DOI:
10.1039/C8RA04919J
(Paper)
RSC Adv., 2018,
8, 30430-30440
An efficient and environmentally sustainable domino protocol for the synthesis of structurally diverse spiroannulated pyrimidophenazines using erbium doped TiO2 nanoparticles as a recyclable and reusable heterogeneous acid catalyst†
Received
8th June 2018
, Accepted 22nd August 2018
First published on 28th August 2018
Abstract
An efficient and environmentally sustainable domino protocol has been presented for the synthesis of structurally diverse spiroannulated pyrimidophenazines involving a four component reaction of 2-hydroxynaphthalene-1,4-dione, benzene-1,2-diamine, cyclic ketones and amino derivatives in the presence of erbium doped TiO2 nanoparticles as a recyclable and reusable heterogeneous acid catalyst. The present synthetic protocol features mild reaction conditions with operational simplicity, excellent yield with high purity, short reaction time and high atom economy with the use of a recoverable and reusable environmentally sustainable heterogeneous catalyst.
Introduction
The synthesis of drug-like structurally diverse complex molecules with bio-relevance by energy efficient and environmentally sustainable synthetic strategies have attracted increasing interest in the field of organic syntheses and drug discovery research. Multicomponent reactions (MCRs) catalyzed by heterogeneous catalysts offer a green and sustainable strategy with high atom economy and low environmental factor (E-factor) due to the involvement of fewer reaction steps, simple workup, easy recovery, and reusability of the catalyst without isolation of intermediates.1–5 Moreover, multicomponent reactions provide easy and rapid access to large libraries of drug-like scaffolds incorporating medicinally privileged heterocyclic substructures.6,7 The nanostructured materials have attracted the attention of chemical research in view of their uses as heterogeneous catalysts in organic transformations, especially because they following the requirements of green chemistry.8,9 In recent years, significant advances have been made in the rational design and synthesis of highly active and selective nanostructured catalysts by controlling the structure and composition of the active nanoparticles (NPs) and by manipulating the interaction between the catalytically active NP species and their support.10–12 The catalytic efficiency, selectivity, and recyclability of nanostructured catalysts depend on the size, shape, composition, and assembly of the NPs, as well as their interaction with the support. The heterogeneous catalytic processes based on mixed metal oxide catalysts received considerable attention and was utilized successfully in organic transformations including heterocyclic synthesis and green synthetic protocols.13–15 The design and development of an efficient, green, sustainable and economical synthetic protocol is one of the major challenges in chemical research. The catalysis with nanostructured catalyst is considered as a significant option for multicomponent reactions in view of efficient and selective catalytic reactions with the waste reduction, atomic efficiency and catalyst recovery.16 Titanium dioxide (TiO2) catalysis is considered very close to an ideal catalysis because of its sustainability and environmental concerns.11,15,17–20 In recent years, TiO2 NPs have emerged as efficient and inexpensive heterogeneous catalyst to promote organic transformations.21
Phenazines are versatile building blocks and encountered in bioactive natural products (Fig. 1(i–vi)) and synthesized pharmaceuticals.22,23 Phenazines have been reported to exhibit a wide spectrum of biological activities including anticancer,24 (Fig. 1(iv)) antimalarial,25 (Fig. 1(v)) antiplasmodial,26 antibacterial,27 antifungal,28 cancer chemopreventive,29 antichagas agent30 and antiparasitic activities (Fig. 1),31 in addition to their uses in the preparation of industrial dyes, and fluorescent or electro-active markers in biological systems.32 Benzophenazines have shown dual inhibition of topoisomerase I and II, two key enzymes influencing DNA topology at different points in the cell cycle.24,33–36 The fluorescent phenazine derivatives have been considered as photosensitizers in photodynamic therapy (PDT)37–39 in which the combination of light and photosensitizer creates highly reactive oxygen species near the tumour to selectively destroy the targeted tissue.
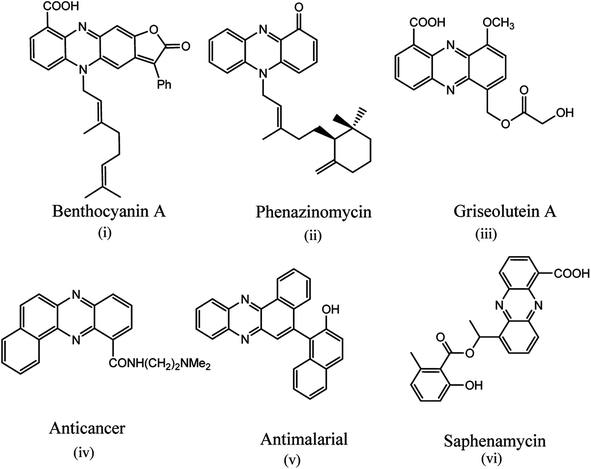 |
| Fig. 1 Biologically active phenazines. | |
Pyrimidine-fused heterocycles with wide range of biological activities are extensively being used in the design of new drugs.40 Pyrimido-pyrimidine derivatives have attracted considerable interest due to their tyrosine kinase inhibitory activity,41 antitumor activity,42 antiviral effect,43 and antioxidant properties.44 Pyridopyrimidines are also privileged scaffolds and reported to exhibit wide spectrum of activities including antitumor, antiallergic, antifolate, antimicrobial, calcium channel antagonist, antibacterial, antiinflammatory, analgesic, antihypertensive, antileishmanial, anticonvulsant, and diuretic.45–47 Pyridopyrimidine constitutes the core structure of some marketed drugs, including the antiasthmatic agent pemirolast,48 the tranquilizer pirenperone49 and the antiallergic agent barmastine.50
Quinoxaline derivatives have also been reported to exhibit a broad spectrum of biological activities, such as antibacterial,51,52 antifungal,53 antiviral,54 anticancer,55 anti-tubercular,53 antimalarial56 and anti-inflammatory54 (Fig. 2). Quinoxaline derivatives have also been reported to inhibit selectively the platelet-derived growth factor (PDGF) receptor kinase, PDGF-dependent DNA synthesis in cell lines and inhibit the human cancer cell lines.57
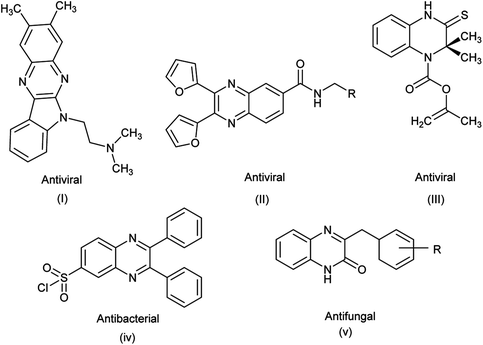 |
| Fig. 2 Biologically active quinoxalines. | |
In view of pharmaceutical importance of phenazines, pyrimidines and quinoxalines as medicinally privileged heterocyclic scaffolds and our continuing research interest in the synthesis of drug-like molecules with structural diversity and molecular complexity incorporating privileged structures,58–61 we are concerned with the development of an efficient and sustainable domino protocol for the synthesis of therapeutically interesting hybrid molecules, spiroannulated pyrimidophenazines, incorporating three-four medicinally privileged heterocyclic systems in a single molecule. The conventional synthetic strategies of phenazines usually involve multistep reaction sequences, which suffer from disadvantages including several synthetic steps, harsh reaction conditions, use of toxic organic solvents, high reaction time, low yields, and tedious isolation and purification of the resulting products.31 Multicomponent reactions (MCRs) with pot-economy are considered superior to the conventional multi-steps synthetic strategy, and offer a convenient approach for the rapid synthesis of complex molecules. Benzo[a]phenazines were synthesized by using p-TSA (10 mol%)/PEG-400 reaction medium via one-pot four-component protocol.38 Microwave-assisted MCRs have also been used for the synthesis of benzopyranophenazines.62–64 Benzo[a]pyrano[2,3-c]phenazines were also synthesized with the use of AcOH,65,66 caffeine,62 theophylline,67 DABCO,63,68 pyridine,69 PTSA,70 ionic-liquid,71 oxalic acid,72 bifunctional thiourea-based organocatalyst73 and nano-copper(II) oxide catalyzed MCRs. But it has been observed that the synthetic strategy to synthesize spirocyclic hybrid molecules with privileged heterocyclic substructures has not been reported in the literature. The present synthetic protocol is probably the first report for the synthesis of spiroannulated pyrimidophenazines, drug-like complex molecules, using nanostructured erbium (Er) doped TiO2 as heterogeneous solid acid catalyst.
Results and discussion
In the present work, we have presented highly efficient and diversity oriented sustainable synthetic protocol to synthesize spirocyclic hybrid molecules, pyrimidophenazines with privileged heterocyclic substructures, involving four component reaction of 2-hydroxynaphthalene-1,4-dione, benzene-1,2-diamine, cyclic ketone and amino derivatives in the presence of erbium doped TiO2 NPs as recyclable and reusable heterogeneous acid catalyst.
Preparation and characterization of catalyst
Er doped TiO2 NPs were synthesized by sol–gel method and characterized by X-ray diffraction (XRD) (X'pert pro-Panalytical, Cu-Kα, 1.54 Å), Fourier transform infrared spectroscopy (FTIR) (Perkin2Elmer 4000–400 cm−1), Transmission electron microscopy (TEM) (Technai T20 FEI), and Energy dispersive X-ray spectroscopy (EDX).
Fig. 3 shows XRD pattern of Er doped TiO2 (2%) NPs and confirms the crystalline behaviour of synthesized NPs. The peaks at the angle of 2θ = 25.25°, 37.80°, 47.79°, 54.12°, and 62.45° are corresponding to reflections from the planes (101), (004), (200), (105), and (213) respectively. All the planes correspond to the anatase phase of TiO2 and matched with the JCPDS card no. 00-021-1272. Absence of any peak other than TiO2 indicates the absence of any compound with Er and it is doped in TiO2 substitutionally or interstitially. The mismatching of the radii of the Er3+ and Ti4+ prevents the Er3+ ions to enter into the TiO2 lattice. So, the substitution of Ti4+ with Er3+ and the formation of Ti–O–Er bonds are expected which was further investigated by FTIR. The broadening and FWHM (full width at half maximum) of the peaks suggests the size of NPs in the range of nanoscale and the particles size was calculated by Debye–Scherrer formula.
where,
d represents the size of the NPs.
λ,
β, and
θ are the wavelength of X-rays incident on the sample, FWHM and angle of diffraction respectively. The average particle size was calculated as ∼7.5 nm.
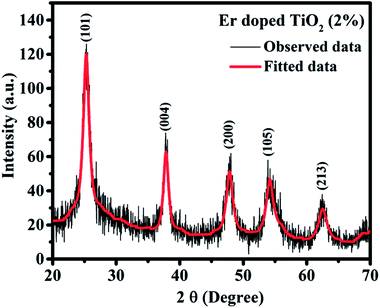 |
| Fig. 3 XRD pattern of Er doped TiO2 (2%) NPs. | |
FTIR spectrum of Er doped TiO2 NPs is shown in Fig. 4. The bands observed from 400 to 1000 cm−1 correspond to the lattice vibrations of Ti–O, and Ti–O–Ti in TiO2 lattice. The vibrations corresponding to Ti–O–Er bonds also occur in the same region. The presence of Er was further confirmed by EDX. The peak at 1071 cm−1 may be assigned to the bond S–O which is expected to occur from thioglycerol used as capping agent. The bands observed from 1200–1800 cm−1 and a broad band at 3235 cm−1 may be ascribed to the bending vibrations of water molecules adsorbed on the surface of NPs and the stretching vibrations of hydroxyl ions (O–H).
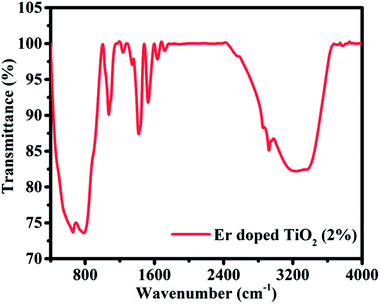 |
| Fig. 4 FTIR spectra of Er doped TiO2 (2%) NPs. | |
Morphological behaviour of NPs was examined by TEM and shown in Fig. 5. The shape of the NPs from Fig. 5(a) seems to be spherical however the NPs are found to be agglomerated. The size of the NPs from TEM image is consistent with the XRD results. Inset of Fig. 5(a) shows the SAED pattern of the NPs consisting of a number of rings. These rings indicate the polycrystalline behaviour of the NPs. From the analysis of HRTEM image (Fig. 5(b)) of NPs, d spacing of (101) plane was found to be 3.57 Å. Inset of Fig. 5(b) shows the inverse FFT and the profile of inverse FFT to calculate the d spacing. The presence of Er in Er doped TiO2 NPs was confirmed by EDX spectra shown in Fig. 5(c).
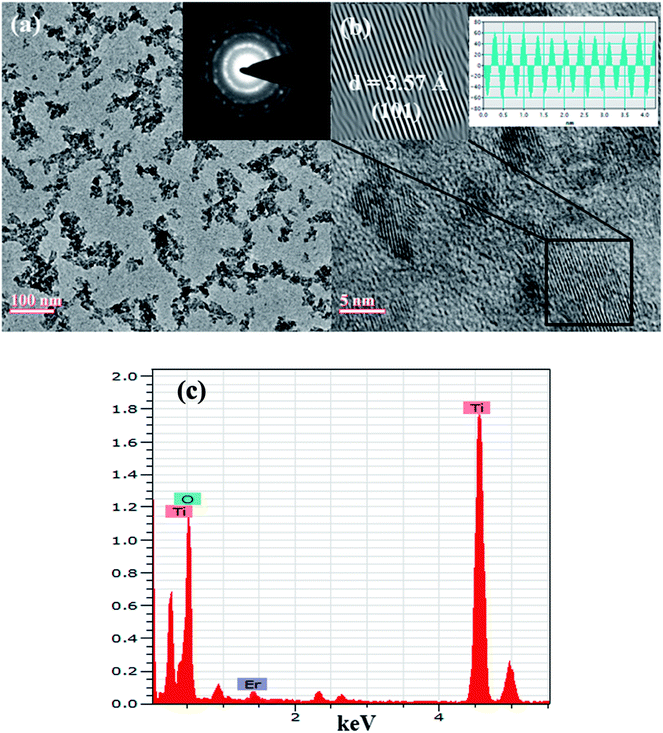 |
| Fig. 5 TEM image, SAED pattern (inset) (a), HRTEM image of lattice planes, IFFT and profile of IFFT (b), and EDX (c) of Er doped TiO2 (2%) NPs. | |
Optimization of reaction conditions
Initially, four-component reaction of 2-hydroxynaphthalene-1,4-dione (1), benzene-1,2-diamine (2), N-methyl-4-piperidone (3a) and 2-aminopyridine (4a) was selected as a simple model reaction to establish the feasibility of the present synthetic strategy and to optimize the reaction conditions (Scheme 1).
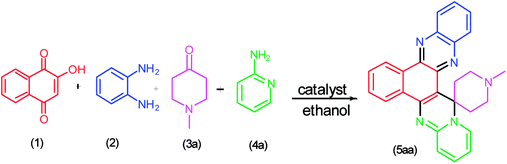 |
| Scheme 1 Model reaction. | |
The multicomponent reaction was carried out under catalyst and solvent free conditions, but the reaction was not successful and did not provide the desired product (no result) (Table 1, entry 1). We have used different solvents in the reaction, but ethanol was found to be the solvent of choice (Table 1, entries 2–7). The model reaction was also performed in the presence of InCl3 and FeCl3 in ethanol as solvent, but the desired product was obtained in moderate yield (Table 1, entries 8 and 9). The reaction was then screened with ZnO, ZrO and TiO2 nanocatalysts using ethanol as solvent and observed that the reaction catalyzed by TiO2 NPs provided comparatively better result as compared with those obtained with the use of ZnO and ZrO nanocatalysts (Table 1, entry 10–12). But when the reaction was carried out with the use of Er doped TiO2 NPs in the presence of ethanol, the encouraging results were obtained (Table 1, entry 13–21). Moreover, the effect on catalyst activity with the range of Er doping with TiO2 NPs was also investigated. To find out the ideal doping of erbium on TiO2 NPs with reference to catalyst activity, the reactions with 0.5%, 1%, 1.5%, 2%, 5%, 7%, and 10% erbium doped TiO2 were performed under similar conditions and observed that 0.5%, 1% and 1.5% Er doped TiO2 nanocatalysts provided 82%, 86% and 91% yields respectively in 20 minutes (Table 1, entry 13–15). As evident from the reaction results, 2% erbium doping provided the best result with 95% yield in 20 minutes (Table 1, entry 16), while erbium doping (5%, 7% and 10%) provided slightly decreased yield (91–87%) (Table 1, entry 17–19). The effect of catalyst loading on efficiency on catalyst was also examined and observed that 25 mg loading of erbium doped TiO2 NPs was optimal and provided excellent yield of the product (Table 1, entry 20 and 21). The higher loading of catalyst did not noticeably improve the yield. Thus, with the optimized reaction conditions, we explored the generality and scope of the present synthetic strategy with different building blocks and synthesized the library of spiroannulated pyrimidophenazines. To our delight, the reactions proceed smoothly and structurally diverse spiroannulated pyrimidophenazines were obtained in excellent yields. The results are summarized in Tables 2–4.
Table 1 Optimization of reaction conditionsa,b
Entry |
Catalyst (mol%) |
Solventc |
Time |
Yieldd (%) |
Bold row indicates the optimization condition for the reaction. 2-Hydroxynaphthalene-1,4-dione (1 mmol), benzene-1,2-diamine (1 mmol), N-methyl-4-piperidone (1 mmol) and 2-aminopyridine (1 mmol) were stirred with refluxing till completion of the reaction as indicated by TLC. Solvents (2.0 ml). Isolated yield after purification. |
1 |
Catalyst free |
None |
12 h |
NR |
2 |
Catalyst free |
CH2Cl2 |
8 h |
NR |
3 |
Catalyst free |
CHCl3 |
8 h |
NR |
4 |
Catalyst free |
1,4-Dioxane |
6 h |
10% |
5 |
Catalyst free |
Methanol |
6 h |
28% |
6 |
Catalyst free |
Ethanol |
6 h |
35% |
7 |
Catalyst free |
Water |
6 h |
23% |
8 |
InCl3 (10 mol%) |
Ethanol |
3 h |
53% |
9 |
FeCl3 (10 mol%) |
Ethanol |
3 h |
55% |
10 |
ZnO NPs (10 mol%) |
Ethanol |
1 h |
60% |
11 |
ZrO NPs (10 mol%) |
Ethanol |
1 h |
72% |
12 |
TiO2 NPs (10 mol%) |
Ethanol |
1 h |
78% |
13 |
0.5% Er doped TiO2 NPs (25 mg) |
Ethanol |
20 min |
82% |
14 |
1% Er doped TiO2 NPs (25 mg) |
Ethanol |
20 min |
86% |
15 |
1.5% Er doped TiO2 NPs (25 mg) |
Ethanol |
20 min |
91% |
16 |
2% Er doped TiO2 NPs (25 mg) |
Ethanol |
20 min |
95% |
17 |
5% Er doped TiO2 NPs (25 mg) |
Ethanol |
20 min |
91% |
18 |
7% Er doped TiO2 NPs (25 mg) |
Ethanol |
20 min |
87% |
19 |
10% Er doped TiO2 NPs (25 mg) |
Ethanol |
20 min |
87% |
20 |
2% Er doped TiO2 NPs (15 mg) |
Ethanol |
20 min |
92% |
21 |
2% Er doped TiO2 NPs (30 mg) |
Ethanol |
20 min |
95% |
Table 2 Synthesis of spiroannulated pyrimidophenazines
Table 3 Synthesis of spiroannulated pyrimidophenazines
Table 4 Synthesis of spiroannulated pyrimidophenazines
Additionally, the catalytic recyclability and reusability was also investigated on the model reaction under optimized reaction conditions. After each cycle, the reaction was followed by extraction of products and catalyst. The collected catalyst was washed with methanol for several times to remove organic substances and reused for the next run. The performance of the recycled catalyst was tested up to five successive runs and observed that the recovered catalyst could be used for five times without an appreciable loss of its activity (Fig. 6).
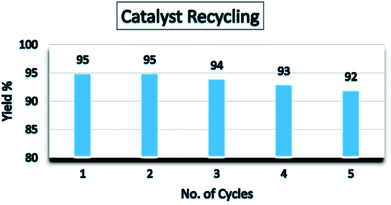 |
| Fig. 6 Recyclability and reusability of erbium doped TiO2 NPs. | |
Mechanism
The mechanism of the reaction probably proceed with the following steps involving the Michael addition, cyclization and dehydration as presented in Scheme 2 (5aa). The doping of erbium with TiO2 NPs increased the efficiency of the resulting catalyst and thus facilitated the reaction in better way as compared with TiO2 NPs (Scheme 2).
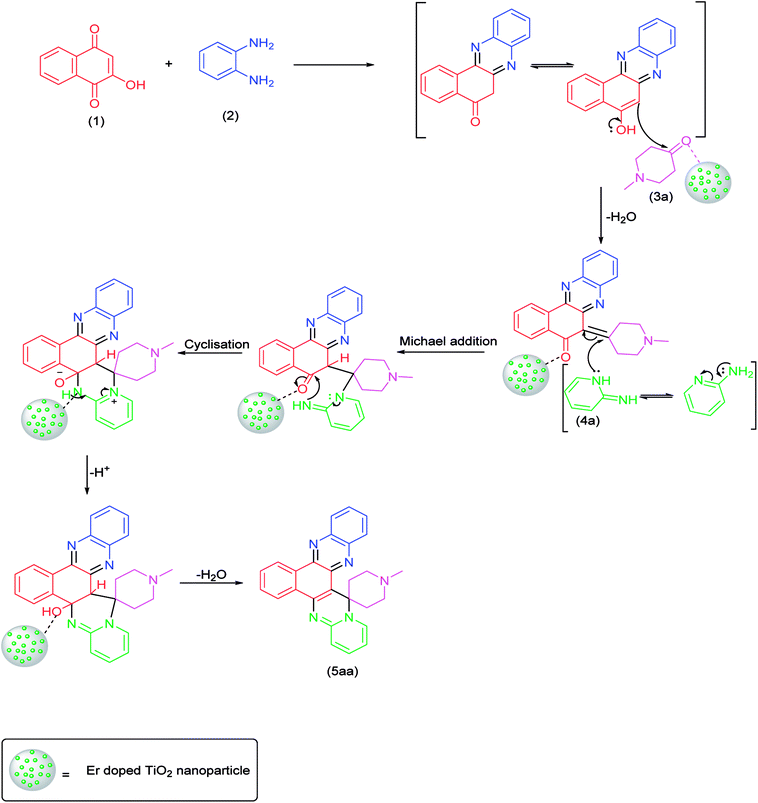 |
| Scheme 2 Proposed mechanism. | |
Experimental
General procedure
The melting points of all the synthesized compounds were determined on electric melting point apparatus and are uncorrected. 2-Hydroxynaphthalene-1,4-dione, benzene-1,2-diamine, cyclic ketones and amino derivatives used in the synthesis of complex spiroheterocycles were purchased from the commercial sources and were used as such. The purity of all the synthesized compounds was checked by TLC. 1H NMR and 13C NMR were recorded on JEOL 400 MHz and 100 MHz NMR spectrometer, respectively. Analytical and spectral data of the synthesized heterocycles are also included.
Typical procedure for synthesis of Er doped TiO2 NPs
Er doped TiO2 NPs were synthesized by sol–gel route using titanium tetra isopropoxide (TTIP) as a precursor. Firstly, water, ethanol, and acetic acid was mixed in a ratio of 2
:
20
:
3 respectively and stirred for 15 min. TTIP (5 ml) was dissolved in the prepared solution and the stirring was continued for half an hour. A separate solution of ErCl3 in water and ethanol (1
:
10) was prepared and added to the TTIP solution. The resultant solution was stirred for half an hour. 1-Thioglycerol (0.25 ml) was added drop-wise and the stirring was further continued for next one hour. Resulted milky white sol was placed in a water bath at 80 °C for two and half hour and an orange gel was formed. Subsequently, the gel was washed with deionised water several times to eradicate the alcohol and dried in oven at 100 °C. After cooling to room temperature the dried gel was grinded and the powder was post-annealed in air at the temperature 500 °C for one hour. The amount of ErCl3 was varied to synthesize Er doped TiO2 with different concentration.
Typical procedure for synthesis of spiroheterocycles
A mixture of 2-hydroxynaphthalene-1,4-dione (1 mmol), bezene-1,2-diamine (1 mmol), cyclic carbonyl compounds (1 mmol), amino derivatives (1 mmol) and Er doped TiO2 NPs (25 mg) in 2.0 ml ethanol was refluxed for 20 min. After the completion of the reaction (monitored by TLC), the catalyst was recovered by filtration, washed with ethanol, dried at room temperature and reused directly for a fresh reaction mixture up to five reaction cycle. The solid compound was purified by recrystallization from absolute ethanol without using any column chromatography.
The analytical and spectral data of the synthesized compounds have been presented and included in the ESI section.†
Conclusion
In conclusion, we have presented highly efficient and diversity oriented sustainable domino protocol to synthesize spiroannulated pyrimidophenazines, hybrid molecules with privileged heterocyclic substructures, involving four component reaction of 2-hydroxynaphthalene-1,4-dione, benzene-1,2-diamine, cyclic ketones and amino derivatives in the presence of erbium doped TiO2 NPs as recyclable and reusable heterogeneous acid catalyst. To the best of our knowledge, this is the first report where erbium doped TiO2 has been prepared and used as a heterogeneous solid acid catalyst first time for the synthesis of drug-like complex molecules incorporating medicinally privileged heterocyclic structures. The doping of TiO2 with erbium influences the efficiency of TiO2 as the catalyst and facilitates the reaction to provide comparatively better yields of the products. The present synthetic protocol offers several advantages including operational simplicity with easy work up, excellent yield with high purity, short reaction times and use of recoverable and reusable environmentally sustainable heterogeneous catalyst. The present synthetic protocol will provide an attractive synthetic methodology for the synthesis of structurally diverse drug-like small molecules for medicinal chemistry and drug discovery research.
Conflicts of interest
There are no conflicts to declare.
Acknowledgements
We gratefully acknowledge UGC New Delhi for the award of Research Fellowships to Kanchan (SRF) and Esha (JRF). UGC Bhopal is also acknowledged for financial support. Head, Department of Chemistry is acknowledged for providing Lab and instrumental facilities in the department.
References
- S. N. Maddila, S. Maddila, W. E. van Zyl and S. B. Jonnalagadda, RSC Adv., 2015, 5, 37360–37366 RSC.
- U. Chinna Rajesh, U. Gulati and D. S. Rawat, ACS Sustainable Chem. Eng., 2016, 4, 3409–3419 CrossRef.
- B. H. Rotstein, S. Zaretsky, V. Rai and A. K. Yudin, Chem. Rev., 2014, 114, 8323–8359 CrossRef PubMed.
- Z. C. Zhang, B. Xu and X. Wang, Chem. Soc. Rev., 2014, 43, 7870–7886 RSC.
- S. K. Kundu and A. Bhaumik, RSC Adv., 2015, 5, 32730–32739 RSC.
- S. Michael Rajesh, B. D. Bala, S. Perumal and J. C. Menendez, Green Chem., 2011, 13, 3248–3254 RSC.
- H. Naeimi, Z. Rashid, A. H. Zarnani and R. Ghahremanzadeh, New J. Chem., 2014, 38, 348–357 RSC.
- M. B. Gawande, P. S. Branco and R. S. Varma, Chem. Soc. Rev., 2013, 42, 3371–3393 RSC.
- B. Karami, M. Kiani, S. J. Hosseini and M. Bahrami, New J. Chem., 2015, 39, 8576–8581 RSC.
- A. Molla and S. Hussain, RSC Adv., 2016, 6, 5491–5502 RSC.
- G. B. Dharma Rao, S. Nagakalyan and G. K. Prasad, RSC Adv., 2017, 7, 3611–3616 RSC.
- P. Sagar Vijay Kumar, L. Suresh, T. Vinodkumar, B. M. Reddy and G. V. P. Chandramouli, ACS Sustainable Chem. Eng., 2016, 4, 2376–2386 CrossRef.
- S. N. Maddila, S. Maddila, W. E. van Zyl and S. B. Jonnalagadda, ChemistryOpen, 2016, 5, 38–42 CrossRef PubMed.
- A. Amoozadeh, S. Rahmani, M. Bitaraf, F. B. Abadi and E. Tabrizian, New J. Chem., 2016, 40, 770–780 RSC.
- C.-H. Kuo, A. S. Poyraz, L. Jin, Y. Meng, L. Pahalagedara, S.-Y. Chen, D. A. Kriz, C. Guild, A. Gudz and S. L. Suib, Green Chem., 2014, 16, 785–791 RSC.
- Z. Zarei and B. Akhlaghinia, New J. Chem., 2017, 41, 15485–15500 RSC.
- I. Pugazhenthi, S. M. Ghouse, F.-R. Nawaz Khan, E. D. Jeong, J. S. Bae, J.-P. Kim, E. H. Chung, Y. S. Kumar and C. Dasaradhan, RSC Adv., 2015, 5, 17257–17268 RSC.
- A. Khazaei, F. Gholami, V. Khakyzadeh, A. R. Moosavi-Zare and J. Afsar, RSC Adv., 2015, 5, 14305–14310 RSC.
- E. Ubba, F.-R. Nawaz Khan, E. D. Jeong and E. H. Chung, RSC Adv., 2014, 4, 57016–57025 RSC.
- S. Rana, M. Brown, A. Dutta, A. Bhaumik and C. Mukhopadhyay, Tetrahedron Lett., 2013, 54, 1371–1379 CrossRef.
- Y. K. Tailor, S. Khandelwal, Y. Kumari, K. Awasthi and M. Kumar, RSC Adv., 2015, 5, 46415–46422 RSC.
- S. Kumar, R. K. Saunthwal, M. Mujahid, T. Aggarwal and A. K. Verma, J. Org. Chem., 2016, 81, 9912–9923 CrossRef PubMed.
- S. Kumar, M. Mujahid and A. K. Verma, Org. Biomol. Chem., 2017, 15, 4686–4696 RSC.
- N. Vicker, L. Burgess, I. S. Chuckowree, R. Dodd, A. J. Folkes, D. J. Hardick, T. C. Hancox, W. Miller, J. Milton, S. Sohal, S. Wang, S. P. Wren, P. A. Charlton, W. Dangerfield, C. Liddle, P. Mistry, A. J. Stewart and W. A. Denny, J. Med. Chem., 2002, 45, 721–739 CrossRef PubMed.
- H. Hussain, S. Specht, S. R. Sarite, M. Saeftel, A. Hoerauf, B. Schulz and K. Krohn, J. Med. Chem., 2011, 54, 4913–4917 CrossRef PubMed.
- M. E. Makgatho, R. Anderson., J. F. O'Sullivan, T. J. Egan, J. A. Freese, N. Cornelius and C. E. J. V. Rensburg, Drug Devel. Res., 2000, 50, 195–202 CrossRef.
- N. V. Borrero, F. Bai, C. Perez, B. Q. Duong, J. R. Rocca, S. Jin and R. W. Huigens III, Org. Biomol. Chem., 2014, 12, 881–886 RSC.
- J. R. Kerr, G. W. Taylor, A. Rutman, N. Høiby, P. J. Cole and R. Wilson, J. Clin. Pathol., 1999, 52, 385 CrossRef PubMed.
- M. Conda-Sheridan, L. Marler, E.-J. Park, T. P. Kondratyuk, K. Jermihov, A. D. Mesecar, J. M. Pezzuto, R. N. Asolkar, W. Fenical and M. Cushman, J. Med. Chem., 2010, 53, 8688–8699 CrossRef PubMed.
- C. Neves-Pinto, V. R. S. Malta, M. d. C. F. R. Pinto, R. H. A. Santos, S. L. de Castro and A. V. Pinto, J. Med. Chem., 2002, 45, 2112–2115 CrossRef PubMed.
- N. Guttenberger, W. Blankenfeldt and R. Breinbauer, Bioorg. Med. Chem., 2017, 25, 6149–6166 CrossRef PubMed.
- M. Koepf, S. H. Lee, B. J. Brennan, D. D. Méndez-Hernández, V. S. Batista, G. W. Brudvig and R. H. Crabtree, J. Org. Chem., 2015, 80, 9881–9888 CrossRef PubMed.
- S. A. Gamage, J. A. Spicer, G. W. Rewcastle, J. Milton, S. Sohal, W. Dangerfield, P. Mistry, N. Vicker, P. A. Charlton and W. A. Denny, J. Med. Chem., 2002, 45, 740–743 CrossRef PubMed.
- G. W. Rewcastle, W. A. Denny and B. C. Baguley, J. Med. Chem., 1987, 30, 843–851 CrossRef PubMed.
- S. Funayama, S. Eda, K. Komiyama, S. Ōmura and T. Tokunaga, Tetrahedron Lett., 1989, 30, 3151–3154 CrossRef.
- S. Wang, W. Miller, J. Milton, N. Vicker, A. Stewart, P. Charlton, P. Mistry, D. Hardick and W. A. Denny, Bioorg. Med. Chem. Lett., 2002, 12, 415–418 CrossRef PubMed.
- B. B. Fischer, A. Krieger-Liszkay and R. I. L. Eggen, Environ. Sci. Technol., 2004, 38, 6307–6313 CrossRef PubMed.
- J. M. Khurana, A. Chaudhary, A. Lumb and B. Nand, Green Chem., 2012, 14, 2321–2327 RSC.
- P. Singh, A. Baheti and K. R. J. Thomas, J. Org. Chem., 2011, 76, 6134–6145 CrossRef PubMed.
- S. Gupta and N. K. Khare, J. Mol. Struct., 2017, 1127, 309–313 CrossRef.
- G. W. Rewcastle, A. J. Bridges, D. W. Fry, J. R. Rubin and W. A. Denny, J. Med. Chem., 1997, 40, 1820–1826 CrossRef PubMed.
- R. B. Tenser, A. Gaydos and K. A. Hay, Antimicrob. Agents Chemother., 2001, 45, 3657–3659 CrossRef PubMed.
- P. Sharma, N. Rane and V. K. Gurram, Bioorg. Med. Chem. Lett., 2004, 14, 4185–4190 CrossRef PubMed.
- J. P. Cruz, T. Carrasco, G. Ortega and F. S. Cuesta, Lipids, 1992, 27, 192–194 CrossRef PubMed.
- M. Hayakawa, H. Kaizawa, H. Moritomo, T. Koizumi, T. Ohishi, M. Okada, M. Ohta, S.-i. Tsukamoto, P. Parker, P. Workman and M. Waterfield, Bioorg. Med. Chem., 2006, 14, 6847–6858 CrossRef PubMed.
- S. R. Natarajan, D. D. Wisnoski, S. B. Singh, J. E. Stelmach, E. A. O'Neill, C. D. Schwartz, C. M. Thompson, C. E. Fitzgerald, S. J. O'Keefe, S. Kumar, C. E. C. A. Hop, D. M. Zaller, D. M. Schmatz and J. B. Doherty, Bioorg. Med. Chem. Lett., 2003, 13, 273–276 CrossRef PubMed.
- M. Font, Á. González, J. A. Palop and C. Sanmartín, Eur. J. Med. Chem., 2011, 46, 3887–3899 CrossRef PubMed.
- Y. Yanagihara, H. Kasai, T. Kawashima and T. Shida, Jpn. J. Pharmacol., 1988, 48, 91–101 CrossRef PubMed.
- R. L. Smith, R. J. Barrett and E. Sanders-Bush, J. Pharmacol. Exp. Ther., 1995, 275, 1050–1057 Search PubMed.
- F. Awouters, J. Vermeire, F. Smeyers, P. Vermote, R. van Beek and C. J. E. Niemegeers, Drug Dev. Res., 1986, 8, 95–102 CrossRef.
- M. M. Badran, K. A. M. Abouzid and M. H. M. Hussein, Arch. Pharmacal Res., 2003, 26, 107 CrossRef.
- D. P. Singh, S. K. Deivedi, S. R. Hashim and R. G. Singhal, Pharmaceuticals, 2010, 3, 2416–2425 CrossRef PubMed.
- M. Waring, T. Ben-Hadda, A. Kotchevar, A. Ramdani, R. Touzani, S. Elkadiri, A. Hakkou, M. Bouakka and T. Ellis, Molecules, 2002, 7, 641 CrossRef.
- J. A. Pereira, A. M. Pessoa, M. N. D. S. Cordeiro, R. Fernandes, C. Prudêncio, J. P. Noronha and M. Vieira, Eur. J. Med. Chem., 2015, 97, 664–672 CrossRef PubMed.
- A. Monge, F. J. Martinez-Crespo, A. Lopez de Cerain, J. A. Palop, S. Narro, V. Senador, A. Marin, Y. Sainz and M. Gonzalez, J. Med. Chem., 1995, 38, 4488–4494 CrossRef PubMed.
- J. B. Rangisetty, C. N. V. H. B. Gupta, A. L. Prasad, P. Srinivas, N. Sridhar, P. Parimoo and A. Veeranjaneyulu, J. Pharm. Pharmacol., 2001, 53, 1409–1413 CrossRef PubMed.
- M. Kovalenko, A. Gazit, A. Böhmer, C. Rorsman, L. Rönnstrand, C.-H. Heldin, J. Waltenberger, F.-D. Böhmer and A. Levitzki, Cancer Res., 1994, 54, 6106–6114 Search PubMed.
- Y. K. Tailor, S. Khandelwal, K. Verma, R. Gopal and M. Kumar, ChemistrySelect, 2017, 2, 5933–5941 CrossRef.
- Y. K. Tailor, S. Khandelwal, R. Gopal, E. Rushell, A. Prajapati and M. Kumar, ChemistrySelect, 2017, 2, 11055–11061 CrossRef.
- A. Rajawat, S. Khandelwal and M. Kumar, RSC Adv., 2014, 4, 5105–5112 RSC.
- A. K. Arya and M. Kumar, Green Chem., 2011, 13, 1332–1338 RSC.
- A. Y. E. Abadi, M.-T. Maghsoodlou, R. Heydari and R. Mohebat, Res. Chem. Intermed., 2016, 42, 1227–1235 CrossRef.
- A. Hasaninejad and S. Firoozi, Mol. Diversity, 2013, 17, 499–513 CrossRef PubMed.
- A. Yazdani-Elah-Abadi, R. Mohebat, M.-T. Maghsoodlou and R. Heydari, Polycyclic Aromat. Compd., 2018, 38, 92–101 CrossRef.
- S.-L. Wang, F.-Y. Wu, C. Cheng, G. Zhang, Y.-P. Liu, B. Jiang, F. Shi and S.-J. Tu, ACS Comb. Sci., 2011, 13, 135–139 CrossRef PubMed.
- P. Saluja, A. Chaudhary and J. M. Khurana, Tetrahedron Lett., 2014, 55, 3431–3435 CrossRef.
- M.-T. M. Afshin Yazdani-Elah-Abadi, R. Mohebat and R. Heydari, Chin. Chem. Lett., 2017, 28, 446–452 CrossRef.
- G. H. Mahdavinia, M. Mirzazadeh and B. Notash, Tetrahedron Lett., 2013, 54, 3487–3492 CrossRef.
- R. Mohebat, A. Yazdani Elah Abadi and M.-T. Maghsoodlou, Res. Chem. Intermed., 2016, 42, 6039–6048 CrossRef.
- R. Mohebat, A. Yazdani Elah Abadi, M.-T. Maghsoodlou and M. Mohammadi, Res. Chem. Intermed., 2016, 42, 5915–5926 CrossRef.
- H. R. Shaterian and M. Mohammadnia, J. Mol. Liq., 2013, 177, 162–166 CrossRef.
- R. Mohebat, A. Y. E. Abadi, M.-T. Maghsoodlou, M. Mohammadi and R. Heydari, Res. Chem. Intermed., 2016, 42, 7121–7132 CrossRef.
- R. Bharti and T. Parvin, Mol. Diversity, 2016, 20, 867–876 CrossRef PubMed.
Footnote |
† Electronic supplementary information (ESI) available. See DOI: 10.1039/c8ra04919j |
|
This journal is © The Royal Society of Chemistry 2018 |
Click here to see how this site uses Cookies. View our privacy policy here.