DOI:
10.1039/C8RA05047C
(Paper)
RSC Adv., 2018,
8, 30725-30731
Comparison of different pretreatments on the synergistic effect of cellulase and xylanase during the enzymatic hydrolysis of sugarcane bagasse
Received
12th June 2018
, Accepted 23rd August 2018
First published on 31st August 2018
Abstract
Sugarcane bagasse (SCB) substrates with different chemical compositions were prepared by different pretreatments including dilute acid (DA), acidic sodium chlorite (ASC), alkali solution (AS), and alkali hydrogen peroxide (AHP). The compositions and chemical structures of pretreated SCB were characterized by HPLC, FTIR, XRD, and SEM. The addition of xylanase can significantly boost cellulase to hydrolyze cellulose and xylan especially for AS and AHP treated substrates. The obvious linear relationships between lignin removal and substrate digestibility were observed. ASC treated substrates obtained the highest digestibility (98.87%) of cellulose due to sufficiently removing lignin from SCB, whereas AHP treated substrates achieved the highest digestibility (84.61%) of xylan by cleaving the acetyl group on xylan and extending delignification. It was found that the synergistic effects between cellulase and xylanase were substrate and time specific. The better degree of synergy for the sugar production was in the initial hydrolysis stage but decreased in the later hydrolysis stage.
1. Introduction
Lignocellulosic biomass is currently considered as a low cost and renewable source,1 which can be used to produce fuels and chemicals via chemical or physical pretreatment followed by enzymatic hydrolysis and microbial fermentation.2 The enzymatic hydrolysis process is the limiting step for the bioconversion of lignocellulosic biomass due to the complexity of its structure.3 To overcome the recalcitrant nature of biomass materials and enhance the enzymatic hydrolysis process, various pretreatment strategies have been developed already.4,5 In general, these pretreatments aim to change the physiochemical structure of lignocellulosic biomass to increase the accessibility of cellulase to their substrate.6 Among various pretreatments, dilute acid (DA) pretreatment is currently one of the most probable strategies for the commercialization of biofuel production from lignocellulosic biomass.7 In DA pretreatment, xylan is solubilized and converted into mainly xylose from lignocellulosic biomass. However, the retained lignin after DA pretreatment can strongly hinder enzyme accessibility to the cellulose for sugar production.8 Acidic sodium chlorite (ASC) pretreatment has been commonly used to prepare holocellulose, since it can selectively remove lignin from lignocellulosic biomass with minor effects on the structure of cellulose and xylan.9 Alkali solution (AS) pretreatment can efficiently break the crosslinking bonds between lignin and hemicellulose, which is an effective method to improve the enzymatic hydrolysis of lignocellulosic biomass.10 Alkali hydrogen peroxide (AHP) pretreatment can improve the digestibility of lignocellulosic biomass significantly because it not only removes lignin but also deconstructs the cell walls.11 Although DA, ASC, AP, and AHP show great potential for lignocellulosic biomass pretreatment, little study gives systematical comparison of these four different pretreatments.
In a typical lignocellulosic biomass, cellulose microfibrils are imbedded in xylan, which are highly cross-linked and taking shape a network by diferulic bridges.12 The addition of xylanase to degrade the xylan is an alternative approach to make cellulose more accessible to cellulase, and to obtain more sugars simultaneously.13 Therefore, the synergistic utilize of cellulase with xylanase has become an attractive approach for efficient hydrolysis of lignocellulosic biomass.14–16 Although many studies have been carried out on the synergistic hydrolysis of lignocellulosic biomass by cellulase and xylanse, the mechanism behind this synergistic effect during the hydrolysis of pretreated lignocellulosic biomass was still unclear, obviously preventing further development of this technology.17–20
In this work, sugarcane bagasse (SCB) substrates with different chemical compositions were prepared by different pretreatments including dilute acid (DA), acidic sodium chlorite (ASC), alkali solution (AS), and alkali hydrogen peroxide (AHP). The compositions and chemical structures of untreated and pretreated SCB were characterized by HPLC, FTIR, XRD, and SEM. Furthermore, the synergistic effects of commercial cellulase and xylanase during the hydrolysis of various pretreated SCB were investigated. By this study, valuable information about the pretreatment of lignocellulosic biomass and further enzymatic hydrolysis can be provided.
2. Experimental
2.1 Materials
SCB was purchased from a sugar factory in Yunnan Province, China. It was first dried in an oven at 80 °C, and then smashed into powder (40–80 mesh) for use. Cellulase complex (92.44 FPU mL−1) was obtained from Imperial Jade Bio-Technology Co., Ltd. (Yinchuan, China). Food grade xylanase (3310.70 IU g−1) was purchased from SUNSON Industry Group Co., Ltd (Beijing, China).
2.2 Pretreatment of SCB
For the preparation of DA treated substrates, the SCB was pretreated using 1% H2SO4 at 120 °C for 1 h with a solid to liquid ratio of 1
:
10 (g mL−1) in 0.5 L high pressure reactor. For the preparation of ASC treated substrates, the SCB was delignified with 0.6 g g−1 dry matter of sodium chlorite and 0.6 mL g−1 of dry matter of acetic acid at 70 °C for 4 h with a solid to liquid ratio of 1
:
32 (g mL−1) in 1 L flask. For the preparation of AS treated substrates, the SCB was pretreated using 1.5% NaOH at 70 °C for 4 h with a solid to liquid ratio of 1
:
20 (g mL−1) in 1 L flask. For the preparation of AHP treated substrates, the SCB was pretreated using 1.5% NaOH and 3% H2O2 at 70 °C for 4 h with a solid to liquid ratio of 1
:
20 (g mL−1) in 1 L flask. The pretreated SCB substrates were filtered to separate solids from liquids through a filtration, followed by washing with tap water until reaching neutral, and then the solid residue was dried at 80 °C for use.
2.3 Chemical characterization
The chemical composition of untreated and pretreated SCB was determined following the standard analytical procedure developed by National Renewable Energy Laboratory (NREL).21 The concentrations of the sugars in the acid and enzymatic hydrolysates were determined by HPLC using a Bio-Rad Aminex HPX-87H column (300 × 7.8 mm) and refractive index detector. The analytical column was eluted at 0.5 mL min−1 with 5 mM H2SO4, at 55 °C.
2.4 Structural characterization
FT-IR spectra of untreated and pretreated SCB samples were collected on a TENSOR 27 FT-IR spectrophotometer over the wavenumbers of 4000–400 cm−1 at a resolution of 4 cm−1. The crystallinity of untreated and pretreated SCB samples was measured by X-ray diffraction (XRD) using a PANalytical X'PertPro MPD diffractometer operating at 40 kV and 40 mA. The samples were scanned in a 2θ range from 5° to 80° at 2° min−1. The calculation of crystallinity index (CrI) was derived from the XRD spectra according to CrI = (I200 − Iam)/I200.22 The surface characteristics of untreated and pretreated SCB samples were observed by scanning electron microscope (S-4800, Hitachi, Japan) operated at 2.0 kV. The oven-dry samples were coated with platinum prior to SEM analysis.
2.5 Enzymatic hydrolysis
The enzymatic hydrolysis of the pretreated SCB samples (2%, w/v) was carried out in 150 mL Erlenmeyer flasks containing 50 mL citrate buffer solution (50 mM) with cellulase (25 IU g−1 dry substrates) and xylanase (400 IU g−1 dry substrates) at 50 °C on a rotary shaker set at 150 rpm. The hydrolysates were terminated by boiling in a water bath for 10 min, and then the samples were analyzed by HPLC. The digestibilities of substrates were calculated according to the following equations: |
 |
(1)
|
|
 |
(2)
|
where cglucose, ccellobiose and cxylose were the glucose, cellobiose and xylose concentration in hydrolysate, g L−1; csubstrate was the substrate loading, g L−1.
2.6 Degree of synergy
The calculation of the degree of synergy (DS) was determined according to the following equations: |
 | (3) |
where c1+2 is the sugar (glucose or xylose) concentration produced during hydrolysis when cellulase and xylanase are both added. c1 and c2 are the sugar (glucose or xylose) concentration produced, respectively, when cellulase and xylanase are added individually during hydrolysis.
3. Results and discussion
3.1 Chemical compositions of pretreated SCB
As shown in Table 1, the chemical compositions were different depending on various pretreatments. Four types of pretreated substrates were generated using DA, ASC, AS, and AHP pretreatments. Compared to the lignin content of raw material, the lignin content of DA treated substrates increased to 23.73%, whereas the lignin content of ASC, AS, and AHP treated substrates reduced to 1.78%, 8.85%, and 3.97%, respectively. The acetyl group of pretreated SCB from DA, AS, and AHP were completely removed, whereas the ASC pretreated SCB retained 81.34% of acetyl group in SCB. For DA pretreatment, the hemicellulose was hydrolyzed into xylose, arabinose, and acetic acid, whereas 96.73% of cellulose and 76.9% of lignin in SCB were still reserved. ASC selectively removed 93.99% of lignin and retained 97.04% of cellulose and 77.79% of xylan in SCB. AS removed 75.46% of lignin and 37.69% of xylan from SCB, whereas AHP removed 89.8% of lignin and 44.57% of hemicellulose from SCB.
Table 1 The effect of different pretreatment methods on chemical composition of SCB
Pretreatments |
Glucan (%) |
Xylan (%) |
Arabinan (%) |
Acetyl (%) |
Klason lignin (%) |
Solid recovery (%) |
Lignin removal (%) |
Untreated |
36.53 |
22.95 |
2.08 |
3.47 |
21.65 |
100.00 |
0 |
DA |
50.37 |
13.94 |
0.00 |
0.00 |
23.73 |
70.15 |
23.10 |
ASC |
48.49 |
24.42 |
2.41 |
3.86 |
1.78 |
73.1 |
93.98 |
AS |
57.78 |
23.82 |
1.97 |
0.00 |
8.85 |
60.03 |
75.46 |
AHP |
64.83 |
22.86 |
2.15 |
0.00 |
3.97 |
55.65 |
89.80 |
3.2 Structural characterization
The structural modifications of DA, ASC, AS, and AHP treated substrates were investigated by FT-IR (Fig. 1). The broad band at 3401 cm−1 was attributed to the stretching of OH group and that at 2904 cm−1 is due to the C–H stretching.23 The absorption at 1736 cm−1 and 1250 cm−1 is due to the stretching of C
O ester,23,24 indicating the presence of acetyl groups of hemicellulose. The absorption at 1510 cm−1 is resulted from C
C aromatic skeletal vibrations of lignin.23,24 The absorption at 1161 cm−1 and 897 cm−1 assigned to the C–O–C stretching at the β-glycosidic bond present in cellulose and xylan.23 The disappearance or weaken of the absorption at 1736 cm−1 and 1250 cm−1, which indicated that acetyl groups and part of hemicellulose were removed from SCB by DA, AS, and AHP pretreatment. Obviously, the ASC treated substrates preserved most of the acetyl groups of hemicellulose. The disappearance or weaken of the absorption at 1510 cm−1, indicates delignification of SCB by ASC, AS, and AHP pretreatment. However, this absorption is strong in DA treated substrates, suggested that DA pretreatment is ineffective for the removal of lignin. The results are in well agreement with the chemical compositions analysis.
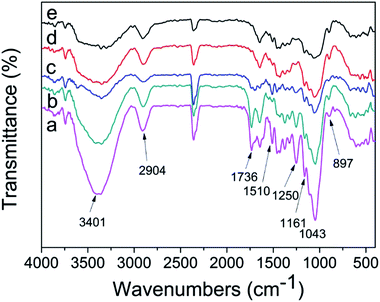 |
| Fig. 1 FT-IR spectrum of untreated (a), ASC (b), DA (c), AHP (d), and AS (e) pretreated SCB. | |
The crystalline structure and crystallinity index are believed to be influential factors affecting the enzymatic digestibility of lignocellulosic biomass.25 As shown in Fig. 2, the diffraction peaks at 2θ of 16.3°, 22.09° and 34.7° were attributed to the cellulose. The minor diffraction peaks at 26.64° and 29.4° were assigned to quartz (JCPDS, no. 65-0466) with SiO2 as the main ingredient and aragonite (JCPDS, no.47-1743) with CaCO3 as the main ingredient, respectively.26 The untreated and pretreated SCB exhibited a predominance of type I cellulose, suggesting that the crystalline structure of cellulose was not changed by these pretreatments. The crystallinity index indicates the relative amount of crystalline cellulose in the sample. The CrI of untreated SCB was 49.17%, and increased to 56.45%, 54.79%, 57.41%, and 57.91% after pretreated by DA, ASC, AS, and AHP respectively. The crystallinity index of DA treated substrates enhanced significantly due to the solubilization of amorphous hemicellulose. The crystallinity index of ASC treated substrates increased because of the oxidation of amorphous lignin. For AS and AHP treated substrates, the increased crystallinity index was due to the removal of both hemicellulose and lignin.
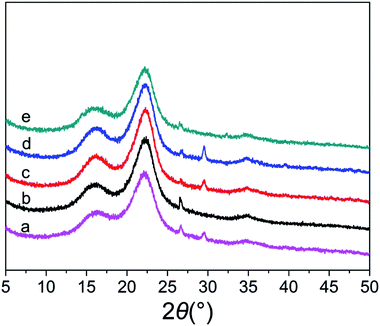 |
| Fig. 2 XRD spectrum of untreated (a), DA (b), AS (c), AHP (d), and ASC (e) pretreated SCB. | |
The surface morphology of untreated and pretreated SCB was observed by SEM (Fig. 3). The surface of SCB showed a rigid and highly ordered morphology structure. After DA pretreatment, the surface of the DA treated substrates exhibited pores, but retained the major features of raw material. After ASC pretreatment, the lignin layer was stripped and then the fibers were exposed. However, the surface of the ASC treated substrates was still intact, suggesting that the ASC had minor effect on cellulose and hemicellulose. The surface of AS treated substrates showed irregular cracks and roughness structure, whereas more pores and disrupted structure with exposed cellulose fibers were observed on surface of AHP treated substrates.
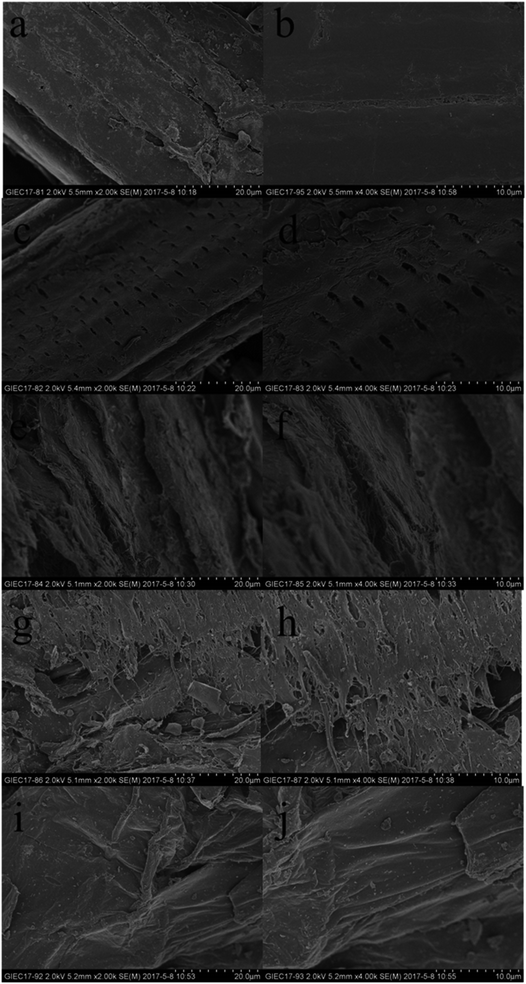 |
| Fig. 3 SEM analysis of untreated (a and b), DA (c and d), AS (e and f), AHP (g and h) and ASC (i and j) pretreated SCB. | |
3.3 Enzymatic hydrolysis of pretreated SCB
The enzymatic hydrolysis performance of the untreated and pretreated SCB is shown in Fig. 4. It was observed that only 13.43% of cellulose and 7.55% of xylan in the raw material were hydrolyzed after 96 h incubation. However, the enzymatic digestibility of cellulose and xylan of DA treated substrates increased to 19.30% and 14.86% after 96 h incubation due to the removal of xylan. The presence of lignin impedes the access of enzymes to cellulose and xylan.27 Thus, delignification process is always required before enzymatic hydrolysis. The digestibility of ASC treated substrates enhanced significantly due to the sufficiently removal of lignin. 97.47% of cellulose and 72.69% of xylan in the ACSP treated substrates were hydrolyzed after 96 h incubation. AS pretreatment can enhance the removal of lignin by breaking the ester bonds crosslinking the lignin and carbohydrates, thus, increasing the cellulose and xylan accessibility.6 73.8% of cellulose and 63.54% of xylan in the AS treated substrates were hydrolyzed after 96 h incubation. The supplementation of an oxidant agent to AS can improve its enzymatic performance significantly by deep removal of lignin.23 91.40% of cellulose and 75.83% of xylan in the AHP treated substrates were hydrolyzed after 96 h incubation.
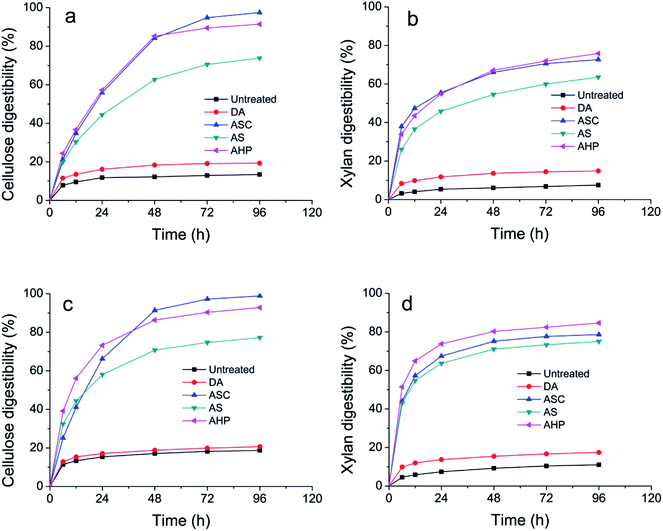 |
| Fig. 4 Enzymatic hydrolysis of untreated, DA, ASC, AS, and AHP pretreated SCB by cellulase (a and b) and cellulase with xylanase (c and d). | |
As shown in Fig. 4c and d, the addition of xylanase into cellulase could enhance hydrolysis of untreated and pretreated lignocellulosic biomass. Compare with enzymatic hydrolysis by cellulase, the digestibility of cellulose in the untreated, DA, ASC, AS, and AHP treated substrates increased by 45.73%, 11.23%, 19.29%, 62.47%, and 60.48%, respectively, after 6 h enzymatic hydrolysis by the cellulases and xylanases. Interestingly, the digestibility of cellulose in the untreated, DA, ASC, AS, and AHP treated substrates increased by 39.27%, 6.88%, 1.44%, 4.64%, and 1.56%, respectively, after 96 h enzymatic hydrolysis by the cellulases and xylanases. Furthermore, the digestibility of xylan in the untreated, DA, ASC, AS, and AHP treated substrates increased by 41.07%, 17.92%, 15.99%, 65.39%, and 51.80%, respectively, after 6 h enzymatic hydrolysis by the cellulases and xylanases, whereas the digestibility of xylan in the untreated, DA, ASC, AS, and AHP treated substrates increased by 46.12%, 17.20%, 8.12%, 18.09%, and 11.58%, respectively, after 96 h enzymatic hydrolysis by the cellulases and xylanases. These results indicate that the addition of xylanases can significantly boost cellulases to hydrolyze cellulose and xylan in the initial stage especially for AS and AHP treated substrates. However, these enhancement effects of the addition of xylanases decreased dramatically in the later stage of enzymatic hydrolysis for pretreated substrates. In addition, it was observed that the digestibility of cellulose was increased with the digestibility of xylan in the hydrolysis of SCB substrates.
The digestibility of cellulose of untreated, DA, ASC, AS, and AHP treated substrates by cellulase and xylanase were 18.70%, 20.62%, 98.87%, 77.22%, and 92.83%, respectively, after 96 h enzymatic hydrolysis. As shown in Fig. 5a, the linear relationships between lignin removal and cellulose digestibility suggested that delignification is essential for efficient enzymatic hydrolysis of cellulose. Lignin is a physical barrier that prevents cellulase and xylanase access to cellulose and xylan.28 The digestibility of xylan of untreated, DA, ASC, AS, and AHP treated substrates by cellulase and xylanase were 11.03%, 17.41%, 78.59%, 75.03%, and 84.61%, respectively, after 96 h enzymatic hydrolysis. As shown in Fig. 5b, the digestibility of xylan of pretreated substrates increased linearly with the removal of lignin except for ASC treated substrates in which xylan retained a considerable portion of side groups such as α-L-arabinofuranosyl and acetyl. The digestibility of xylan of ASC and AHP treated substrates by xylanase were 8.05% and 25.39%, respectively, after 96 h enzymatic hydrolysis, indicating that the xylan in ASC treated substrates were harder to be hydrolyzed than the xylan in AHP treated substrates. It is reported that the presence of side groups on xylan impeded the xylanase to hydrolysis of xylan29 and the removal of acetyl groups by AHP increased the access of xylanase to xylan. Therefore, it is reasonable that the xylan digestibility of AHP treated substrates was higher than the xylan digestibility of ASC treated substrates. Previous study showed that xylan isolated from SCB was substituted with α-L-arabinofuranoside and 4-O-methyl-α-D-glucuronic acid.30 The insufficient of xylan-degrading enzymes in cellulase, leading to the accumulation of substituted xylo-oligosaccharides, might be the reason why the digestibility of xylan was lower than the digestibility of cellulose for all pretreated substrates. Therefore, xylan-degrading enzymes such as α-L-arabinofuranosidases and α-glucuronidases were required for the completely hydrolysis of xylan in AHP treated substrates.
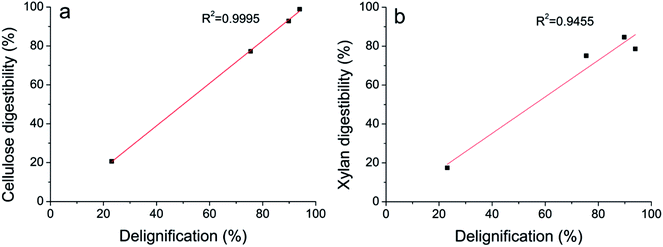 |
| Fig. 5 The relationships between delignification and digestibility of pretreated substrates. | |
The relationships between DS for sugar production and hydrolysis time is shown in Fig. 6. DS for the production of glucose decreased with the increased hydrolysis time when AHP treated substrates were hydrolyzed. DS for the production of glucose increased slightly and then decreased with the increased hydrolysis time when DA, AS, and ASC treated substrates were hydrolyzed. All DS for the production of glucose were greater than 1.0. DS for the production of xylose decreased with the increased hydrolysis time when AS and AHP treated substrates were hydrolyzed. DS for the production of xylose increased slightly and then decreased with the increased hydrolysis time when DA and ASC treated substrates were hydrolyzed. Interestingly, for AS and AHP treated substrates, DS for the production of xylose reduced to less than 1.0 after 48 h enzymatic hydrolysis. These results indicated that pretreatment methods and hydrolysis time have a significant impact on the synergistic hydrolysis of pretreated SCB by cellulases and xylanase. In the initial stage, the addition of xylanase contributed to the hydrolysis of cellulose by cellulase. Obviously, this critical step is beneficial for further large-scale application of enzymatic hydrolysis of lignocellulosic biomass in future.
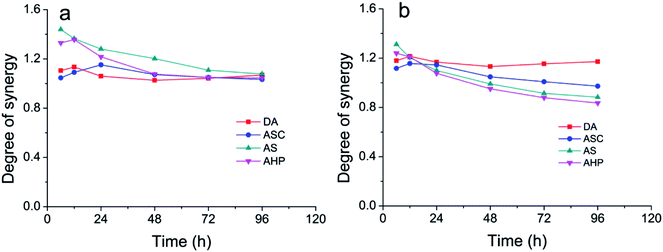 |
| Fig. 6 The DS of cellulase and xylanase during the enzymatic hydrolysis of pretreated substrates for the production of glucose (a) and xylose (b). | |
4. Conclusions
SCB substrates with different chemical compositions were prepared by different pretreatment methods. The obvious linear relationships between lignin removal and substrates digestibility indicated that delignification is essential for efficient enzymatic hydrolysis of both cellulose and xylan. Synergistic effect between cellulase and xylanase were substrate and time specific. The better DS for the sugar production was found in the initial stage but it decreased in the later hydrolysis stage. The highest digestibility of xylan from AHP treated substrates was only 84.61% suggested that xylan-degrading enzymes such as α-L-arabinofuranosidases and α-glucuronidases were required for the completely hydrolysis of xylan from pretreated substrates.
Conflicts of interest
There are no conflicts to declare.
Acknowledgements
This work was supported by the National Natural Science Foundation of China (31600475, 21606229), the Natural Science Foundation of Guangdong Province (2016A030310124, 2017A030310052), the project of Guangzhou Science and Technology (201707010241, 201610010014), the Key Research and Development Plan of Jiangsu Province (BE2016706, BE2016125), Special Support Project of Guangdong Province (2016TQ03N881), and Youth Innovation Promotion Association CAS (2015290).
References
- Y. Wang, S. De and N. Yan, Chem. Commun., 2016, 52, 6210–6224 RSC.
- S. Behera, R. Arora, N. Nandhagopal and S. Kumar, Renewable Sustainable Energy Rev., 2014, 36, 91–106 CrossRef.
- M. E. Himmel, S. Y. Ding, D. K. Johnson, W. S. Adney, M. R. Nimlos, J. W. Brady and T. D. Foust, Science, 2007, 315, 804–807 CrossRef PubMed.
- P. Alvira, E. Tomás-Pejó, M. Ballesteros and M. J. Negro, Bioresour. Technol., 2010, 101, 4851–4861 CrossRef PubMed.
- G. H. Yang, K. Y. Lum and J. Dong, Chempluschem, 2015, 80, 1565–1572 CrossRef.
- X. Meng and A. J. Ragauskas, Curr. Opin. Biotechnol., 2014, 27, 150–158 CrossRef PubMed.
- A. K. Mathew, B. Parameshwaran, R. K. Sukumaran and A. Pandey, Bioresour. Technol., 2016, 199, 13–20 CrossRef PubMed.
- H. Lou, M. Wang, H. Lai, X. Lin, M. Zhou, D. Yang and X. Qiu, Bioresour. Technol., 2013, 146, 478–484 CrossRef PubMed.
- S. Y. Ding and E. A. Bayer, Science, 2012, 338, 1055–1060 CrossRef PubMed.
- J. S. Kim, Y. Y. Lee and T. H. Kim, Bioresour. Technol., 2016, 199, 42–48 CrossRef PubMed.
- C. Alvarez-Vasco and X. Zhang, Biomass Bioenergy, 2017, 96, 96–102 CrossRef.
- B. C. Saha, J. Ind. Microbiol. Biotechnol., 2003, 30, 279–291 CrossRef PubMed.
- R. Kumar and C. E. Wyman, Bioresour. Technol., 2009, 100, 4203–4213 CrossRef PubMed.
- G. A. L. Gonçalves, Y. Takasugi, L. Jia, Y. Mori, S. Noda, T. Tanaka, H. Ichinose and N. Kamiya, Enzyme Microb. Technol., 2015, 72, 16–24 CrossRef PubMed.
- J. Hu, V. Arantes, A. Pribowo and J. N. Saddler, Biotechnol. Biofuels, 2013, 6, 112 CrossRef PubMed.
- Q. Qing and C. E. Wyman, Biotechnol. Biofuels, 2011, 4, 18 CrossRef PubMed.
- L. Jia, G. A. L. Gonçalves, Y. Takasugi, Y. Mori, S. Noda, T. Tanaka, H. Ichinose and N. Kamiya, Bioresour. Technol., 2015, 185, 158–164 CrossRef PubMed.
- S. Malgas, M. Thoresen, J. S. van Dyk and B. I. Pletschke, Enzyme Microb. Technol., 2017, 103, 1–11 CrossRef PubMed.
- H.-T. Song, Y. Gao, Y.-M. Yang, W.-J. Xiao, S.-H. Liu, W.-C. Xia, Z.-L. Liu, L. Yi and Z.-B. Jiang, Bioresour. Technol., 2016, 219, 710–715 CrossRef PubMed.
- J. Zhang and L. Viikari, Appl. Biochem. Biotechnol., 2014, 174, 1393–1402 CrossRef PubMed.
- A. Sluiter, B. Hames, R. Ruiz, C. Scarlata, J. Sluiter, D. Templeton and D. Crocker, Determination of structural carbohydrates and lignin in biomass, National Renewable Energy Laboratory, 2008 Search PubMed.
- L. Segal, J. J. Creely, A. E. Martin-Jr and C. M. Conrad, Text. Res. J., 1959, 29, 786–794 CrossRef.
- Q. Qing, L. Zhou, M. Huang, Q. Guo, Y. He, L. Wang and Y. Zhang, Bioresour. Technol., 2016, 201, 230–236 CrossRef PubMed.
- Y.-L. Loow, T. Y. Wu, G. H. Yang, L. Y. Ang, E. K. New, L. F. Siow, J. Md. Jahim, A. W. Mohammad and W. H. Teoh, Bioresour. Technol., 2018, 249, 818–825 CrossRef PubMed.
- H. Liu, B. Pang, Y. Zhao, J. Lu, Y. Han and H. Wang, Fuel, 2018, 221, 21–27 CrossRef.
- H. Guo, H. Zhang, F. Peng, H. Yang, L. Xiong, C. Wang, C. Huang, X. Chen and L. Ma, Appl. Catal., A, 2015, 503, 51–61 CrossRef.
- L. Kumar, V. Arantes, R. Chandra and J. Saddler, Bioresour. Technol., 2012, 103, 201–208 CrossRef PubMed.
- V. Arantes and J. N. Saddler, Biotechnol. Biofuels, 2010, 3, 4 CrossRef PubMed.
- H. Li, J. Wu, F. Jiang, Y. Xue, J. Liu, L. Gan, N. Ali and M. Long, J. Chem. Technol. Biotechnol., 2015, 90, 2083–2091 CrossRef.
- H. Li, H. Wu, L. Xiong, X. Chen, C. Wang, G. Qi, C. Huang, H. Guo, M. Luo, J. Liu, M. Long and X. Chen, Carbohydr. Polym., 2017, 175, 199–206 CrossRef PubMed.
|
This journal is © The Royal Society of Chemistry 2018 |
Click here to see how this site uses Cookies. View our privacy policy here.