DOI:
10.1039/C8RA04583F
(Paper)
RSC Adv., 2018,
8, 28405-28413
The sustainable synthesis of peptidomimetics via chemoenzymatic tandem oxidation–Ugi reaction†
Received
29th May 2018
, Accepted 26th July 2018
First published on 8th August 2018
Abstract
A simply and green synthetic protocol based on the selective laccase-oxidation of alcohol to a corresponding aldehyde and a following Ugi reaction in a micellar system made of SDS was developed and is reported herein. Special emphasis was placed on the metal-free chemoenzymatic tandem reaction based on alcohol oxidation strategies using molecular oxygen from air, followed by an Ugi reaction. The reaction was carried out without the use of a transition metal or organic solvents as a reaction medium. The presented protocol offers an efficient and environmentally friendly procedure.
Introduction
The sustainable synthesis of peptidomimetics is still a challenge for green chemistry.1 One of the methods used for the synthesis of these compounds relies on an Ugi reaction, which is recognized as an advanced tool for sustainable organic synthesis.2 The Ugi reaction is atom efficient since only one molecule of water is lost from the starting materials during the reaction.3 It has significant potential in molecule formation, which has seen it continuously used in the field of medicinal chemistry.4,5 The first example of the application of α-aminoacyl amides in pharmaceuticals was the local anesthetic xylocaine obtained in a one-pot Ugi reaction.6 Moreover, this type of reaction was used by process chemists at Merck for the production of the blockbuster anti-HIV drug Crixivan.7
As carbonyl reagents for the Ugi reaction, aldehydes or ketones are used. Owing to the increasing need for more economic and environmentally friendly synthetic protocols, the development of tandem oxidative processes combining an alcohol oxidation with a multicomponent reaction is actually of great interest.8–10
The generation of an aldehyde in situ in the reaction mixture allows overcoming some of the disadvantages, e.g., instability during storage leading to the formation of the corresponding alcohols, carboxylic acids, and other impurities.11 A great number of systems for the oxidation of alcohols to aldehydes, including TPAP (tetrapropylammonium perruthenate)12,13 and copper14–16 catalyses, are known.
It has been proved that the oxidation system is compatible with multicomponent reaction conditions and therefore can be combined in a one-pot procedure (Scheme 1).17–19 Che et al. described the oxidation of secondary amines to imines by singlet oxygen (generated from oxygen and the porphyrin photosensizer), followed by the Ugi reaction.20 Recently, Zhu et al. reported the tandem oxidation of alcohols using o-iodoxybenzoic acid (IBX) or Oxone® in the presence of a catalytic amount of sodium 2-iodobenzenesulfonate as an oxidant, followed by the Ugi (U-4CR) reaction (Scheme 1).21,22 One of the main drawbacks of IBX is its shock sensitivity, and limited solubility in many common organic solvents.23 For this purpose, to keep the reaction mixture homogeneous, a high dilution is required for the oxidation conditions, which is inconvenient for multicomponent reactions.24
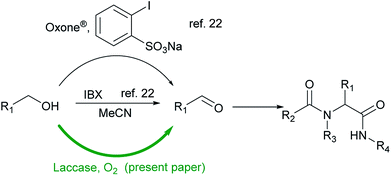 |
| Scheme 1 Methods used for the synthesis of peptidomimetics based on a tandem oxidation–Ugi reaction. | |
The application of metal complexes for the oxidation of an alcohol generates many impurities, which is intolerable for pharmaceutical or the cosmetic industry. This was justified by the fact that pharmacopeia limits of heavy metal contaminations are below 5 ppm. Therefore, most classical chemical methods applying metal catalysts are unacceptable.25
In order to overcome the existing limitations in the currently used experimental procedures to synthesize pollutants-free peptidomimetics, a new strategy is proposed herein. For the oxidation of alcohols, enzymatic oxidation can be used, which may be further combined with an Ugi reaction to provide the target compounds. In this system, water can be used as a solvent since both reactions proceed efficiently in this solvent.26
Water is cheap, safe and leads to the progress in environmentally safe chemical synthesis.27,28 However, water is not a perfect solvent due to the insolubility or low solubility of most organic compounds. In order to enhance the solubility of substrates, surfactants (surface active agents) as amphiphilic molecules can be used.29–31
For the oxidation of alcohols, the group of multi-copper oxidases, such as laccases (EC 1.10.3.2), show miscellaneous biochemical properties. Some are known for the oxidation of a wide range of electron-rich compounds, e.g., phenols,32 allylic alcohols,33,34 and aromatic alcohols,35–37 or amines38 using air oxygen as an electron acceptor (Scheme 2).
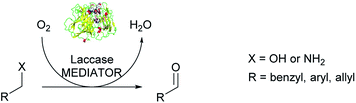 |
| Scheme 2 Schematic representation of LTv/TEMPO oxidation. | |
Enzymatic and micellar catalysis have been intensively investigated in our group and our ongoing studies are part of the research devoted to Ugi multicomponent reactions performed in aqueous media.39 In the present paper, we focus our attention on the chemoenzymatic tandem oxidation–Ugi reaction in a micellar system. Herein, we report a straightforward metal-free protocol for the synthesis of α-acylamino amides via the oxidation of primary alcohols by laccase from a Trametes versicolor (LTv)/TEMPO system, followed by an Ugi reaction. In the first step, the primary alcohol is selectively oxidized to an aldehyde by oxygen from air (without the usage of O2, which is potentially explosive), applying LTv as a catalyst and TEMPO as a mediator. Afterward, the formed aldehyde was applied in an Ugi reaction with carboxylic acid, amine, and isocyanide. Both reactions could be performed in an aqueous surfactant system, with increase productivity, without the need for the addition of an organic solvent or transition metal, which is beneficial from an environmental point of view.
Results and discussion
As components for the model chemoenzymatic tandem oxidation–Ugi reaction, benzyl alcohol (1a), p-methoxybenzylamine (3a), phenylacetic acid (4a), and p-methoxybenzyl isocyanide (5a) were selected. According to the literature data, benzyl alcohol (1a) is compatible with laccase-catalyzed oxidation, providing the corresponding aldehyde 2a exclusively.40,41 Recently, we proved that the Ugi reaction can be conducted in DDAB (didodecyldimethylammonium bromide) micelles.39
In present studies, we turned our attention to an aerobic oxidation of benzyl alcohol (1a) to benzaldehyde (2a) catalyzed by laccase from Trametes versicolor (LTv). We investigated the influence of didodecyldimethylammonium bromide (DDAB) on the reaction in the presence of LTv and TEMPO. For this purpose, we performed two parallel reactions: the oxidation of benzyl alcohol 1a in the presence and absence of DDAB. Both reactions were stirred overnight at room temperature. TLC analyses in both cases showed a full conversion to benzaldehyde (2a) exclusively. The experiments showed that an aerobic oxidation of benzyl alcohol could be performed efficiently in the presence of a surfactant. This observation encouraged us to combine this system with an Ugi-four component reaction (U-4CR). Recently, we presented the chemoenzymatic tandem oxidation–Passerini reaction based on the laccase-catalyzed oxidation of benzyl alcohol.17 Herein, we examined an analogous approach with the more demanding, four component Ugi reaction. For gaining more insights into the effect of the surfactant on the Ugi reaction, the condensation of benzaldehyde (2a) p-methoxybenzylamine (3a), phenylacetic acid (4a), and p-methoxybenzyl isocyanide (5a) in the presence of DDAB in phosphate buffer (PBS) pH 5.2 was investigated. The model product 6a was obtained with a 35% yield. Next, we performed the tandem oxidation–Ugi reaction in the presence of DDAB. The reaction was stirred overall for 72 h at room temperature, leading to 6a with a 30% yield.
For the first 24 h, enzymatic oxidation took place and next the rest of the substrates for the Ugi reaction were added. Due to the fact that oxidation, which is the first step of the chemoenzymatic tandem reaction, in the presence of surfactants is quantitative, the obtained yield of the target 6a was not satisfying. Therefore, we wondered if DDAB was likewise optimal for chemoenzymatic tandem reaction or only for the separated steps.
Furthermore, we investigated the influence of different types of surfactants (20 mol%) on the model Ugi reaction, with 1a, 3a, 4a, 5a as the substrates in the presence of LTv, TEMPO, in PBS pH 5.2. In all cases, the surfactants were used in the amount above their cmc (critical micellar concentration).42 The collected results indicated that SDS is optimal for the tandem Ugi reaction and was thus used in the further studies (Table 1).
Table 1 Effect of different surfactants on the model Ugi reactiona
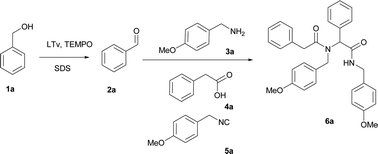
|
|
Additive |
Yieldb (%) |
Reaction conditions: benzyl alcohol (1a, 0.5 mmol), TEMPO (0.1 mmol), laccase from Trametes versicolor (15 mg), and the additive (0.1 mmol) were mixed for 24 h at room temperature. Then, p-metoxybenzylamine (3a, 0.5 mmol), phenylacetic acid (4a, 0.5 mmol), and p-methoxybenzyl isocyanide (5a, 0.5 mmol) were added and the reaction was stirred for an additional 48 h in 5 mL PBS (5.2 pH, 100 mM) at room temperature. Yields of isolated product 6a. |
1. |
— |
19 |
2. |
Tween 80 |
21 |
3. |
Triton X-100 |
19 |
4. |
Span 60 |
10 |
5. |
DDAB |
30 |
6. |
CTAB |
21 |
7. |
DODAB |
15 |
8. |
TPGS-750-M |
20 |
9. |
SDS |
45 |
10. |
AOT |
35 |
11. |
SDS/Triton X-100 (1 : 1) |
10 |
12. |
SDS/DDAB (1 : 1) |
<5 |
The literature data show that the type of mediator and reaction time have a great influence on the alcohol oxidation by the laccase. For this reason, HOBt was also tested as a mediator instead of TEMPO.43 However, when HOBt was applied, the formation of any product was observed (Table 2, entry 8). In the next step, we investigated the influence of time on the reaction course. The optimal time for the chemoenzymatic tandem reaction was 72 h, affording product 6a with a 45% yield (Table 2, entries 4–7). Further prolongation of the reaction time did not affect the reaction yield. We also tested the impact of other parameters, such as the mediator and enzyme, on the studied chemoenzymatic tandem reaction (Table 2, entries 1 and 2). When one of the mentioned components of the studied reaction was absent, the formation of the desired product 6a was not observed. The addition of SDS enhanced significantly the yield of 6a from 19% to 45% (Table 2, entries 3, 6). The obtained results indicated that the LTv/TEMPO system was the most efficient for the presented tandem reaction.
Table 2 Aerobic oxidation of benzyl alcohol (1a) by the LTv/TEMPO system combined with an Ugi reactiona
|
Mediator |
Time (h) |
Yielde (%) |
Reaction conditions: benzyl alcohol (1a, 0.5 mmol), mediator (0.1 mmol), laccase from Trametes versicolor (15 mg), SDS (0.1 mmol) in 5 mL PBS (5.2 pH, 100 mM) for 24 h at room temperature. Then, p-metoxybenzylamine (3a, 0.5 mmol), phenylacetic acid (4a, 0.5 mmol) and p-methoxybenzyl isocyanide (5a, 0.5 mmol) were added. Estimated by TLC. Reaction carried out without enzyme. Reaction carried out without SDS. Yields of isolated product 6a. |
1. |
— |
72 |
<1b |
2. |
TEMPO |
72 |
NDb,c |
3. |
TEMPO |
72 |
19d |
4. |
TEMPO |
24 |
<1b |
5. |
TEMPO |
48 |
11 |
6. |
TEMPO |
72 |
45 |
7. |
TEMPO |
96 |
45 |
8. |
HOBt |
72 |
<1b |
According to the literature data, the stability and activity of laccase from Trametes versicolor strictly depend on the reaction conditions (e.g., pH, concentration of surfactant, temperature).44–47 It is well known that laccase, which was used in our studies, is active in the pH range 3.0 to 6.0.44,48 With this data in mind, we tested the optimal pH for the model reaction. We carried out reactions between the model substrates (1a, 3a, 4a, 5a) in the presence of SDS, TEMPO, and LTv in phosphate buffer in the pH range between 3.0 and 7.0. The obtained results are given in Fig. 1. Initially, pH 5.2 was optimal for the model tandem LTv/TEMPO oxidation-U4CR one-pot system. Between pH 3.0 and 5.2, the yield of the product 6a increased to 45%. In the range of pH 5.2 to 6.0, the yield varied slightly. Above pH 6.0, the yield of 6a decreased significantly, which can be explained by the lower activity of laccase.48
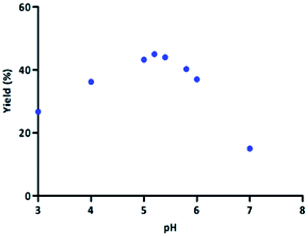 |
| Fig. 1 Effect of pH on the isolated reaction yield of 6a of the model laccese/TEMPO oxidation-U4CR system. Reaction conditions: benzyl alcohol (1a, 0.5 mmol), TEMPO (0.1 mmol), laccase from Trametes versicolor (15 mg), SDS (0.1 mmol) in 5 mL PBS (5.2 pH, 100 mM) were mixed for 24 h at room temperature. Then, p-metoxybenzylamine (3a, 0.5 mmol), phenylacetic acid (4a, 0.5 mmol) and p-methoxybenzyl isocyanide (5a, 0.5 mmol) were added and stirred for an additional 48 h. | |
Due to the fact that the concentration of the surfactant can influence the reaction course, we performed additional studies. We investigated the surfactant content (from 0 to 100 mol%) on the chemoenzymatic tandem reaction. The results are presented in Fig. 2. In all cases, the reaction yield in the presence of SDS was higher than in pure PBS. While upon changing the amount of SDS from 0 to 20 mol%, the yield of product 6a increased up to 45%. Further elevation of the SDS concentration caused a reduction in the reaction yield to 28% (40 mol% of SDS). Increasing SDS from 40 to 100 mol% did not have any influence on the reaction yield. Due to the collected data of SDS, 20 mol% of SDS was selected for the further studies.
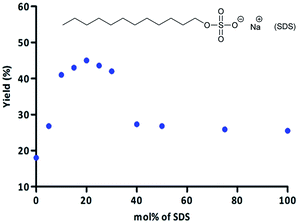 |
| Fig. 2 Effect of SDS on the isolated yield of 6a from the model laccase/TEMPO oxidation-U4CR system. Reaction conditions: benzyl alcohol (1a, 0.5 mmol), TEMPO (0.1 mmol), laccase from Trametes versicolor (15 mg), SDS (0.1 mmol) in 5 mL PBS (5.2 pH, 100 mM) were mixed for 24 h at room temperature. Then, p-metoxybenzylamine (3a, 0.5 mmol), phenylacetic acid (4a, 0.5 mmol) and p-methoxybenzyl isocyanide (5a, 0.5 mmol) were added and stirred for an additional 48 h. | |
Very important from an environmental point of view is the reusability of a catalytic system. Therefore, we investigated if the catalytic system containing the micelles of SDS, LTv, and TEMPO could be used more than once. The filtrate containing the surfactant was used as a medium for the next reaction. The precipitate of 6a was purified by recrystallization from n-hexane/ethyl acetate. The SDS micelles were used for three consecutive reaction cycles. It was found that there was a gradual decrease in reaction yield from 45% to 10%, probably caused by the loss of surfactant, laccase, or TEMPO during filtration.
After the optimization of the U-4CR, a series of experiments with various alcohols 1, carboxylic acids 4, and isocyanides 5 was performed, providing α-aminoacyl amides 6a–6r (Table 4). The isolated yields of 6 were determined after 72 h at room temperature and were compared with the yields from the Ugi reaction carried out from the corresponding aldehyde (Table 4). For the first part of the experiments, the influence of different alcohols on the chemoenzymatic tandem reaction was investigated. For the reactions carried out with different derivatives of benzyl alcohol 1, the products 6a–6f from the Ugi reaction were isolated with yields up to 45%. It was worth noticing that the oxidation of alcohols was quantitative for all the used benzyl alcohol derivatives (1a–1f). The obtained results show that the use of alcohols with electron-withdrawing or electron-donating groups at the aromatic ring did not have a significant impact on enzymatic oxidation and revealed that the yield of the tandem process was limited by the Ugi reaction. The products of allyl alcohol 6h and ethanol 6g were not observed. Reactions carried out with aliphatic alcohol, like dodecanol, resulted in the product 6i with a yield of 11%. According to the literature data, the enzymatic oxidation of aliphatic alcohols catalyzed by LTv does not proceed qualitatively, which results in a low yield of the tandem process.49 Next, the influence of different isocyanides 5 and carboxylic acids 4 was investigated on the chemoenzymatic tandem reaction. The application of benzyl isocyanide resulted in the product 6j with a 48% yield, while the reaction with cyclohexyl isocyanide resulted in product 6k with a 30% yield. The use of n-butyl and t-butyl isocyanides resulted in yields of 35% of 6m and 33% of 6l, respectively. As can be seen, the developed conditions using SDS promoted the Ugi reaction with aromatic isocyanides as the substrates. Replacing phenylacetic acid with hydrocynamic acid resulted in the product 6n with a higher yield of 55% vs. 45%.
Table 3 Effect of laccase from Trametes versicolor or TEMPO on the Ugi reaction in the presence of SDS micellesa
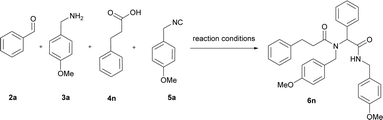
|
|
Reaction conditions |
Yield of 6nd (%) |
Reaction conditions of benzaldehyde (2a, 0.5 mmol), p-metoxybenzylamine (3a, 0.5 mmol), hydrocynamic acid (4n, 0.5 mmol), p-methoxybenzyl isocyanide (5a, 0.5 mmol), SDS (0.1 mmol) in 5 mL PBS (5.2 pH, 100 mM) for 48 h at room temperature. Laccase from Trametes versicolor (15 mg). TEMPO (0.1 mmol). Yields of isolated product 6a. |
1. |
SDS micelles |
67 |
2. |
LTv, SDS micelles |
48b |
3. |
TEMPO, SDS micelles |
20c |
4. |
LTv, TEMPO, SDS micelles |
Traceb,c |
Table 4 Scope and limitation of the LTv/TEMPO oxidation U4CR system
Reaction conditions: aldehyde 2 (0.5 mmol), amine 3 (1 eq.), carboxylic acid 4 (1 eq.), and isocyanide 5 (1 eq) SDS (0.1 mmol) in 5 mL of phosphate buffer (pH 5.2, 100 mM) were stirred for 48 h at room temperature. Reaction conditions: alcohol 1 (0.5 mmol), TEMPO (0.1 mmol), LTv (15 mg), and SDS (0.1 mmol) in 5 mL of phosphate buffer (pH 5.2, 100 mM) were stirred for 24 h at room temperature. Next, amine 3 (1 eq.), carboxylic acid 4 (1 eq.), and isocyanide 5 (1 eq.) were added and the reaction was stirred for an additional 48 h at room temperature. |
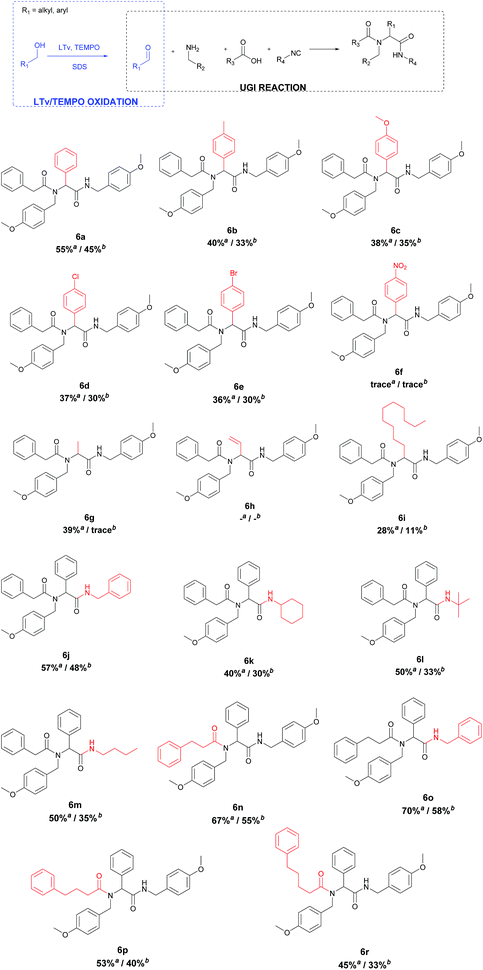 |
However, the application of 4-phenylbutyric acid and 5-phenylvaleric acid in the presence of SDS led to products 6p and 6r with lower yields of 53% and 45%, respectively, the products 6a–6r were obtained with the SDS micellar system using the corresponding aldehydes in slightly higher yields in most cases than in the chemoenzymatic tandem reaction (Table 4).
To clarify the higher yields of the classical Ugi reaction compared to the chemoenzymatic tandem reaction, additional experiments were performed. The influence of LTv and TEMPO on the classical Ugi reaction in the presence of SDS micelles was examined (Table 3). Benzaldehyde (2a), p-methoxybenzyl amine (3a), hydrocynamic acid (4n) and p-methoxybenzyl isocyanide (5a) were stirred in PBS at pH 5.2 in the presence of SDS to give the product 6n with a 67% yield (Table 3, entry 1). The addition of LTv or TEMPO to the reaction reduced the yield of 6n to 48% and 20%, respectively (Table 3, entries 2, 3). In the case when the reaction was performed in the presence of TEMPO and LTv, the product of 6n was not observed (Table 3, entry 4). Next, we also tested other substrates for the Ugi reaction alone with LTv and TEMPO in the presence of SDS micelles. It is well known that benzyl amine can be oxidized to aldehyde via laccase.50 With these data in mind, we verified the substrate 3a in the presence of LTv, TEMPO, and SDS micelles. The p-methoxybenzyl amine was oxidized to p-anisaldehyde with a 33% yield. This indicated that the lower yield in oxidative tandem oxidation–Ugi reaction was the result of the descending concentration of amine during the reaction course performed with laccase. Next, the excess of p-methoxybenzyl amine was investigated on the Ugi reaction in micellar media in the presence of LTv and TEMPO. We observed that in the reaction with 2 eq. of p-methoxybenzyl amine 3a, benzaldehyde (2a, 1 eq.), hydrocynamic acid (4a, 1 eq.), and p-methoxybenzyl isocyanide (5a, 1 eq.) in the presence of SDS micelles enhanced the reaction yield of 6n to 65%. Also the application of 2 eq. of 3a in the reaction with 1a, 4n, and 5a in the presence of LTv and TEMPO in micellar media resulted in the product 6n in a higher yield (60%).
Furthermore, we wondered whether the laccase transformed the products from the Ugi reaction. In this purpose, the stability of product 6n was examined. After 6 days, the compound 6n was recovered with 85% yield. According to the literature data, the carboxylic acids can form dimers or polymers in the presence of laccase.51 Therefore, we verified whether the hydrocynamic acid could react with itself in the presence of TEMPO, LTv, and SDS. However, no new product was observed. It is well known that the isocyanides 5 have low stability and spontaneous hydrolysis in aqueous solution.52 Due to the fact that the synthesis of isocyanides requires a toxic reagent, such as phosphoryl chloride (POCl3) their sustainable application is contentious and questionable. To overcome this inconvenience, phase-transfer catalysis (PTC)53 was applied, leading to the isocyanide 5a with a 66% yield. The reaction was performed from p-methoxybenzyl amine 3a using 50% NaOH solution, CHCl3, and TBAB (see ESI†).
Conclusions
We developed a green and environmentally sustainable method for the synthesis of peptidomimetics As substrates, several alcohols were used, which were oxidized by laccase, followed by Ugi reaction providing the target compounds. No transition metal, toxic organic solvents, or harsh oxidants were required. To achieve high substrate solubility, water surfactants were used. The influence of different surfactants on the course of chemoenzymatic tandem Ugi reaction was investigated. We found that the presence of the anionic SDS surfactant had a beneficial effect on the reaction yield compared to pure PBS. During our studies, the optimal concentration and the reusability of SDS were determined. After a detailed optimization of the reaction conditions, the scope of the substrates was evidenced. 17 peptidomimetics of a structure of α-aminoacyl amides were obtained and fully characterized. A comparison of the two protocols: chemoenzymatic tandem oxidation–Ugi reaction with classical Ugi reaction in the presence of SDS micelles was made. In most cases, the chemoenzymatic tandem oxidation–Ugi reaction resulted in slightly lower yields, but the products were obtained free from any impurities, which is of great importance for medicinal chemistry applications.
Experimental
1H NMR and 13C NMR spectra were recorded in CDCl3 with Bruker 400 MHz spectrometers. Tetramethylsilane (TMS) was used as an internal standard. TLC was performed on Kieselgel 60 F254 aluminum sheets. Melting points were determined with a model SMP-20 device from Buchi (Flawil, Switzerland). Fluorescence spectra were recorded in quartz cuvettes with an F-7000 spectrofluorometer (Shimadzu, Kyoto, Japan). Laccase from Trametes versicolor, as a powder, light brown, ≥0.5 U mg−1, was purchased from Sigma Aldrich, product number 38429.
Almost all the chemicals were commercially available. Only p-methoxybenzyl isocyanide, hexylisocyanide, and 2,4-dimethoxybenzylisocyanide were synthesized from the corresponding amine. Dilauryldimethylammonium bromide (
didodecyldimethylammonium bromide (DDAB), purity > 98.0%), was purchased from TCI, product number D1974. Dioctadecyldimethylammonium bromide (DODAB), purity ≥ 98.0% (AT), was purchased from Sigma Aldrich, product number 40165. Dioctylsulfosuccinate sodium salt (AOT = sodium bis(2-ethylhexyl) sulfosuccinate, purity 98%), was purchased from Sigma Aldrich, product number 323586. Sorbitan monostearate (Span 60) was purchased from TCI, product number GL01-YQ. Tween 80 was purchased from Schuchardt Mȕnchen (now Merck). Sodium dodecyl sulfate (SDS), purity ≥ 99.0% (GC), was purchased from Sigma Aldrich, product number L6026. Triton X-100 was purchased from Sigma Aldrich, product number T9284. TPGS-750-M (DL-α-tocopherol methoxypolyethylene glycol succinate) was purchased from Sigma Aldrich, product number 763896. Hexadecyltrimethylammonium bromide (CTAB), purity ≥ 99.0%, was purchased from Sigma Aldrich, product number H9151. The remaining starting materials were purchased from Sigma Aldrich or TCI.
General procedure for the synthesis of products 5a–r (with SDS as additive)
An alcohol (0.5 mmol), laccase from Trametes versicolor (20 mg), TEMPO (0.1 mmol), and SDS (0.1 mmol) were stirred in phosphate buffer (5 ml, pH 5.2, c = 0.1 M) at room temperature. After 24 h, an amine (0.5 mmol), carboxylic acid (0.5 mmol), and isocyanide (0.5 mmol) were added and the mixture was stirred for an additional 48 h. The reaction was carried out in an open vessel. After extraction with ethyl acetate or dichloromethane (3 × 20 mL), the resulting combined organic layers were dried with MgSO4 and then, the solvent was removed by distillation under reduced pressure. The product was purified by column chromatography (silica gel, hexane/AcOEt; 6
:
4/v/v). The NMR spectra are given in the ESI.†
6a N-[(4-Methoxyphenyl)methyl]-2-[(4-methoxyphenyl)methyl-(2-phenylacetylo)amino]-2-phenylacetamide
Pale yellow oil; 1H NMR (400 MHz, CDCl3) δ 7.14–7.25 (10H, m, Ph), 7.04–7.10 (2H, m, Ph), 6.84 (2H, m, Ph), 6.73 (2H, m, Ph), 6.64 (2H, m, Ph), 6.03 (1H, s br, NH), 5.78 (1H, s, CH), 4.60 (1H, m, CHa), 4.40 (1H, m, CHb), 4.27 (2H, m, CH2), 3.68 (6H, m, 2 × CH3), 3.61 (2H, m, CH2). 13C NMR (100 MHz; CDCl3) δ ppm = 40.9, 42.8, 54.8, 54.9, 63.1, 113.5, 113.6, 126.4, 127.1, 127.1, 128.2, 128.2, 128.2, 128.3, 128.4, 128.4, 128.5, 128.5, 128.6, 128.7, 129.3, 134.3, 158.3, 169.0, 172.4; HRMS calcd for C32H32N2O4Na [M + Na]+: 521.2255 found: 531.2260.
6b N-[(4-Methoxyphenyl)methyl]-2-[(4-methoxyphenyl)methyl(2-phenylacetylo)amino]-2-[4-methylphenyl]acetamide
Pale yellow oil; 1H NMR (400 MHz, CDCl3) δ 7.05–7.22 (9H, m, Ph), 6.96–6.98 (2H, m, Ph), 6.86–6.88 (2H, m, Ph), 6.72–6.75 (2H, m, Ph), 6.65–6.67 (2H, m, Ph), 5.90 (1H, s br, NH), 5.67 (1H, s, CH), 4.56–4.60 (1H, m, CHa), 4.38–4.29 (3H, m, CH2 + CHb), 3.59–3.70 (6H, m, 2 × CH3 + CH2), 2.20 (3H, s, CH3). 13C NMR (100 MHz; CDCl3) δ ppm = 20.9, 41.1, 43.0, 55.1, 55.1, 63.1, 113.7, 113.8, 128.4, 128.8, 128.8, 128.9, 129.3, 129.5, 134.7, 138.2, 158.6, 169.5, 172.6; HRMS calcd for C33H34N2O4Na [M + Na]+: 545.2416 found: 545.2418.
6c N-[(4-Methoxyphenyl)methyl]-2-[(4-methoxyphenyl)methyl-(2-phenylacetylo)amino]-2-[4-methoxyphenyl]acetamide
Pale yellow oil; 1H NMR (400 MHz, CDCl3) δ 7.12–7.31 (11H, m, Ph), 6.89–6.91 (2H, m, Ph), 6.81–6.83 (2H, m, Ph), 6.73–6.75 (2H, m, Ph), 6.10 (1H, s br, NH), 5.73 (1H, s, CH), 4.64–4.68 (1H, m, CHa), 4.34–4.47 (3H, m, CH2 + CHb), 3.71–3.79 (6H, m, 2 × CH3 + CH2). 13C NMR (100 MHz; CDCl3) δ ppm = 43.1, 50.0, 55.2, 62.5, 114.0, 126.9, 127.4, 127.4, 128.6, 128.7, 128.7, 128.8, 128.8, 129.0, 130.9, 130.9, 134.4, 134.5, 158.8, 172.8, 178.0; HRMS calcd for C32H31N2O4Na [M + Na]+: 565.1872 found: 565.1873.
6d N-[(4-Methoxyphenyl)methyl]-2-[(4-methoxyphenyl)methyl-(2-phenylacetylo)amino]-2-[4-chlorophenyl]acetamide
Pale yellow oil; 1H NMR (400 MHz, CDCl3) δ 7.12–7.31 (11H, m, Ph), 6.89–6.91 (2H, m, Ph), 6.81–6.83 (2H, m, Ph), 6.73–6.75 (2H, m, Ph), 6.10 (1H, s br, NH), 5.73 (1H, s, CH), 4.64–4.68 (1H, m, CHa), 4.34–4.47 (3H, m, CH2 + CHb), 3.71–3.79 (6H, m, 2 × CH3 + CH2). 13C NMR (100 MHz; CDCl3) δ ppm = 43.1, 50.0, 55.2, 62.5, 114.0, 126.9, 127.4, 127.4, 128.6, 128.7, 128.7, 128.8, 128.8, 129.0, 130.9, 130.9, 134.4, 134.5, 158.8, 172.8, 178.0; HRMS calcd for C32H31ClN2O4Na [M + Na]+: 493.2464 found: 493.2467.
6e N-[(4-Methoxyphenyl)methyl]-2-[(4-methoxyphenyl)methyl-(2-phenylacetylo)amino]-2-[4-bromophenyl]acetamide
Pale yellow oil; 1H NMR (400 MHz, CDCl3) δ 7.12–7.36 (12H, m, Ph), 6.89–6.91 (2H, m, Ph), 6.84–6.81 (2H, m, Ph), 6.75–6.73 (2H, m, Ph), 6.13 (1H, s br, NH), 5.71 (1H, s, CH), 4.64–4.68 (1H, m, CHa), 4.34–4.47 (3H, m, CH2 + CHb), 3.71–3.79 (8H, m, 2 × CH3 + CH2). 13C NMR (100 MHz; CDCl3) δ ppm = 41.2, 43.1, 50.0, 55.2, 62.4, 113.9, 122.5, 126.9, 127.4, 127.4, 128.6, 128.8, 128.8, 129.0, 131.2, 131.6, 134.4, 151.2, 158.8, 168.8, 172.7; HRMS calcd for C32H31BrN2O4Na [M + Na]+: 467.2311 found: 467.2309.
6g N-[(4-Methoxyphenyl)methyl]-2-[(4-methoxyphenyl)methyl-(2-phenylacetylo)amino]-2-propionamide
White powder; mp. 94–95 °C; 1H NMR (400 MHz; CDCl3) δ ppm = 7.13–7.17 (4H, m, Ph), 6.96–7.05 (5H, m, Ph), 6.65–7.77 (5H, m, Ph + CH), 4.94–4.99 (1H, m, NH), 4.37–4.49 (2H, q, J = 17.7 Hz, CH2), 4.15–4.17 (2H, d, J = 5.5 Hz, CH2), 3.69 (6H s, 2 × CH3), 3.52–3.54 (2H, m, CH2), 1.18–1.26 (3H, m, CH3); 13C NMR (100 MHz; CDCl3) δ ppm = 14.0, 41.0, 42.7, 42.7, 47.8, 53.2, 55.1, 113.8, 114.1, 126.8, 126.9, 128.5, 128.7, 128.8, 129.0, 129.1, 130.2, 134.4, 158.8, 170.9, 172.9; HRMS calcd for C27H30N2O4Na [M + Na]+: 469.2103 found: 469.2099.
6i N-[(4-Methoxyphenyl)methyl]-2-[(4-methoxyphenyl)methyl-(2-phenylacetylo)amino]-2-dodecanamide
Yellow powder; mp. 92–93 °C; 1H NMR (400 MHz; CDCl3) δ ppm = 7.17–7.19 (4H, m, Ph), 6.97–7.02 (5H, m, Ph), 6.77–7.74 (5H, m, Ph + CH), 4.76–4.79 (1H, m, NH), 4.46 (2H, s, CH2), 4.16–4.22 (2H, q, J = 17.2 Hz CH2), 3.72 (6H s, 2 × CH3), 3.55–3.56 (2H, m, CH2), 1.11–1.21 (20H, m, CH2), 0.79–0.83 (3H, m, CH3); 13C NMR (100 MHz; CDCl3) δ ppm = 14.0, 22.6, 26.4, 28.3, 29.3, 29.4, 29.4, 29.5, 31.9, 41.3, 42.8, 42.8, 48.3, 55.2, 58.5, 114.0, 114.2, 126.9, 127.3, 128.6, 128.7, 128.9, 129.0, 129.2, 130.3, 134.5, 158.9, 170.4, 173.3; HRMS calcd for C36H48N2O4Na [M + Na]+: 483.2260 found: 483.2256.
6j N-Benzyl-2-[(4-methoxyphenyl)methyl-(2-phenylacetylo)amino]-2-phenylacetamide
Pale yellow oil; 1H NMR (400 MHz, CDCl3) δ 7.09–7.27 (15H, m, Ph), 6.83–6.85 (2H, m, Ph), 6.62–6.64 (2H, m, Ph), 6.05 (1H, s br, NH), 5.73 (1H, s, CH), 4.54–4.57 (1H, m, CHa), 4.36–4.37 (3H, m, CH2 + CHb), 3.61–3.71 (5H, m, CH3 + CH2); 13C NMR (100 MHz; CDCl3) δ ppm = 43.1, 43.5, 55.1, 55.1, 66.8, 113.7, 127.2, 127.5, 128.4, 128.5, 128.6, 128.7, 128.8, 129.7, 134.6, 158.6, 172.6, 174.1, HRMS calcd for C31H30N2O3Na [M + Na]+: 501.2154 found: 501.2154.
6k N-Cyclohexyl-2-[(4-methoxyphenyl)methyl-(2-phenylacetylo)amino]-2-phenylacetamide
Pale yellow oil; 1H NMR (400 MHz, CDCl3) δ 7.19–7.42 (10H, m, Ph), 6.84–6.86 (2H, m, Ph), 6.68–6.65 (2H, m, Ph), 5.76 (1H, s br, NH), 5.54 (1H, s, CH), 4.57–4.62 (1H, d, J = 17.9 Hz, CHa), 4.36–4.41 (1H, d, J = 17.6 Hz, CHb), 3.60–3.69 (5H, m, CH3 + CH2), 1.81 (2H, m, CH2), 1.51 (2H, m, CH2), 1.26–1.19 (2H, m, CH2), 0.99 (2H, m, CH2), 0.81–0.76 (1H, m, CH); 13C NMR (100 MHz; CDCl3) δ ppm = 24.7, 24.1, 25.4, 32.7, 41.3, 48.4, 55.2, 113.9, 113.9, 126.8, 127.4, 128.3, 128.4, 128.4, 128.6, 128.6, 128.6, 128.9, 128.9, 129.6, 134.8, 134.8, 158.7, 168.4, 172.7; HRMS calcd for C30H34N2O4Na [M + Na]+: 493.2467 found: 493.2464.
6l N-t-Butyl-2-[(4-methoxyphenyl)methyl-(2-phenylacetylo)amino]-2-phenylacetamide
Pale yellow oil; 1H NMR (400 MHz, CDCl3) δ 7.10–7.26 (10H, m, Ph), 6.79–6.80 (2H, m, Ph), 6.61–6.65 (2H, m, Ph), 5.82 (1H, s br, NH), 5.66 (1H, s, CH), 4.58–4.62 (1H, m, CHa), 4.41–4.45 (1H, m, CHb), 3.56–3.67 (3H, m, CH3 + CH2), 1.06–1.35 (10H, m, CH(CH3)3). 13C NMR (100 MHz; CDCl3) δ ppm = 28.5, 51.5, 55.2, 113.7, 113.8, 127.3, 128.3, 128.5, 128.7, 128.9, 129.3, 129.4, 134.7, 158.6, 168.8, 172.9; HRMS calcd for C28H32N2O3Na [M + Na]+: 467.2311 found: 467.2309.
6m N-n-Butyl-2-[(4-methoxyphenyl)methyl-(2-phenylacetylo)amino]-2-phenylacetamide
Pale yellow oil; 1H NMR (400 MHz, CDCl3) δ 7.14–7.33 (10H, m, Ph), 6.91–6.93 (2H, m, Ph), 6.72–6.74 (2H, m, Ph), 6.10 (1H, s br, NH), 5.93 (1H, s, CH), 4.67–4.71 (1H, d, J = 17.5 Hz, CHa), 4.54–4.49 (1H, d, J = 17.3 Hz, CHb), 3.65–3.74 (5H, m, CH3 + CH2), 3.65–3.74 (2H, m, CH2), 3.19 (2H, s, CH2), 1.22–1.38 (4H, m, C2H4), 0.85–0.88 (3H, m, CH3). 13C NMR (100 MHz; CDCl3) δ ppm = 13.2, 19.5, 30.9, 38.9, 40.8, 49.4, 54.7, 62.6, 113.4, 126.3, 127.0, 128.0, 128.0, 128.1, 128.2, 128.5, 128.9, 129.2, 134.3, 158.2, 169.0, 172.4; HRMS calcd for C28H32N2O3Na [M + Na]+: 467.2311 found: 467.2306.
6n N-[(4-Methoxyphenyl)methyl]-2-[(4-methoxyphenyl)methyl-3-(phenylpropionyl)amino]-2-phenylacetamide
Pale yellow oil; 1H NMR (400 MHz, CDCl3) δ 7.08–7.29 (12H, m, Ph), 6.81–6.86 (4H, m, Ph), 6.61–6.67 (2H, m, Ph), 6.14 (1H, s br, NH), 5.90 (1H, s, CH), 4.59–4.63 (1H, m, CHa), 4.54–4.70 (3H, m, CH2 + CHb), 3.73–3.77 (6H, m, 2 × CH3), 2.93–2.95 (2H, m, CH2), 2.54–2.70 (2H, m, CH2). 13C NMR (100 MHz; CDCl3) δ ppm = 31.3, 35.7, 43.1, 49.6, 55.1, 55.2, 63.2, 113.8, 113.9, 126.0, 127.3, 128.3, 128.4, 128.6, 128.9, 129.2, 129.6, 141.0, 158.5, 158.9, 169.5, 174.0; HRMS calcd for C33H34N2O4Na [M + Na]+: 545.2416 found: 545.2413.
6o N-Benzyl-2-[(4-methoxyphenyl)methyl-4-(phenylprobutyryl)amino]-2-phenylacetamide
1H NMR (400 MHz, CDCl3) δ 7.16–7.32 (12H, m, Ph), 7.06–7.08 (2H, m, Ph), 6.85–6.87 (2H, m, Ph), 6.64–6.70 (3H, m, Ph + NH), 6.07 (1H, s, CH), 4.62–4.67 (1H, m, CHa), 4.44–4.51 (3H, m, CHb + CH2), 3.73 (3H, s, CH3), 2.88–2.93 (2H, m, CH2), 2.56–2.68 (2H, m, CH2). 13C NMR (100 MHz; CDCl3) δ ppm = 31.3, 35.7, 43.6, 49.6, 55.2, 63.0, 113.8, 126.0, 127.3, 127.3, 127.6, 128.4, 128.5, 128.6, 128.7, 129.5, 129.7, 135.1, 138.2, 141.0, 158.6, 169.8, 174.2; HRMS calcd for C32H32N2O3Na [M + Na]+: 515.2311 found: 515.2310.
6p N-[(4-Methoxyphenyl)methyl]-2-[(4-methoxyphenyl)methyl-4-(phenylbutyryl)amino]-2-phenylacetamide
Pale yellow oil; 1H NMR (400 MHz, CDCl3) δ 6.19–6.58 (12H, m, Ph), 5.78–6.10 (4H, m, Ph), 6.85–6.87 (2H, m, Ph), 5.30 (1H, s, NH), 5.04 (1H, s, CH), 3.68–3.73 (1H, m, CHa), 3.45–3.73 (3H, m, CHb + CH2), 2.87 (3H, s, CH3), 1.66–1.78 (2H, m, CH2), 1.28–1.49 (2H, m, CH2), 1.04–1.12 (2H, m, CH2). 13C NMR (100 MHz; CDCl3) δ ppm = 26.7, 33.3, 35.1, 43.1, 49.7, 55.2, 55.2, 63.2, 113.8, 114.0, 15.8, 127.4, 128.2, 128.3, 128.4, 128.5, 128.7, 129.0, 129.1, 129.7, 141.7, 158.6, 158.9, 169.6, 174.7; HRMS calcd for C32H32N2O3Na [M + Na]+: 506.2569 found: 506.2566.
6r N-[(4-Methoxyphenyl)methyl]-2-[(4-methoxyphenyl)methyl-4-(phenylpentyl)amino]-2-phenylacetamide
Pale yellow oil; 1H NMR (400 MHz, CDCl3) δ 7.24 (2H, m, Ph), 7.14–7.18 (5H, m, Ph), 7.02–7.11 (5H, m, Ph), 6.81–6.83 (2H, m, Ph), 6.71–6.75 (2H, m, Ph), 6.61–6.63 (2H, m, Ph), 6.06 (1H, s, NH), 5.82 (1H, s, CH), 4.54–4.58 (1H, d, J = 17.2 Hz, CHa), 4.26–4.41 (3H, m, CHb + CH2), 3.60–3.68 (6H, m, CH3 × 2), 2.44–2.48 (2H, m, CH2), 2.10–2.33 (2H, m, CH2), 1.47–1.66 (4H, m, C2H4). 13C NMR (100 MHz; CDCl3) δ ppm = 24.7, 30.9, 33.8, 35.6, 43.1, 49.7, 55.1, 55.2, 63.2, 113.8, 113.9, 125.6, 127.3, 128.2, 128.3, 128.3, 128.4, 128.6, 128.9, 129.1, 129.6, 142.2, 158.8; HRMS calcd for C34H36N2O3Na [M + Na]+: 520.2726 found: 520.2722.
Conflicts of interest
There are no conflicts to declare.
Acknowledgements
We gratefully acknowledge the financial support of the Polish State Committee for Scientific Research project OPUS No. 2016/23/B/ST5/03307 for financial support.
Notes and references
- S. B. Lawrenson, R. Arav and M. North, Green Chem., 2017, 19, 1685 RSC.
- R. C. Cioc, E. Ruijter and R. V. A. Orru, Green Chem., 2014, 16, 2958 RSC; Y. Gu, Green Chem., 2014, 16, 2958 RSC.
- I. Ugi, B. Werner and A. Dömling, Molecules, 2003, 8, 53 CrossRef.
- R. Wamg and Z.-Q. Liu, J. Org. Chem., 2013, 78, 8696 CrossRef PubMed.
- K. Rossen, P. J. Pye, L. M. DiMichele, R. P. Volante and P. K. Reider, Tetrahedron Lett., 1998, 39, 6823 CrossRef.
- I. Ugi and C. Steinbrückner, US Pat. 3247200, 1966.
- C. Hulme and V. Gore, Curr. Med. Chem., 2003, 10, 51 CrossRef PubMed.
- R. J. K. Taylor, M. Reid, J. Foot and S. A. Raw, Acc. Chem. Res., 2005, 38, 851 CrossRef PubMed.
- M. Davi and H. Lebel, Org. Lett., 2009, 11, 41 CrossRef PubMed.
- B. E. Maki and K. A. Sheidt, Org. Lett., 2009, 11, 1651 CrossRef PubMed.
- W. L. F. Armarego and C. L. L. Chai, Purification of Laboratory Chemicals, Elsevier, Amsterdam, 6th edn, 2009, vol. 2, p. 71 Search PubMed.
- S. V. Ley, J. Norman, W. P. Griffith and S. P. Marsden, Synthesis, 1994, 639 CrossRef.
- I. E. Markó, P. R. Giles, M. Tsukazaki, I. Chélle-Regnaut, C. J. Urch and S. M. Brown, J. Am. Chem. Soc., 1997, 119, 12661 CrossRef.
- I. E. Markó, A. Gautier, P. R. Giles, I. Chélle-Regnaut, M. Tsukazaki, C. J. Urch and S. M. Brown, J. Org. Chem., 1998, 63, 7576 CrossRef.
- I. E. Markó, P. R. Giles, M. Tsukazaki, I. Chélle-Regnaut, A. Gautier, S. M. Brown and C. J. Urch, J. Org. Chem., 1999, 64, 2433 CrossRef.
- M. F. Semmelhack, C. R. Schmidt, D. A. Cortés and C. S. Chou, J. Am. Chem. Soc., 1984, 106, 3374 CrossRef.
- D. Paprocki, D. Koszelewski, A. Żądło, P. Walde and R. Ostaszewski, RSC Adv., 2016, 6, 68231 RSC.
- T. Ngouansavanh and J. Zhu, Angew. Chem., Int. Ed., 2006, 45, 3495 CrossRef PubMed.
- J. Brioche, G. Masson and J. Zhu, Org. Lett., 2010, 12, 1432 CrossRef PubMed.
- G. Jiang, J. Chen, J.-S. Huang and C.-M. Che, Org. Lett., 2009, 11, 4568 CrossRef PubMed.
- T. Ngouansavanh and J. Zhu, Angew. Chem., Int. Ed., 2007, 46, 5775 CrossRef PubMed.
- F. Drouet, G. Masson and J. Zhu, Org. Lett., 2013, 15, 2854 CrossRef PubMed.
- M. Frigerio, M. Santagostino and S. Sputore, J. Org. Chem., 1999, 64, 4537 CrossRef.
- M. Fabbrini, C. Galli, P. Gentili and D. Macchitella, Tetrahedron Lett., 2001, 42, 7551 CrossRef.
- M. Gupta, S. Paul and R. Gupta, Curr. Sci., 2010, 99, 1341 Search PubMed.
- S. M. C. Pirrung and K. Das Sarma, J. Am. Chem. Soc., 2003, 126, 444 CrossRef PubMed.
- K. Atul, K. G. Maneesh and K. Mukesh, Tetrahedron Lett., 2011, 52, 4521 CrossRef.
- D. G. Blackmond, A. Armstrong, V. Coombie and A. Wells, Angew. Chem., Int. Ed., 2007, 46, 3798 CrossRef PubMed.
- T. Dwars, E. Paetzold and G. Oehme, Angew. Chem., Int. Ed., 2005, 44, 7174 CrossRef PubMed.
- P. Walde, H. Umakoshi, P. Stano and F. Mavelli, Chem. Commun., 2014, 50, 10177 RSC.
- P. Walde, Origins Life Evol. Biospheres, 2006, 36, 109 CrossRef PubMed.
- K. W. Wellington, R. Bokako, N. Raseroka and P. Steenkamp, Green Chem., 2012, 14, 2567 RSC.
- E. Brenna, M. Crotti, F. G. Gatti, D. Monti, F. Parmeggiani, A. Pugliese and F. Tentori, Green Chem., 2017, 19, 5122 RSC.
- L. Martínez-Montero, V. Gotor, V. Gotor-Fernandez and I. Lavanderá, ACS Catal., 2018, 8, 2413 CrossRef.
- Y.-Z. Qin, Y.-M. Li, M.-H. Zong, H. Wu and N. Li, Green Chem., 2015, 17, 3718 RSC.
- L. Martínez-Montero, V. Gotor, V. Gotor-Fernández and I. Lavandera, Green Chem., 2017, 19, 474 RSC.
- J. Albarrán-Velo, M. López-Iglesias, V. Gotor, V. Gotor-Fernández and I. Lavandera, RSC Adv., 2017, 7, 50459 RSC.
- P. Galletti, F. Funiciello, R. Soldati and D. Giacomini, Adv. Synth. Catal., 2015, 357, 1840 CrossRef.
- A. Madej, D. Paprocki, D. Koszelewski, A. Zadlo-Dobrowolska, A. Brzozowska, P. Walde and R. Ostaszewski, RSC Adv., 2017, 7, 33344 RSC.
- M. Fabbini, C. Galli, P. Gentili and D. Macchitella, Tetrahedron Lett., 2001, 42, 7551 CrossRef.
- S. A. Tromp, I. Matijoṧyte, R. A. Sheldon, I. W. C. E. Arends, G. Mul, M. T. Kreutzer, J. A. Moulijn and S. de Vries, ChemCatChem, 2010, 2, 827 CrossRef.
- P. Mukerjee and K. J. Mysels, Critical Micelle Concentration of Aqueous Surfactant Systems, NSRDS-NBS 36, Government Printing Office, Washington, DC, US, 1971 Search PubMed.
- O. V. Morozova, G. P. Shumakovich, S. V. Shleev and Y. I. Yaropolov, Appl. Biochem. Microbiol., 2007, 43, 583 CrossRef.
- M. J. Han, H. T. Choi and H. G. Song, J. Microbiol., 2005, 43, 555 Search PubMed.
- L. A. Beaudette, O. P. Ward, M. A. Pickard and P. M. Fedorak, Lett. Appl. Microbiol., 2000, 30, 155 CrossRef PubMed.
- J. Michizoe, H. Ichinose, N. Kamiya, T. Maruyama and M. Goto, J. Biosci. Bioeng., 2005, 99, 642 CrossRef PubMed.
- P.-P. Champagne, M. E. Nesheim and J. A. Ramsay, Enzyme Microb. Technol., 2010, 46, 147 CrossRef PubMed.
- P. Baiocco, A. M. Barreca, M. Fabbrini, C. Galli and P. Gentili, Org. Biomol. Chem., 2013, 1, 191 RSC.
- M. Fabbrini, C. Galli, P. Gentili and D. Macchitella, Tetrahedron Lett., 2001, 42, 7551 CrossRef.
- P. Galletti, F. Funiciello, R. Soldati and D. Giacomini, Adv. Synth. Catal., 2015, 357, 1840 CrossRef.
- F. Carunchio, C. Crescenzi, A. M. Girelli, A. Messina and A. M. Tarola, Talanta, 2001, 55, 189 CrossRef PubMed.
- N. Shapiro and A. Vigalok, Angew. Chem., 2008, 120, 2891 CrossRef.
- M. Mąkosza, Elsevier, 1980, 9, 1 Search PubMed.
Footnote |
† Electronic supplementary information (ESI) available. See DOI: 10.1039/c8ra04583f |
|
This journal is © The Royal Society of Chemistry 2018 |