DOI:
10.1039/C8RA04013C
(Paper)
RSC Adv., 2018,
8, 21084-21091
Application of hydrotalcite in soil immobilization of iodate (IO3−)†
Received
11th May 2018
, Accepted 5th June 2018
First published on 8th June 2018
1. Introduction
Stable iodine (127I) is considered an essential microelement for human health, with a recommended daily intake of between 80 and 150 mg per day.1 However, radioactive iodine, such as 129I, 131I, is a risk-contributing contaminant of environmental and health concern due to its easy uptake and bioaccumulation through the food chain and its high radiotoxicity. A tremendous amount of radioiodine has been released into our environment not only during atomic weapon testing, spent nuclear fuel reprocessing and nuclear accidents2,3 but also during normal operation of nuclear power plants (NPPs)4 or discharging of medical wastewater from thyroid cancer treatments.5 For example, in the 2011 Fukushima NPP accident, enormous amounts of radioactive iodine (131I) were emitted into the atmosphere and ocean.6 These long-lived radionuclides eventually move down to surface soil by wet and dry fallout or irrigation. In aqueous environments and soil systems, iodine exists mainly as iodide (I−) and iodate (IO3−).7,8 In some cases, IO3− is the predominant iodine form, which accounts for up to approximately 70% of total iodine, with iodide and organoiodine being minor components.9,10 The fate and mobility of iodine in soils depend largely on its interactions with soil components. Several recent studies reported that natural organic matter (NOM), and especially its aromatic components, played an important role in the sorption of iodine to soil and/or sediment.11–15 However, due to the lack of aromatic carbon in soil, especially soil with relatively-low organic matter, as well as its weak affinity for many geological materials, iodine species (I− and IO3−) show high mobility and, subsequently, high ecological risks.12,16–18 Therefore, scavenging materials and remediation actions are urgently needed.
In the past decade, considerable research effort has been made toward identifying natural and synthetic materials for removing or attenuating the transport of iodine in wastewater systems or aqueous solutions.4,19 In the literature, many materials were reported for the capture of I− and IO3− from aqueous systems, including claystone,20 ordinary Portland cement,21 sulfur-terminated (001) chalcopyrite surface,22 crystalline silver chloride,23 metallic oxides (such as hydrous ferric oxide (HFO) and γ-Al2O3),24 carbon-based materials (such as superfine powered activated carbon25 and biochar16), magnetite nanoparticles supported on organically modified montmorillonite (MNP-OMMTs),26 and layered double hydroxide (LDH) materials.27,28 Several groups have investigated the potential application of LDH and related materials in the removal of iodine from aqueous solutions.7,9,29–32 Theiss et al. briefly reviewed the published scientific literature, and, with further investigation, concluded that LDH is a promising iodine removal material.33
LDHs, minerals based on a brucite-like structure readily found in nature, contain exchangeable anions intercalated into the interlayer regions.9 They are represented by the following general formula:
|
[M1−x2+Mx3+(OH)2]x+[An−]x/nΔmH2O
| (1) |
where M
2+ and M
3+ are divalent (such as Mg
2+, Fe
2+ or Ni
2+) and trivalent cations (such as Al
3+, Fe
3+ or Cr
3+), respectively.
An− is the interlayer exchangeable anion (mainly nitrate or chloride), and the value of
x is equal to the molar ratio of M
3+/(M
2+ + M
3+), usually 0.2 <
x < 0.33. The presence of exchangeable
An− and variation of identities of M
2+ and M
3+ of LDH and isostructural materials give rise to potential high selectivity and the capacity to uptake the anion of interest.
9 The LDH and related materials have been widely applied to remove various anions and oxyanions, such as iodine species (I
−, IO
3−), fluorine (F
−, BF
4−), chlorine (Cl
−, ClO
4−), bromine (Br
−, BrO
3−), chromium (CrO
4−), arsenic (arsenite, arsenate), boron, anionic dye, and aniline from aqueous solutions.
33–39 The underlying mechanisms of sorption of anions by LDHs includes:
7,33 (1) anion exchange; (2) surface adsorption; and (3) reformation. Although LDH shows a relatively high potential sorption capacity for iodine anions, the presence of competing anions, including carbonate, phosphate and sulphate, has a significant impact on uptake of the target anions from an aqueous solution.
7,9,33,40 Furthermore, we must note the fact that most of the research focuses on the removal or sorption of target anions (I
− or IO
3−) from aqueous solutions. Only recently, a study utilizing fixed bed columns packed with LDH to removal fluoride exhibited a lower sorption capacity than the results reported for the corresponding batch methods.
41 Soil is a much complex system containing various coexisting anions without pH and Eh control, which may significantly affect the sorption efficiency of iodine by LDH. However, to date, little information is available on the application of LDH to immobilize iodine in soil system. Therefore, the retardation efficiency and mechanism of these target anions by LDH in soil systems needs further investigation.
In this study, the main objectives were to evaluate the immobilization of iodate by Mg–Al–NO3 LDH in a soil system by (1) examining the sorption property of iodate on Mg–Al–NO3 LDH; (2) investigating the sorption characterization of iodate in soil amended with Mg–Al–NO3 LDH in a batch system; (3) evaluating the effects of Mg–Al–NO3 LDH in the immobilization of iodate in soil using the column system.
2. Materials and methods
2.1 Materials
Magnesium nitrate hexahydrate (Mg(NO3)2·6H2O), aluminium nitrate nonahydrate (Al(NO3)3·9H2O), sodium hydroxide (NaOH), hydrochloric acid (HCl), and potassium carbonate (K2CO3) were purchased from Aladdin Reagent Co. (Shanghai). For safety, 127I in the form of potassium iodate (KIO3) was the only iodine isotope used in these experiments. All reagents were AR grade and used as received without further purification or pre-treatment.
2.2 Material preparation
The main sorbent used in this study was the 2
:
1 Mg/Al LDH, the preparation of which followed the description of Thiess et al.27 A batch of LDH with an ideal formula of Mg3Al(NO3)7 was prepared at pH 10 by coprecipitation method. Briefly, a NaOH solution (4.0 mol L−1) was added dropwise into a 150 mL solution containing Mg(NO3)2·6H2O (0.12 mol) and Al(NO3)3·9H2O (0.06 mol) with vigorous magnetic stirring under a nitrogen supply. The slurry was then refluxed at 65 °C for 24 h. The residue was separated by centrifugation and washed several times with deionized, CO2-free water. The product was dried at 60 °C in vacuum for 12 h, and then ground and sieved through a 0.1 mm-mesh sieve. In addition to the LDH described above, 3
:
1 and 4
:
1 Mg/Al LDH samples were also prepared by a similar coprecipitation method.
The soil sample used in this study was collected from an agricultural land near Qinshan Nuclear Power Plant. It has a pH of 7.7, 0.97% organic carbon content, and 9.00 cmol(+) kg−1 cation exchangeable capacity (CEC), as described in our previous work.16
2.3 Characterization of the LDH samples
The X-ray diffraction (XRD) patterns of the LDH samples were collected using an X-ray diffractometer (type XD-2600), with Cu Kα radiation at 40 keV 35 mA. The morphologies of the LDH samples were characterized using a Sigma 500 field emission scanning electron microscope (SEM) (Zeiss, Germany). The pore size distribution and pore volume of each LDH sample were also evaluated by the Brunauer–Emmett–Teller method using a gas sorption analyzer (NOVA-1200, Quantachrome Corp., USA).
2.4 Batch sorption experiments and analytical method
The sorption was conducted in triplicate by a batch equilibration technique as described in our previous work.16 Batch sorption experiments were carried out without pH control and excluding the atmosphere or dissolved carbonate, which is impractical in real world applications especially in a soil system. In the kinetic and isotherm sorption, the required portions of soil, LDHs or LDH-soil mixture (1.5 g for soil, 15 mg for LDHs, 1.5 g for LDH-soil mixture with three contents of LDH) were weighted into 22 mL centrifuge tubes containing 20 mL solutions of IO3−, with concentrations ranging from 0–200 mg L−1. Controls without sorbents were set up in parallel to account for the possible solute loss by handling and other possible ways. After shaken for a certain kinetic time or equilibrium time (4 h based on sorption kinetics) using a temperature-controlled shaker at 150 rpm at 25 °C, the suspensions were separated by centrifuging for 20 min at 4000g. The supernatants were filtrated using a 0.22 μm filter membrane (ANPEL Co., Ltd, Shanghai). The concentration of iodate in the supernatants was determined following the method described in our previous work.16 Briefly, ten millilitres of the aqueous sample was mixed with 0.5 mL of 6.0 mol L−1 HCl. Then, 0.5 mL of 0.1 mol L−1 K2CO3 was added and fully mixed. After keeping the sample still for 10 min, it was measured with a spectrophotometer (Shimadzu UV-Vis 2600, Japan) at a 352 nm wavelength. The limit of detection for IO3− was 0.6 mg L−1.
The sorption of iodate by soil and LDH was determined using the following equation:
|
 | (2) |
where
Ci and
Ct (mg L
−1) represent the initial and final (at any time
t) concentrations of iodate. The sorption capacity of the soil or LDH for iodate at time
t,
Qt (mg kg
−1), was obtained as follows:
|
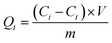 | (3) |
where
V (mL) was the volume of the solution, which equalled 20 mL in the present study;
m (mg) represents the mass of sorbents and used 1500 mg of soil with and without LDH.
To determine the sorption kinetics, the obtained dynamic experimental data was fitted with the pseudo-second-order model, which can be written as follows:
|
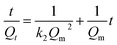 | (4) |
where
k2 was the pseudo-second-order rate constant (g mg
−1 min
−1). As time approaches zero, according to the pseudo-second-order model, the initial sorption rate
h (mg g
−1 min
−1) was calculated as follows:
The logarithmic form of the Freundlich model (original form: Qe = KFCe1/n) was used to calculated the Freundlich parameters and is expressed as shown in the following eqn (6):
|
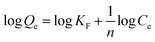 | (6) |
where
Qe is the amount adsorbed per unit weight of sorbent, mg kg
−1;
Ce is the equilibrium concentration, mg L
−1;
KF [(mg kg
−1) (mg L
−1)
−1, equal to L kg
−1] and
n (dimensionless) are the Freundlich isotherm constants, describing the sorption capacity and the isotherm curvature.
2.5 Column experiments
The soil mixed with LDH was packed in a glass column (45 mm inner diameter, 250 mm height). The portions of LDH amended in the soil were 0, 0.66%, 1.0%, and 1.33%. Two hundred grams of the soil-LDH mixture was gently placed in the column and the height of the beds was approximately 120 mm, with a 10 mm quartz sand layer below and above the soil-LDH layer. Twenty millilitres of 200 mg L−1 KIO3 solution was added to the column from the top. Water was introduced at a constant volumetric flow rate of 2 mL min−1 to drain off the entire KIO3 solution as much as possible. Fifteen liquid samples of 10 mL were withdrawn. The collected pore water was immediately filtered by a 0.45 μm membrane filter (ANPEL Co., Ltd., China) and iodate concentrations were analysed as described above.
3. Results and discussion
3.1 Characterization of the LDH sorbents
Powder XRD patterns of three Mg–Al–NO3 LDHs with different cation ratios were collected and are presented in Fig. 1. The four major peaks of all the three LDHs are located at approximately 11.5°, 22.9°, 34.6°, and 39.0°, which can be indexed to the (003), (006), (012), and (015) planes of Mg–Al hydrotalcite (JCPDS 35-0965).37 The positions of the key peaks of all three materials (2
:
1, 3
:
1, and 4
:
1 Mg–Al–NO3 LDH) showed no notable shift and no additional phases were observed, indicating that the samples prepared did indeed contain Mg–Al–NO3 LDH materials. The power XRD pattern intensities of the samples shown in Fig. 1 were not scaled but an indicator of the relative crystallinity was provided. The intensities of the key peaks, including d003 and d006, increased along with the increase of the cation ratio, indicating higher crystallization with a higher cation ratio (e.g., 4
:
1 Mg–Al–NO3 LDH). However, all the key peaks of the three LDHs were sufficiently narrow and intense and these results verified the successful crystallization.27
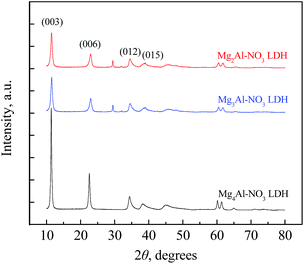 |
| Fig. 1 XRD patterns of three MgAl–NO3 LDH samples. | |
SEM images of the three Mg–Al–NO3 LDH samples showed agglomeration of platelets of irregular size and shape and were significantly affected by the cation ratio (Fig. 2). A layered structure was observed in 2
:
1 Mg–Al–NO3 LDH (Fig. 2A), while the SEM image of the 4
:
1 Mg–Al–NO3 LDH sample displayed microcrystalline granules embedded onto the heterogeneous matrix (Fig. 2C). The microscopy morphology results are consistent with the X-ray diffractograms and demonstrated that the relatively less crystalline heterogeneous phase in 2
:
1 Mg–Al–NO3 LDH was more consistent with a hydrotalcite-like structure.9 The average pore size distribution was approximately 10 nm (data not shown).
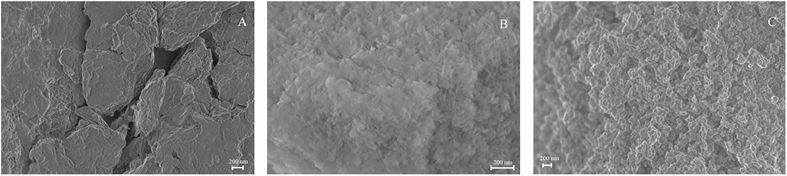 |
| Fig. 2 SEM images of MgAl–NO3 LDH samples with different Mg/Al ratios of 2 (A), 3 (B), and 4 (C). | |
3.2 Removal of iodate (IO3−) from aqueous solution by LDH
To evaluate the ability of Mg–Al–NO3 LDH to adsorb IO3−, as well as the effect of the cation (Mg/Al) ratio on the sorption of IO3−, batch studies were performed and the results are presented in Fig. 3. The M2+
:
M3+ cation ratio of the LDH is an important factor that influences anion uptake. The Mg/Al ratio significantly affected the iodate uptake, due to different charge density.33 The greatest removal of IO3− from an aqueous solution was 57.0% by 2
:
1 Mg–Al–NO3 LDH, while iodate uptake of 40.5% and 28.0% were observed when using 3
:
1 and 4
:
1 Mg–Al–NO3 LDH, respectively. However, the results in the present experiment appear inconsistent with those reported by Toraishi et al.,42 where the 3
:
1 LDH was found to be the optimal cation ratio for iodate sorption. It is interesting to note that some studies reported that the 4
:
1 LDH was preferable for iodide sorption.30,31 It is reasonable that the 2
:
1 LDH contains more trivalent aluminium ions and high charge density in the substituted brucite layers, which could accommodate more IO3− compared to the 3
:
1 and 4
:
1 LDH.27 In addition, the initial iodate concentration, LDH dosage, and temperature influenced the sorption efficiency of IO3− from the aqueous solution (shown in Fig. S1–S3†). In the present condition (200 mg L−1 of initial iodate concentration, 0.75 g L−1 of LDH dosage, and 25 °C), the removal efficiency of IO3− by Mg2–Al–NO3 LDH was comparable or even higher than that previously reported.9,36
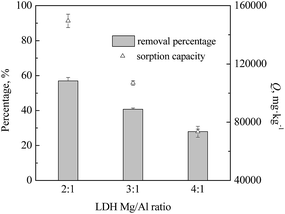 |
| Fig. 3 Effect of the cation ratio on the sorption of IO3− by Mg–Al–NO3 LDHs. | |
All the three Mg–Al–NO3 LDHs showed large iodate sorption capacities, as shown in Fig. 3. Similarly, the 2
:
1 Mg–Al–NO3 LDH showed the largest sorption capacity of 149
528 mg IO3− kg−1, followed by 106
882 and 73
414.3 mg kg−1 for the 3
:
1 and 4
:
1 Mg–Al–NO3 LDH, respectively. Furthermore, Mg–Al–NO3 LDH had a much greater sorption capacity for anions, compared to Ni–Al-LDH and Zn–Al-LDH.9,34 Therefore, the 2
:
1 Mg–Al–NO3 LDH was chosen to use in the majority of the following immobilization studies.
3.3 Effects of Mg2–Al–NO3 LDH on the soil sorption of IO3− in the batch system
Fig. 4A shows the kinetic sorption behaviour of iodate ions by soil, Mg2–Al–NO3 LDH, and soil amended with 1% of LDH. The iodate sorption by all three sorbents exhibited a biphasic phenomenon: a steep initial ascending trend of sorption capacity (Qt), followed by a steady but slower accumulation.
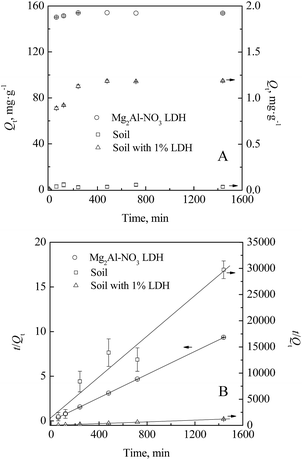 |
| Fig. 4 Kinetic sorption of IO3− by soil in the absence and presence of Mg2Al–NO3 LDH: (A) sorption capacity; (B) simulation with the pseudo-second-order model. | |
The equilibriums were achieved in less than 240 minutes for soil, soil amended with LDH and pure Mg2–Al–NO3 LDH. The soil has a very weak affinity, with the sorption capacity of 59.2 mg kg−1, which was consistent with previous observations and follows the range of 25–70 mg kg−1 reported in the literature.16,18,43 The Mg2–Al–NO3 LDH showed an enormous maximum sorption capacity, up to 154.2 mg g−1, which is more than 2600 times higher than that of the soil. Therefore, with the addition of only 1% of Mg2–Al–NO3 LDH to soil, the sorption capacity can reach 20 times higher (1187 mg kg−1) than that of soil.
The pseudo-second-order model has been applied to the kinetic sorption data shown Fig. 4B. The calculated constants (k2, Qm, h) as well as the regression coefficients (R2) are listed in Table 1 and demonstrate that the experimental data fit well to the pseudo-second-order model. It was surprising that the Mg2–Al–NO3 LDH had the lowest pseudo-second-order rate constant (k2) of 0.00768 g mg−1 min−1, while the greatest k2 was obtained in the soil system (0.25307 g mg−1 min−1). This is probably due to the quite small iodate sorption capacity (Qm, 0.05142 g g−1) of soil, which is easily reaches equilibrium. Instead, the initial sorption rate h might be a better parameter to reveal the kinetics of iodate sorption. As seen in Table 1, it's much more reasonable that the calculated h followed the order: Mg2–Al–NO3 LDH > soil amended with 1% LDH > soil, with h values of 0.000669, 0.0552, and 182 mg g−1 min−1. Moreover, the values of Qm calculated from the pseudo-second-order model are very close to the measured values. The pseudo-second-order model is based on the assumption that chemisorption is the rate-limiting step, involving valence forces through the sharing or exchange of electrons between sorbent and sorbate.41 Therefore, the observations in this study indicated that the second order adoption reaction and diffusion process may be the limiting step.29
Table 1 Kinetic parameters of iodate onto the LDH, soil and soil-LDH mixture using the pseudo-second order model
Sorbent |
k2, g mg−1 min−1 |
Qm, mg g−1 |
h, mg g−1 min−1 |
R2 |
Mg2Al–NO3 LDH |
0.00768 |
153.8 |
182 |
0.9999 |
Soil |
0.25307 |
0.05142 |
0.000669 |
0.9499 |
Soil with 1% LDH |
0.03769 |
1.210 |
0.0552 |
0.9998 |
Adsorption isotherms were analysed to investigate the adsorption capacity and affinity of LDH and soil with IO3− at different equilibrium concentrations and shown in Fig. 5. Again, the Mg2–Al–NO3 LDH showed a larger iodate sorption amount than that of the soil (Fig. 5A). With the increase of the addition portion of LDH in the soil (0.66% to 1.33%), the sorption capacity subsequently increased. The isothermal sorption data of IO3− onto the soil, Mg2–Al–NO3 LDH, and their mixtures were plotted and fitted well to the Freundlich model (Fig. 5B). The calculated Freundlich parameters are summarized in Table 2. The Mg2–Al–NO3 LDH demonstrated very high affinity for IO3− (KF reaches 21
380 L kg−1), which is much higher than the ones reported in other studies.9 It is reasonable to note that the high removal of IO3− from the aqueous solution was observed across the initial test concentration range (40–300 mg L−1) (Fig. S1†). Moreover, the addition of Mg2–Al–NO3 LDH in the soil at a relatively low dosage also exhibited a higher sorption affinity for IO3−, compared with the soil (KF value of 4.74 L kg−1). The KF values were 13.1, 26.4, and 136 L kg−1 for 0.66%, 1%, and 1.33% additions of LDH, respectively.
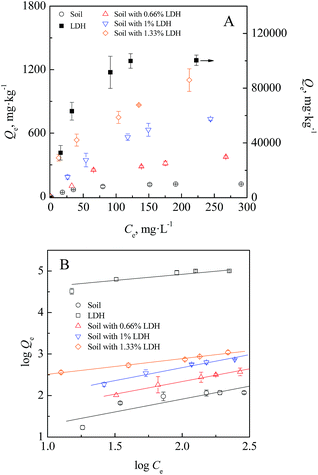 |
| Fig. 5 Isotherm sorption of IO3− by soil in the absence and presence of Mg2Al–NO3 LDH: (A) sorption capacity; (B) stimulated with the Freundlich model. | |
Table 2 Isothermal sorption parameters of iodate onto soil with and without LDHa
Sorbent |
Freundlich parameters |
KF*/KF, soil |
KF, L kg−1 |
n |
R2 |
KF*: Freundlich isothermal sorption parameter of iodate for LDH and soil mixed with LDH, KF, soil: Freundlich isothermal sorption parameter of iodate for soil. |
Soil |
4.7402 |
1.61 |
0.7616 |
|
LDH |
21 380 |
3.38 |
0.8864 |
4510 |
Soil with 0.66% LDH |
13.086 |
1.64 |
0.9845 |
2.761 |
Soil with 1% LDH |
26.363 |
1.60 |
0.9730 |
5.562 |
Soil with 1.33% LDH |
135.55 |
2.64 |
0.9900 |
28.60 |
More importantly, the ratio between the KF values of Mg2–Al–NO3 LDH and that of the soil (KF*/KF, soil), representing the enhancement level of iodate sorption affinity of sorbent as a soil amendment, was quite large, i.e., 4510 for IO3−. In our previous work, biochar was used as an effective soil amendment to immobilize iodide and iodate in arable land soil, due to the specific I–C interaction of the iodine anion (I− and IO3−) with the aromatic structure of biochar.11,16 Many studies have reported that various LDH types had high affinity for iodine anions through anion exchange, surface adsorption, and the reconstruction effect.4,27–29,33,36 Given the high potential immobilization ability of LDH, we applied 0.66%, 1%, and 1.33% of Mg2–Al–NO3 LDH to immobilize the behaviour of iodate in the soil system. The results exhibited that the addition of LDH could significantly enhance the sorption affinity of soil, with KF*/KF, soil values of 2.761, 5.562, and 28.60. The retarding effects of LDH on the transport behaviour of iodate in soil were further analysed in continuous systems.
3.4 Effects of Mg2–Al–NO3 LDH on the soil immobilization of IO3− in the continuous systems
Laboratory column flushing was conducted to evaluate the performance of Mg2–Al–NO3 LDH in retarding soil IO3−. The instantaneous effluent concentration of IO3− from soil columns with different Mg2–Al–NO3 LDH additions are plotted in Fig. 6A versus the elution volume. With an increasing elution volume, a similar tendency of the effluent iodate concentration was shared by all the four soil-LDH mixture systems. That is, a sharp increase of iodate concentration to maximum value in the outlet is observed, followed a gradual decrease to a relatively low level. However, the addition of the Mg2–Al–NO3 LDH postponed the appearance of the effluent peak and sharply decreased the maximum concentration. Additionally, it can be seen that the changes had an apparent dependence on the portion of Mg2–Al–NO3 LDH. For example, the maximum value of effluent iodate (59 mg L−1) appeared at the volume of 20 mL in soil, while in soil amended with 1.33% of Mg2–Al–NO3 LDH, it reached a maximum value of 6.08 mg L−1 at the volume of 110 mL. The results can be explained by the mass transfer phenomena that takes place in the column flushing. The added Mg2–Al–NO3 LDH improved the affinity of soil with IO3− as mentioned above, which reduced the desorption of IO3− from the solid matrix. The eluted amount of IO3− versus the total amount of IO3− (m/m0) were also calculated and are shown in Fig. 6B. Similarly, the m/m0 values in all systems increased sharply and gradually decreased to a relatively small level.
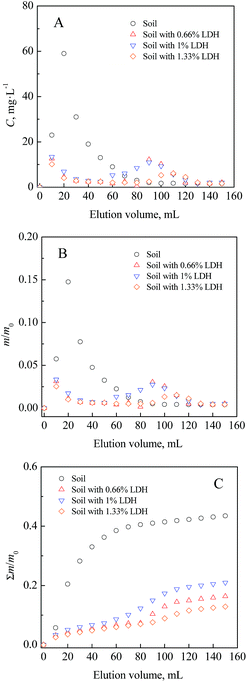 |
| Fig. 6 Effects of the Mg2Al–NO3 LDH addition on the IO3− concentration: (A) instantaneous effluent concentration; (B) accumulated IO3− amount; (C) total amount of eluted IO3−. | |
Moreover, the total eluted percentage (%) of IO3− was calculated using the total eluted amount of IO3− versus the total amount of IO3− (Σm/m0), which is illustrated in Fig. 6C. The application of Mg2–Al–NO3 LDH significantly retarded the mobility of iodate in soil. The eluted percentage of IO3− reduced with the increase of added Mg2–Al–NO3 LDH, from 43.5% in soil to 21.0%, 16.4%, and 12.9% for 0.66%, 1%, and 1.33% LDH additions, respectively. Additionally, the increase in elution volume (or time) of treated solution to reach a stable maximum was also observed when the LDH addition increased. For instance, it only needs a 60 mL solution to wash out more than 40% of the IO3− in the soil system; however, more than 110 mL of solution is needed to achieve the flushing plateau with the addition of 1% Mg2–Al–NO3 LDH. Soil was considered a weak matrix to maintain iodine species such as iodide and iodate.12,16–18 In the literature, many materials have been synthetized to capture these mobile compounds from aqueous solutions.16,22–28 However, the efficiencies of these excellent materials should be further examined in soil due to the interferences of variables in real world applications.27 For example, affinity parameters were obtained ranged from 363 to 2240 L kg−1 by Co–Cr and Ni–Cr hydrotalcite,9 and 600 to 900 L kg−1 by microporous acetyl cellulose membrane.4 The efficiency in the column study was comparable to that obtained in the batch experiments, without obvious adverse effects by the soil variables, such as coexisting ions and soil pH. The results indicated that Mg2–Al–NO3 LDH could potentially be applied to effectively immobilize iodate in soil.
4. Conclusions
The Mg2–Al–NO3 LDH has been successfully synthesized and applied to immobilize iodate in soil in both the batch and column systems. The Mg2–Al–NO3 LDH demonstrated a strong affinity for IO3−, with a high removal efficiency of 57.0% and a sorption capacity of 149
528 mg kg−1. The cation ratio (Mg
:
Al in this study) was an important factor influencing the iodate sorption capacity. The capacities were 106
882 and 73
414.3 mg kg−1 for the 3
:
1 and 4
:
1 Mg–Al–NO3 LDH samples, respectively, which was much lower than that of the 2
:
1 LDH. The addition of Mg2–Al–NO3 LDH in soil with different portions resulted in significant retention of IO3− in both the batch and column experiments. For example, the affinity parameter KF of soil with 1.33% Mg2–Al–NO3 LDH added was 136 L kg−1, which was 28.6 times higher than soil without an LDH addition. Moreover, the eluted iodate percentage was only 12.9% in the soil column with the 1.33% Mg2–Al–NO3 LDH addition, whereas almost 43.5% iodate was washed out in the soil column without LDH addition. The results suggested that LDH could effectively immobilize iodate in soil without obvious interference by coexist ions.
Conflicts of interest
There are no conflicts to declare.
Acknowledgements
This work was supported by the National Natural Science Foundation of China (21407037 and 41271249), Project of the Natural Science Foundation of Zhejiang Province (LQ14B070006), and the Special Funding for Introduced Innovative R&D Team of Dongguan (2014607101003).
References
- Guidelines for drinking-water quality, WHO press, World Health Organization, Geneva, Switzerland, 2011 Search PubMed.
- S. Xu, S. Freeman, X. Hou, A. Watanabe, K. Yamaguchi and L. Zhang, Iodine isotopes in precipitation: temporal responses to 129I emissions from the Fukushima nuclear accident, Environ. Sci. Technol., 2013, 47, 10851–10859 CrossRef PubMed.
- X. Hou, A. Aldahan, S. Nielsen and G. Possnert, Time series of 129I and 127I speciation in precipitation from Denmark, Environ. Sci. Technol., 2009, 43, 6522–6528 CrossRef PubMed.
- S. Kulyukhin, Fundamental and applied aspects of the chemistry of radioactive iodine in gas and aqueous media, Russ. Chem. Rev., 2012, 81, 960 CrossRef.
- P. Rose, R. Swanson and J. Cochran, Medically-derived 131I in municipal sewage effluent, Water Res., 2012, 46, 5663–5671 CrossRef PubMed.
- K. Hirose, 2011 Fukushima Dai-ichi nuclear power plant accident: summary of regional radioactive deposition monitoring results, J. Environ. Radioact., 2012, 111, 13–17 CrossRef PubMed.
- L. Iglesias, C. Walther, F. Medina, A. Hölzer, A. Neumann, M. Lozano-Rodriguez, M. Álvarez and N. Torpapava, A comprehensive study on iodine uptake by selected LDH phases via coprecipitation, anionic exchange and reconstruction, J. Radioanal. Nucl. Chem., 2016, 307, 111–121 CrossRef.
- X. Hou, V. Hansen, A. Aldahan, G. Possnert, O. Lind and G. Lujaniene, A review on speciation of iodine-129 in the environmental and biological samples, Anal. Chim. Acta, 2009, 632, 181–196 CrossRef PubMed.
- T. Levitskaia, S. Chatterjee, B. Arey, E. Campbell, Y. Hong, L. Kovarik, J. Peterson, N. Pence, J. Romero, V. Shutthanandan, B. Schwenzer and T. Varga, RedOx-controlled sorption of iodine anions by hydrotalcite composites, RSC Adv., 2016, 6, 76042–76055 RSC.
- D. Kaplan, M. Denham, S. Zhang, C. Yeager, C. Xu, K. Schwehr, H. Li, F. Ho, D. Wellman and P. Santschi, Radioiodine biogeochemistry and prevalence in groundwater, Crit. Rev. Environ. Sci. Technol., 2014, 44, 2287–2335 CrossRef PubMed.
- S. Choung, W. Um, M. Kim and M. Kim, Uptake mechanism for iodine species to black carbon, Environ. Sci. Technol., 2013, 47, 10349–10355 Search PubMed.
- C. Xu, E. Miller, S. Zhang, H. Li, Y. Ho, K. Schwehr, D. Kaplan, S. Otosaka, K. Roberts, R. Brinkmeyer, C. Yeager and P. Santschi, Sequestration and remobilization of radioiodine (129I) by soil organic matter and possible consequences of the remedial action at Savannah River Site, Environ. Sci. Technol., 2011, 45, 9975–9983 CrossRef PubMed.
- C. Xu, S. Zhang, Y. Sugiyama, N. Ohte, Y. Ho, N. Fujitake, D. Kaplan, C. Yeager, K. Schwehr and P. Santschi, Role of natural organic matter on iodine and 239,240Pu distribution and mobility in environmental samples from the northwestern Fukushima Prefecture, Japan, J. Environ. Radioact., 2016, 153, 156–166 CrossRef PubMed.
- P. Santschi, C. Xu, S. Zhang, K. Schwehr, R. Grandbois, D. Kaplan and C. Yeager, Iodine and plutonium association with natural organic matter: a review of recent advances, Appl. Geochem., 2017, 85, 121–127 CrossRef.
- Y. Unno, H. Tsukada, A. Takeda, Y. Takaku and S. Hisamatsu, Soil–soil solution distribution coefficient of soil organic matter is a key factor for that of radioiodide in surface and subsurface soils, J. Environ. Radioact., 2017, 169–170, 131–136 CrossRef PubMed.
- D. Zhang, L. Lu, T. Lü, M. Jin, J. Lin, X. Liu and H. Zhao, Application of a rice husk-derived biochar in soil immobilization of iodide (I−) and iodate (IO3−), J. Environ. Radioact., 2018, 18, 1540–1547 Search PubMed.
- G. Lefèvre, J. Bessière, J. Ehrhardt and A. Walcarius, Immobilization of iodide on copper(I) sulfide minerals, J. Environ. Radioact., 2003, 70, 73–83 CrossRef.
- W. Shetaya, S. Young, M. Watts, E. Ander and E. Bailey, Iodine dynamics in soils, Geochim. Cosmochim. Acta, 2012, 77, 457–473 CrossRef.
- B. Riley, J. Vienna, D. Strachan, J. McCloy and J. Jerden, Materials and processes for the effective capture and immobilization of radioiodine: a review, J. Nucl. Mater., 2016, 470, 307–326 CrossRef.
- S. Savoye, B. Frasca, B. Grenut and A. Fayette, How mobile is iodide in the Callovo–Oxfordian claystones under experimental conditions close to the in situ ones?, J. Contam. Hydrol., 2012, 142–143, 82–92 CrossRef PubMed.
- M. Atkins and F. Glasser, Application of Portland cement-based materials to radioactive waste immobilization, Waste Management, 1992, 12, 105–131 CrossRef.
- K. Li, Y. Zhao, P. Zhang, C. He, J. Deng, S. Ding and W. Shi, Combined DFT and XPS investigation of iodine anions adsorption on the sulfur termination (001) chalcopyrite surface, Appl. Surf. Sci., 2016, 390, 412–421 CrossRef.
- M. Mostafa, H. Ramadan and M. El-Amir, Sorption and desorption studies of radioiodine onto silver chloride via batch equilibration with its aqueous media, J. Environ. Radioact., 2015, 150, 9–19 CrossRef PubMed.
- T. Nagata and K. Fukushi, Prediction of iodate adsorption and surface speciation on oxides by surface complexation modeling, Geochim. Cosmochim. Acta, 2010, 74, 6000–6013 CrossRef.
- M. Ikari, Y. Matsui, Y. Suzuki, T. Matsushita and N. Shirasaki, Removal of iodide from water by chlorination and subsequent adsorption on powered activated carbon, Water Res., 2015, 68, 227–237 CrossRef PubMed.
- J. Jiang and D. Lee, Magnetite nanoparticles supported on organically modified montmorillonite for adsorptive removal of iodide from aqueous solution: optimization using response surface methodology, Sci. Total Environ., 2018, 615, 549–557 CrossRef PubMed.
- F. Theiss, G. Ayoko and R. Frost, iodide removal using LDH technology, Chem. Eng. J., 2016, 296, 300–309 CrossRef.
- F. Theiss, G. Ayoko and R. Frost, Leaching of iodide (I−) and iodate (IO3−) anions from synthetic layered double hydroxide materials, J. Colloid Interface Sci., 2016, 478, 311–315 CrossRef PubMed.
- F. Theiss, G. Ayoko and R. Frost, Sorption of iodide (I−) from aqueous solution using Mg/Al layered double hydroxides, Mater. Sci. Eng., C, 2017, 77, 1228–1234 CrossRef PubMed.
- G. Fetter, E. Ramos, M. Olguin, P. Bosch, T. López and S. Bulbulian, Sorption of 131I− by hydrotalcites, J. Radioanal. Nucl. Chem., 1997, 221, 63–66 CrossRef.
- L. Linag and L. Li, Adsorption behavior of calcined layered double hydroxides towards removal of iodide contaminants, J. Radioanal. Nucl. Chem., 2006, 273, 221–226 CrossRef.
- T. Toraishi, S. Nagasaki and S. Tanaka, Adsorption behavior of IO3− by CO32− and NO3−-hydrotalcite, Appl. Clay Sci., 2002, 22, 17–23 CrossRef.
- F. Theiss, S. Couperthwaite, G. Ayoko and R. Frost, A review of the removal of anions and oxyanions of the halogen elements from aqueous solution by layered double hydroxides, J. Colloid Interface Sci., 2014, 417, 356–368 CrossRef PubMed.
- L. Lv, J. He, M. Wei, D. Evans and X. Duan, Factors influencing the removal of fluoride from aqueous solution by calcined Mg–Al–CO3 layered double hydroxides, J. Hazard. Mater., 2006, 133, 119–128 CrossRef PubMed.
- A. Ay, B. Zümreoglu-Karan and A. Temel, Boron removal by hydrotalcite-like, carbonate-free Mg–Al–NO3-LDH and a rationale on the mechanism, Microporous Mesoporous Mater., 2007, 98, 1–5 CrossRef.
- S. Yu, X. Wang, Z. Chen, J. Wang, S. Wang, T. Hayat and X. Wang, Layered double hydroxide intercalated with aromatic acid anions for the efficient capture of aniline from aqueous solution, J. Hazard. Mater., 2017, 321, 111–120 CrossRef PubMed.
- C. Lei, X. Zhu, B. Zhu, C. Jiang, Y. Le and J. Yu, Superb adsorption capacity of hierarchical calcined Ni/Mg/Al layered double hydroxides for Congo red and Cr(VI) ions, J. Hazard. Mater., 2017, 321, 801–811 CrossRef PubMed.
- D. Tang and G. Zhang, Efficient removal of fluoride by hierarchical Ce–Fe bimetal oxides adsorbent: thermodynamics, kinetics and mechanism, Chem. Eng. J., 2016, 283, 721–729 CrossRef.
- L. Yang, Z. Shahrivari, P. Liu, M. Sahimi and T. Tsotsis, Removal of trace levels of arsenic and selenium from aqueous solutions by calcined and uncalcined layered double hydroxides (LDH), Ind. Eng. Chem. Res., 2005, 44, 6804–6815 CrossRef.
- B. Hunter, W. Hieringer, J. Winkler, H. Gray and A. Müller, Effect of interlayer anions on [NiFe]-LDH nanosheet water oxidation activity, Energy Environ. Sci., 2016, 9, 1734 Search PubMed.
- M. Jiménez-Núñez, M. Solache-Ríos and M. Olguín, Fluoride sorption from aqueous solutions and drinking water by magnesium, cobalt, and nickel hydrotalcite-like compounds in batch and column systems, Sep. Sci. Technol., 2010, 45, 786–793 CrossRef.
- T. Toraishi, S. Nagasaki and S. Tanaka, Adsorption behavior of IO3− by CO32− and NO3−- hydrotalcite, Appl. Clay Sci., 2002, 22, 17–23 CrossRef.
- C. Hong, H. Weng, G. Jilani, A. Yan, H. Liu and Z. Xue, Evaluation of iodide and iodate for adsorption–desorption characteristics and bioavailability in three types of soil, Biol. Trace Elem. Res., 2012, 146, 262–271 CrossRef PubMed.
Footnote |
† Electronic supplementary information (ESI) available. See DOI: 10.1039/c8ra04013c |
|
This journal is © The Royal Society of Chemistry 2018 |