:
1.32
:
0.26; the actual operation ratio was about 1
:
1.25
:
0.25. The parameters used in eqn (2) are the actual operating parameters. |
NH4+ + 1.32NO2− + 0.066HCO3− + 0.13H+ → 1.02N2 + 0.26NO3− + 0.066CH2O0.5N0.15 + 2.03H2O
| (1) |
|
 | (2) |
2.4 Analytical methods
The influent and effluent samples were collected on a daily basis and were analyzed immediately. All samples were analyzed after filtration through 0.45 μm pore size Millipore filter units. NH4+–N, NO2−–N, and NO3−–N were measured with a Lachat Quik Chem8500 Flow Injection Analyzer (Lachat Instruments, Milwaukee, USA). The pH and temperature were measured with the WTW 340i pH probe (WTW company, Germany). The total nitrogen (TN) concentration was calculated via the sum of ammonium nitrogen, nitrite nitrogen, and nitrate nitrogen concentration.
3. Results and discussion
3.1 Start-up of the DEAMOX process in the continuous reactor
The immobilizing method was used in the anammox reactor to evenly distribute the anaerobic ammonium oxidation bacteria in the reactor. Due to the long growth cycle of anaerobic ammonium oxidation bacteria,13 the immobilizing method could either decrease or completely avoid the loss of anammox sludge during operation.
Phase I was the stable operation period of the immobilized anaerobic ammonium oxidation. The hydraulic retention time (HRT) was 4 h, the operating temperature was maintained at about 30 °C, and the pH of the influent was controlled at 7.5 ± 0.1. These operating conditions remained unchanged during the start–up process. As shown in Fig. 2, the removal rates of NH4+–N and NO2−–N were 100% and 82.6%, respectively. The TN removal rate was 76.5%.
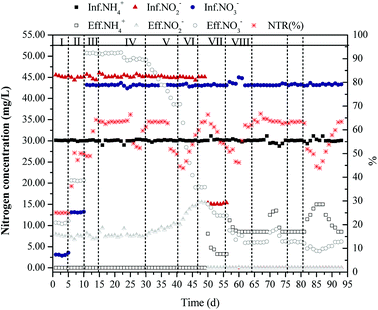 |
| Fig. 2 Start-up of partial denitrification coupled with immobilization of anammox in continuous upflow reactor. | |
During phase II, 10 mg L−1 and 40 mg L−1 NO3−–N were added to the influent, respectively. The addition of NO3−–N had no impact on the nitrogen removal rate of anaerobic ammonium oxidation bacteria or the reaction ratio of 1
:
1.25
:
0.25. After 16 days, to cultivate heterotrophic denitrifying bacteria, a small amount of COD (about 10 mg L−1) was added to the reactor and the COD/NO3−–N ratio was about 0.23. Under these conditions, denitrifying bacteria could freely distribute according to their growth needs. Due to the low biomass of the denitrifying bacteria in the reactor, the COD could not be effectively removed at the very beginning. Therefore, to reduce or completely avoid the influence of COD on anammox, the COD in the influent was controlled at a lower concentration. Previous studies showed that the addition of a small amount of COD did not impact the anaerobic ammonium oxidation.14–17 As shown in Fig. 2, the addition of COD did not impact anammox bacteria and the effluent nitrate concentration decreased slightly, indicating that the denitrifying bacteria gradually grew in the reactor. After 30 days of operation, the effluent nitrate concentration remained stable at about 49.12 mg L−1 and decreased by 1.71 mg L−1 compared to before addition of COD. Due to the lower amount of added COD, most COD was used to meet the growing needs of the microorganism. Therefore, the denitrification effect was not prominent and the NTR value was approximately 0.
During phase V, the dosage of COD increased to 60 and 110 mg L−1, with COD/NO3−–N ratios of approximately 1.39 and 2.56, respectively. During the phase, the denitrification efficiency continued to increase. During phase VI, the removal rate of NO3−–N reached 27.4% and 72.8%. On the 41st day, the NTR value slowly increased to 24.4%, indicating that a gradual increase of the partial denitrification efficiency. When the dosage of COD increased to 120 mg L−1, the removal rate of NO3−–N continued to improve; however, the NTR value did not increase significantly, indicating that the increase of COD did not enhance the partial denitrification efficiency and the increased COD caused the NO3−–N directly converting to N2. However, when the concentration of NO2−–N in the influent gradually decreased to 0, the NTR value increased significantly even if the dosage of COD remained unchanged. As shown in Fig. 2, on the 49th day, when the influent concentration of NO2−–N decreased to 15 mg L−1, the NTR value rapidly increased and stabilized at 48.9%. When the concentration of the influent NO2−–N decreased, part of electron acceptor required for anammox were provided via partial denitrification; therefore, the ability of anaerobic ammonium oxidation bacteria to compete with denitrifying bacteria for intermediate product of denitrification (NO2−–N) was enhanced. Moreover, an increasing amount of NO3−–N was reduced to NO2−–N rather than N2. However, the NTR value increased with the decreasing concentration of NO2−–N in the influent and partial denitrification was enhanced without changing other conditions. At this time, the removal rate of NO3−–N increased correspondingly, which stabilized at 87.0% on the 56th day. In theory, if 1 g NO3−–N was completely reduced to N2, about 2.86 g of BOD5 was required. In fact, the proportion was higher than this ratio because of various reasons. However, 1 g NO3−–N only required about 1.14 g BOD5 when it was reduced to NO2−–N instead of N2. Under the condition that the amount of the carbon source was identical and relatively insufficient, the higher the NTR value, the more NO3−–N could be reduced.
After 56 days, no nitrite was present in the influent and only NH4+–N, NO3−–N, and carbon sources were added to achieve partial denitrification coupling anammox without adding any nitrite. When the nitrite concentration was 0 in the influent, the NTR value and the removal rate of NO3−–N increased immediately, which kept at 63.5% and 98.4% on the 64th day, respectively.
After stable operation for a period of time, to determine the optimum range of COD/NO3−–N ratio, the influent concentration of COD was changed to adjust the COD/NO3−–N ratio of the influent, with the concentration of NH4+–N and NO3−–N in the influent remaining unchanged. On the 65th day, the influent COD/NO3−–N ratio decreased to around 2.3. The NTR value and the removal rate of NO3−–N remained unchanged. On the 70th day, both the NTR value and the removal rate of NO3−–N rapidly decreased to 52.2% and 88.1% when the COD/NO3−–N ratio decreased to 2.1, respectively. To prevent the deterioration of the operation state, the ratio was restored to 2.5 on the 73rd day. The nitrogen removal efficiency of the coupling reactor rapidly recovered to the original stable state after only 4 days. On the 76th day, the ratio increased to 2.7; however, the coupling efficiency was not decreased, indicating that the increase in COD concentration did not impact the effect of partial denitrification and that COD was in excess under this condition. On the 82nd day, the ratio continued to increase to 2.9. As shown in Fig. 2, the removal rate of NO3−–N still remained at a high level, while the NTR value rapidly decreased to about 45.0%. The nitrogen removal efficiency of the coupling reactor has been gradually recovered with a recovery time of seven days, since the COD/NO3−–N changed to 2.5 on the 88th day. Although the efficiencies of the reactor had all recovered rapidly, the recovery needed longer with higher COD/NO3−–N ratio than with lower COD/NO3−–N ratio.
3.2 Impact of increasing pH on the coupling system
According to the studies of Glass et al.18 and Li et al.,19 the increase of pH promotes the accumulation of nitrite nitrogen during the denitrification process. Glass et al. studied the accumulation of nitrite in denitrification process in sequencing batch reactors at pH values of 6.5, 7, 7.5, 8.5, and 9. When the pH was 6.5 and 7, the denitrification efficiency of nitrate was inhibited. However, the denitrification efficiency was significant when for pH values of 7.5, 8.5, and 9. With increasing influent pH, the NTR value continued to increase and the peaks of NO2−–N accumulation concentration reached 250 mg L−1, 500 mg L−1, and 900 mg L−1, respectively. Li et al. increased the pH value to about 9.2 via utilizing denitrification alkalization in the denitrification process with high nitrate nitrogen concentration (nitrogen load was about 55 kg N m−3 d−1) in the influent. The accumulation concentration of NO2−–N reached as high as 451.1 ± 49.0 mg L−1. Therefore, in this study, the pH of the influent increased from about 7.5 to 8.0 and 8.5, which was within the optimum range of the anaerobic ammonium oxidation reaction. The impact of pH on the coupling reactor was investigated. As shown in Fig. 3, from the 94th to the 109th day and from the 110th to the 119th day, the pH of the influent maintained at 8.0 and 8.5. The results indicate that the ammonia nitrogen removal rate and the NTR value of the coupling reactor decreased continuously in response to increasing the influent pH to 8.0; however, they tended to stabilize at the 102nd day, decreasing from 75.1% and 63.4% to 58.1% and 54.6%, respectively. After the 109th day, the further increase of pH in influent to 8.5 noticeably weakened the nitrogen removal efficiency of the coupling reactor. The NTR value and ammonia nitrogen removal rate decreased to 49.4% and 36.1%, respectively. The increased range of pH matched the optimum reaction conditions for anaerobic ammonium oxidation. Thus, the increase of pH observed in this study had little impact when anaerobic ammonium oxidation bacteria contended for electron acceptor, NO2−–N, it mainly affected the accumulation of NO2−–N in the denitrification process. The accumulation of NO2−–N decreased with increasing pH, which was not consisted with the study results of Glass and Li. The reason for this inconsistency was likely the different types of heterotrophic denitrifying bacteria cultivated. On the 120th day, the pH of the influent recovered to the initial state and the efficiency of coupling reactor also slowly recovered. After 11 days, the coupling reactor recovered to the optimal state and operated stably. Compared to changing the addition volume of the carbon source, the change of pH had a greater impact on the recovery of coupling efficiency and the recovery time was longer. However, due to the small increase of pH, the range of which was within the optimum range of the anaerobic ammonium oxidation reaction, the recovery time was relatively short.
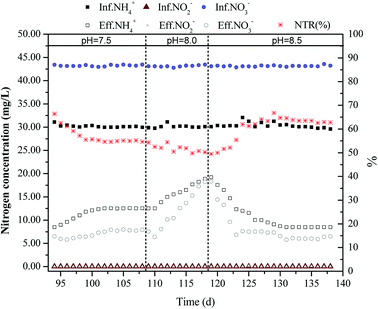 |
| Fig. 3 Effect of pH on partial denitrification coupled with immobilization of anammox. | |
3.3 Adding a solid carbon source (PCL) to increase the total nitrogen removal rate
PCL is a type of biodegradable polymer (BDPs) synthesized from petroleum as raw material and belongs to the aliphatic polyesters, which could be used as carbon source to effectively degrade nitrate nitrogen in wastewater. Furthermore, many studies have reported that PCL had good biodegradability and biocompatibility, and thus it is widely used in biological denitrification.20 PCL is characterized as a slow-release carbon source, which follows an easily controlled process, This characteristic was the premise of the practical application of PCL.21 In this study, PCL was used as a solid carbon source and about 100 g PCL particles were fixed on the upper part of the reactor by means of fine meshes, which were added on flow-separated balls to remove the nitrate nitrogen produced by anaerobic ammonia oxidation, thus increasing the total nitrogen removal rate of the reactor. Chu et al.22 used PCL as a biological denitrification fixed-bed carrier to remove the NO3−–N in the wastewater via denitrification. It was predicted that 1.6–3.7 g PCL should be consumed if 1 g NO3−–N was removed. Therefore, the NO3−–N in the effluent of coupling reactor was about 6.5 mg L−1 and the hydraulic retention time of the reactor (HRT) was about 4 h. Consequently, 100 g PCL particles can be used at least 100/(6.5 × 3.7 × 6 × 24/4/1000) = 116 days to 100/(6.5 × 1.6 × 6 × 24/4/1000) = 267 days until they were consumed completely. PCL was added after the reactor ran for 139 days. Fig. 4 shows the influent and effluent of the reactor after PCL addition. It could be seen that since the heterotrophic denitrifying bacteria in the reactor were mainly distributed in the lower part of the reactor, the capacity of bacteria to decompose PCL was poor. Therefore, a small amount of PCL could be transferred to the liquid phase at the very beginning. As a result of this poor denitrification efficiency, the concentration of NO3−–N in the effluent did not decrease significantly. After a period of operation, the denitrification efficiency increased rapidly. Since the denitrifying bacteria grew fast, the concentration of NO3−–N in the effluent was closed to 0 after 12 days. Since then, the reactor continued to operate stably. The addition of PCL contributed to the removal rate of the TN, which increased from 80.3% to 88.5%. Based on the DEAMOX process, the complete denitrification with PCL as solid carbon source could be successfully realized and stably operated. This also provided an effective method for the treatment of nitrogen wastewater. When the reactor operated for 156 days, a large number of denitrifying organisms adhered to the PCL particles. This result was consistent with most previous study results.23
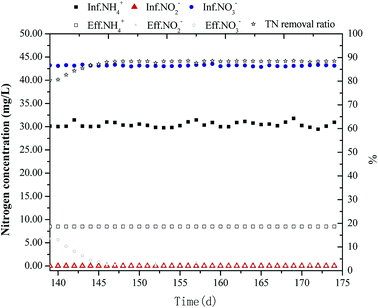 |
| Fig. 4 Changes of nitrogen concentration and TN removal rate in effluent with addition of solid carbon source. | |
4. Conclusion
The novel DEAMOX process for low C/N wastewater was successfully established based on a continuous flow reactor of immobilized anammox. The electron acceptor required for anammox was completely derived from the NO2−–N produced via partial denitrification. Appropriately increasing the pH of the influent to 8.0 and 8.5 decreased the coupling efficiency and the NTR value decreased to 49.4%. When the pH recovered to the initial state, the coupling efficiency recovered rapidly after 11 days. After only 12 days, the complete denitrification of the solid carbon source was successfully started up on the basis of the DEMOX process. The nitrate produced by the anaerobic ammonium oxidation reaction was completely removed, and the removal rate of TN increased from 80.3% to 88.5%.
Conflicts of interest
There are no conflicts to declare.
Acknowledgements
This research was supported by the National Major Project [2017ZX07103] and Natural Science Foundation of Beijing Municipality [8172012].
References
- W. Zhu, J. Li, H. Dong, D. Wang and P. Zhang, Biodegradation, 2017, 28, 437–452 CrossRef PubMed.
- L. Zhang, Y. Narita, L. Gao, M. Ali, M. Oshiki, S. Ishii and S. Okabe, Water Res., 2017, 125, 249–258 CrossRef PubMed.
- X. Tang, Y. Guo, B. Jiang and S. Liu, Water Res., 2018, 136, 95–103 CrossRef PubMed.
- A. Pedrouso, I. Aiartza, N. Morales, J. R. Vázquez-Padín, F. Rogalla, J. L. Campos, A. Mosquera-Corral and A. V. del Rio, Sep. Purif. Technol., 2018, 200, 94–101 CrossRef.
- J. Wu, Environ. Sci. Pollut. Res., 2017, 24, 25839–25848 CrossRef PubMed.
- S. Kalyuzhnyi, M. Gladchenko, A. Mulder and B. Versprille, Water Res., 2006, 40, 3637–3645 CrossRef PubMed.
- S. Cao, S. Wang, Y. Peng, C. Wu, R. Du, L. Gong and B. Ma, Bioresour. Technol., 2013, 149, 570–574 CrossRef PubMed.
- R. Du, S. Cao, B. Li, M. Niu, S. Wang and Y. Peng, Water Res., 2017, 108, 46–56 CrossRef PubMed.
- W. Li, Z. Y. Cai, Z. J. Duo, Y. F. Lu, K. X. Gao, G. Abbas, M. Zhang and P. Zheng, Chemosphere, 2017, 182, 532–538 CrossRef PubMed.
- S. Ge, Y. Peng, S. Wang, C. Lu, X. Cao and Y. Zhu, Bioresour. Technol., 2012, 114, 137–143 CrossRef PubMed.
- K. Isaka, Y. Date, T. Sumino and S. Tsuneda, Appl. Microbiol. Biotechnol., 2007, 76, 1457–1465 CrossRef PubMed.
- J. Li, W. Zhu, H. Dong and D. Wang, Biodegradation, 2017, 28, 245–259 CrossRef PubMed.
- M. Strous, J. J. Heijnen, J. G. Kuenen and M. S. M. Jetten, Appl. Microbiol. Biotechnol., 1998, 50, 589–596 CrossRef.
- S. Jenni, S. E. Vlaeminck, E. Morgenroth and K. M. Udert, Water Res., 2014, 49, 316–326 CrossRef PubMed.
- J. S. Guillén, Y. Yimman, C. L. Vazquez, D. Brdjanovic and J. B. Van Lier, Water Sci. Technol., 2014, 69, 2079–2084 CrossRef PubMed.
- C. Chen, X. Huang, C. Lei, T. C. Zhang and W. Wu, Bioresour. Technol., 2013, 148, 172–179 CrossRef PubMed.
- S. Q. Ni, J. Y. Ni, D. L. Hu and S. Sung, Bioresour. Technol., 2012, 110, 701–705 CrossRef PubMed.
- C. Glass and J. Silverstein, Water Res., 1998, 32, 831–839 CrossRef.
- W. Li, X. Y. Shan, Z. Y. Wang, X. Y. Lin, C. X. Li, C. Y. Cai, G. Abbas, M. Zhang, L. D. Shen, Z. Q. Hu and H. P. Zhao, Water Res., 2016, 88, 758–765 CrossRef PubMed.
- L. Chu and J. Wang, Chem. Eng. J., 2011, 170, 220–225 CrossRef.
- Y. Xu, T. L. Qiu, M. L. Han, J. Li and X. M. Wang, Procedia Environ. Sci., 2011, 10, 72–77 CrossRef.
- L. Chu and J. Wang, Chemosphere, 2013, 91, 1310–1316 CrossRef PubMed.
- W. Wu, L. Yang and J. Wang, Environ. Sci. Technol., 2013, 97, 2725–2733 Search PubMed.
|
This journal is © The Royal Society of Chemistry 2018 |