DOI:
10.1039/C8RA03704C
(Paper)
RSC Adv., 2018,
8, 21637-21643
Increased effective mass and carrier concentration responsible for the improved thermoelectric performance of the nominal compound Cu2Ga4Te7 with Sb substitution for Cu†
Received
30th April 2018
, Accepted 25th May 2018
First published on 14th June 2018
Abstract
Although the ternary chalcopyrite compound Cu2Ga4Te7 has relatively high thermal conductivity and electrical resistivity, it has a high carrier concentration, thus making it a good thermoelectric candidate. In this work we substitute Sb for Cu in this compound, aiming at engineering both the electrical and thermal properties. Rietveld refinement revealed that the nominal compounds Cu2−xSbxGa4Te7 (x = 0–0.1) crystallize with the crystal structure of CuGaTe2 with the real compositions deviating from those of their nominal ones. Besides, Sb resides in Cu sites, which increases both the effective mass and the Hall carrier concentration. Therefore, the Seebeck coefficient increases at high temperatures, and the lattice thermal conductivity reduces due to increased phonon scattering from point defects and electron–phonon interactions. As a consequence, the thermoelectric (TE) performance improves with the highest TE figure of merit (ZT) of 0.58 at 803 K. This value is about 0.21 higher than that of the pristine Cu2Ga4Te7.
1. Introduction
Thermoelectric (TE) materials can directly convert heat into electricity and vice versa. The efficiency of TE devices is strongly dependent on the performance of materials, i.e. the dimensionless figure of merit (ZT), which is defined by the relation, ZT = Tα2σ/κ. Here the parameters T, α, σ and κ are the absolute temperature, the Seebeck coefficient, and the electrical and total thermal conductivity respectively. In order to enhance the ZT value, one should increase the power factor PF, PF = α2σ, and reduce the κ value that is mainly contributed by the lattice (κL) and electronic (κe) components. Since the three physical parameters α, σ and κe are closely related to the carrier concentration, it is not easy to control them separately. The strategies to enhance the ZT value proposed in recent years are those like nanostructure1–3 and band structure engineering,4–6 liquid-like thermoelectric explorations,7,8 as well as the study of magnetoelectric interactions,9,10 etc. These approaches either improve the power factor (α2σ) or reduce the lattice component (κL), provided that the carrier concentration is optimized.11 In addition to the above approaches, there is a strong need to develop new TE materials.
Ternary I–III–VI compounds have been paid much attention in recent years for thermoelectric applications.12–14 Owing to their inherent crystal or band structures,15–17 one often employs approaches, such as doping or solid solution formation, to improve their TE performances.18–20 The typical doping elements are those such as Ag,14,20 Sb,21,22 Mn19 etc., since impurity doping effectively engineers the band structures and introduces lattice disorder, thus increasing the carrier concentration and phonon scattering.
Cu2Ga4Te7 is one of the I–III–VI ternary compounds with two crystal structures. One is cubic (zinc blende) and the other is a tetragonal (chalcopyrite) structure.23 Because of the one-seventh cation vacancies in its unit cell, this compound is usually a p-type semiconductor with a Hall carrier concentration (nH) of 1.0 × 1018 to 8.3 × 1019 cm−3.24,25 Although the nH value is close to the optimal one with respect to the TE performance,26 the compound Cu2Ga4Te7 has a relatively high electrical resistivity and thermal conductivity.24,25 It was reported that the highest ZT value of Cu2Ga4Te7 is less than 0.47 at ∼770 K,25,27 and 0.64 at 940 K.28 Therefore, there is a requirement to further improve its TE performance.
Inspired by an effective hybridization of active Sb-5p orbital with those of Cu-4s and Te-5p in the valence band in the newly developed Cu-deficient Cu18Ga25Te50 (cation/anion = 0.86),21 which unpins the Fermi level and enhances the carrier concentration,21 we postulate that an incorporation of Sb in Cu2Ga4Te7 with an almost identical cation/anion ratio (0.857) might also have a profound impact on the structure and transport properties. However, unlike a proper replacement of Sb for Te in Cu18Ga25Te50,21 in this work we design the chemical compositions with a replacement of Sb for Cu in Cu2Ga4Te7, to gain further insight into the potential effect on physical properties. Through Sb replacement, the nominal compounds Cu2−xSbxGa4Te7 crystallize with the crystal structure of CuGaTe2 with the real compositions deviating from those of their nominal ones. Besides, an addition of Sb increases the effective mass (m*) of the carrier. Coupled with the enhancement in carrier concentration and phonon–electron interactions, the TE performance was improved.
2. Experimental
2.1 Sample preparation
Four elements (Cu, Ga, Te, and Sb) (Emei Semicon. Mater. Co., Ltd. Sichuan, CN), with purities of more than 99.999%, were loaded into different vacuum silica tubes according to the formula Cu2−xSbxGa4Te7 (x = 0.05, 0.1, 0.2) and then melted at 1373 K. When they were melted, the samples were rocked for 30 s every 1 h to ensure a homogeneous composition without segregation. After cooling down from 1373 K to room temperature (RT), the solidified ingots were pulverized and then ball-milled at a rotation rate of 350 rpm for 5 h in stainless steel bowls that contained benzinum. Subsequently, the dried powder was quickly sintered by using spark plasma sintering apparatus (SPS-1030) at a peak temperature of 823 K and a pressure of 55 MPa. The holding time at 823 K was controlled to be ∼2 min. The densities (d) of the polished bulks, which were more than 95% of the theoretical density (5.84 g cm−3),24 were measured using Archimedes’ method. Pristine Cu2Ga4Te7 (x = 0) was also prepared for comparison.
Bulk samples with sizes of 2.5 × 3 × 12 mm3 and 2 × 2 × 7 mm3 were prepared for the measurement of electrical properties and Hall coefficients respectively, and those of ϕ 10 × 1.5 mm2 for thermal diffusivity measurements.
2.2 Physical property measurements
The physical parameters, which involve Seebeck coefficients (α) and electrical conductivities (σ) as a function of temperature, were measured under a helium atmosphere from RT to ∼805 K in a ULVAC ZEM-3 instrument system with an uncertainty of 6.0% for each. The thermal diffusivities were measured by using TC-1200RH apparatus from RT to ∼ 805 K. Owing to the lower than RT Debye temperature of Cu2Ga4Te7 (222 K, ref. 28), the Dulong–Petit rule is viable to estimate the heat capacities (Cp) above RT.23 The thermal conductivities (κ) were then directly calculated as the products of material densities (d), specific heats (Cp) and thermal diffusivities (κ). The lattice contributions (κL) were obtained by subtracting the electronic part (κe) from the total κ, i.e., κL = κ − κe, here κe is expressed by the Wiedemann–Franz law, κe = L0σT, where L0 is the Lorenz number, estimated at 2.45 × 10−8 W Ω K−2 for degenerate environments of semiconductors.29 The three parameters (α, σ, and κ) were finalized by taking the average values of several samples tested by the same method.
Hall coefficients (RH) were measured by using a four-probe configuration in a system (PPMS, Model-9) with a magnetic field up to ±2 T. The Hall mobility (μ) and carrier concentration (nH) were calculated according to the relations μ = |RH|σ and nH = 1/(eRH) respectively, where e is the electron charge.
2.3 Chemical compositions and structural analyses
Structural analysis of the powders was made by using a powder X-ray diffractometer (D8 Advance) operating at 50 kV and 40 mA with Cu Kα radiation (λ = 0.15406 nm) in the range from 10° to 110° with a step size of 0.02°, and an X’Pert Pro, PANalytical code was used to do the Rietveld refinement of the XRD patterns of the titled compounds. The lattice constants a and c were directly obtained from the refinement of the XRD patterns using Jade software.
The chemical compositions of the samples Cu2−xSbxGa4Te7 (x = 0, 0.2) were checked using an electron probe micro-analyzer (EPMA) (S-4800, Hitachi, Japan) with an accuracy of >97%.
3. Results and discussions
3.1 Composition analyses and XRD
Fig. S1† shows the EMPA mappings of four elements, Cu, Sb, Te and Ga, for the sample at x = 0.2. The average chemical compositions of stoichiometric Cu2Ga4Te7 and Cu1.8Ga4Sb0.2Te7 are shown in Table S1,† where the number of moles of Te was normalized to 7.0. Generally, the relative molar fractions shown in Table S1† are close to those of the nominal ones, and the four elements are distributed relatively uniformly in the matrix without much segregation, indicating that the titled materials were well prepared.
The Rietveld refinement using the XRD data of three compounds Cu2−xSbxGa4Te7 (x = 0, 0.05, and 0.1) was conducted, and the results are shown in Fig. 1. Here we did not present the refined XRD data of the compound at x = 0.2 due to abnormal SOFs (site of occupation factors) and big S (goodness of fit indicator) values, likely caused by the precipitation of the visible impurity, Sb (see the XRD patterns of the powders in Fig. 2(a)). Although the precipitation of impurities does not affect the overall compositions, it is noted that the nominal compounds Cu2−xSbxGa4Te7 (x = 0, 0.05 and 0.1) actually crystallize in a crystal structure of CuGaTe2 (PDF, 79-2331(122), s.g.: I
2d), and the real compositions from refinement are Cu0.714GaTe2, Cu0.696Sb0.018GaTe2 and Cu0.68Sb0.034GaTe2 respectively. The deviation of the refined compositions from the nominal ones was highly likely, and can be assumed to be caused by the precipitation of impurity phases when some element contents exceeded their solubilities at certain temperatures. Because of the low analyzing accuracy of XRD analysis, some tiny secondary phases are hard to identify.
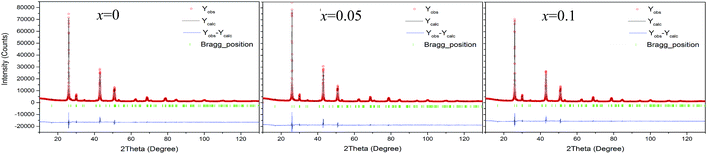 |
| Fig. 1 Rietveld refinements using X-ray diffraction data of the three compounds Cu2−xSbxGa4Te7 (x = 0, 0.05, and 0.1). | |
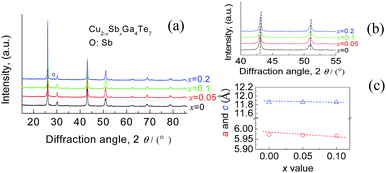 |
| Fig. 2 (a) XRD patterns of the powders Cu2−xSbxGa4Te7 (x = 0–0.1); (b) close-up view of XRD patterns between 40° and 55°; (c) refined lattice constants a and c as a function of the x value, with an analysis error of <0.4%. | |
Shown in Fig. 2(b) is a close-up view of the XRD patterns between 40° and 55°, where the peak positions tend to shift toward large angles, indicating the shrinkage of the crystal lattice. The lattice constants a (5.9662–5.9724) and c (11.8570–11.8587) against the Sb content (x value), taken from the refined results shown in Table 1, are presented in Fig. 2(c). The structural variables in Table 1, such as RB (Bragg factor), Rp (profile factor), Rwp (weighted profile factor), and S, are in the ranges of 7.02–7.89%, 5.22–5.24%, 6.77–7.28%, and 2.73–2.91 respectively. Although the S values seem a little large, they are comparable to those from the refinements on Cu18Ga25Te50 (ref. 21) and Cu3In5Te9.30 Besides, the Wyckoff positions, atomic coordinates and SOFs are presented in Table 2. The SOF values indicate that the element Sb totally resides in the Cu 4b site with SOFs of 0.018 (x = 0.05) and 0.034 (x = 0.1) respectively, while Ga and Te atoms reside in 4a and 8d sites (SOFs = 1). Fig. 3 represents the lattice structure distortion parameter η, where η = c/2a, against the x value for the ternary chalcopyrite compounds. The η value tends to increase and approaches 1.0 as the x value increases, indicating that the crystal structure distortion gets weakened as more Sb is added.
Table 1 Refined structure parameters of Cu2−xSbxGa4Te7 (x = 0, 0.05, and 0.1)
|
x = 0 |
x = 0.05 |
x = 0.1 |
Chemical formula |
(Cu0.714GaTe2) |
(Cu0.696Sb0.018GaTe2) |
(Cu0.680Sb0.034GaTe2) |
Space group |
I 2d (no. 122) |
I 2d (no. 122) |
I 2d (no. 122) |
Z |
4 |
4 |
4 |
a (Å) |
5.9724 (3) |
5.9697 (3) |
5.9662 (3) |
b (Å) |
5.9724 (3) |
5.9697 (3) |
5.9662 (3) |
c (Å) |
11.8570 (1) |
11.8587 (9) |
11.8580 (1) |
V (Å3) |
423.00 (5) |
422.61 (4) |
422.09 (5) |
RB (%) |
7.02 |
7.56 |
7.89 |
Rp (%) |
5.22 |
5.64 |
5.40 |
Rwp (%) |
6.77 |
7.28 |
6.89 |
S |
2.91 |
2.73 |
2.83 |
Table 2 Wyckoff positions, atomic coordinates, and occupancies of Cu2−xSbxGa4Te7
Compositions |
Atom |
Site |
x |
y |
z |
Biso (Å2) |
Occupancy |
x = 0 |
Cu |
4b |
0 |
0 |
0.5 |
0.5(2) |
0.714(1) |
Ga |
4a |
0 |
0 |
0 |
0.9(2) |
1 |
Te |
8d |
0.265(6) |
0.25 |
0.125 |
1.22(2) |
1 |
x = 0.05 |
Cu |
4b |
0 |
0 |
0.5 |
0.9(3) |
0.696(1) |
Sb |
4b |
0 |
0 |
0.5 |
0.9(3) |
0.018(1) |
Ga |
4a |
0 |
0 |
0 |
0.9(2) |
1 |
Te |
8d |
0.2643(7) |
0.25 |
0.125 |
1.29(3) |
1 |
x = 0.1 |
Cu |
4b |
0 |
0 |
0.5 |
1.3(2) |
0.68(1) |
Sb |
4b |
0 |
0 |
0.5 |
1.3(2) |
0.034(1) |
Ga |
4a |
0 |
0 |
0 |
0.9(1) |
1 |
Te |
8d |
0.2645(8) |
0.25 |
0.125 |
1.10(2) |
1 |
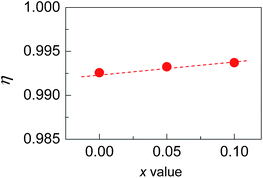 |
| Fig. 3 The lattice distortion parameter η in Cu2−xSbxGa4Te7 (x = 0–0.1) with a chalcopyrite structure as a function of Sb content (x value). | |
3.2 Transport properties
The measured Hall coefficients (RH) are positive, indicating that the materials exhibit p-type semiconducting behavior. The calculated Hall carrier concentration (nH) and mobility (μ) at RT are shown in Fig. 4. Upon Sb incorporation, the nH value grows from 1.02 × 1018 cm−3 (x = 0) to 3.89 × 1019 cm−3 (x = 0.05) as Sb content increases, and then it reduces to 3.32 × 1019 cm−3(x = 0.2). The μ value reduces drastically from 20.3 cm2 V−1 s−1 (x = 0) to 3.9 cm2 V−1 s−1 (x = 0.05) followed by an increasing tendency. At x = 0.2, the μ value is 9.9 cm2 V−1 s−1. These results imply that the transport properties (nH and μ) of carrier are very sensitive to Sb incorporation in Cu2Ga4Te7. However, after incorporation of a small amount of Sb (x = 0.05) in the Cu site, the carrier transport becomes relatively inactive, and only small changes in the nH (μ) value were observed as the Sb content increases. The reason for this might be that the SbCu defect provides two extra electrons which neutralize the p-type holes. On the other hand, the slight changes in nH and μ at x ≥ 0.05 imply that alteration of the chemical environment plays a minor role, based on the estimation made using the valence count rule31,32 (the results are not shown here). In this regard, the origin of the enhancement in nH might be due to the unpinning of the Fermi level followed by its movement into the inner side of the valence band as Sb occupies the Cu site, as is observed in Sb-substituted Cu18Ga25Te50.21
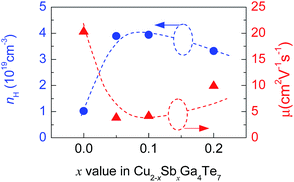 |
| Fig. 4 The Hall carrier concentration (nH) and mobility (μ) at RT of Cu2−xSbxGa4Te7 (x = 0–0.2) compounds as a function of Sb content (x value). | |
3.3 TE performance
The Seebeck coefficients (α) of the Cu2−xSbxGa4Te7 (x = 0–0.2) compounds as a function of temperature are presented in Fig. 5(a). The α values, which are positive, increase as the measured temperature increases, until the peak temperature (∼600 K) is reached. After that, they start to decrease with increasing temperature. Above ∼700 K, the α values at x ≥ 0.05 are much higher than those of the Sb-free sample (x = 0). This might be the result of the dominant increase of effective mass. In order to substantiate this assumption, the dependence of the Seebeck coefficients on the Hall carrier concentration is depicted in Fig. 5(b), assuming that the Pisarenko relation26,33 with the SPB model is valid in the Cu–Ga–Te systems.34–36 This dependence indicates that the α values of the Sb-incorporated samples (circled by dotted line) are much higher than those predicted by the Pisarenko relation at the corresponding carrier concentrations. The solid line depicted in Fig. 5(b) corresponds to the relationship between α and nH for the Sb-free Cu2Ga4Te7 at RT with an effective mass of m* = 0.04me. It is therefore determined that the effective carrier mass increases upon Sb incorporation (see the further discussion below). Besides, as Sb content (x value) increases, the electrical conductivity (σ) has a slight decrease over the whole temperature range (see Fig. 5(c)), and at ∼800 K the σ value decreases from 1.73 × 104 Ω−1 m−1 (x = 0) to 1.68 Ω−1 m−1 (x = 0.05) and 1.34 × 104 Ω−1 m−1 (x = 0.2). The power factors (PF), PF = α2σ, are presented in Fig. 5(d). It was observed that the highest PF value for the Sb-free sample is 4.67 μW cm−1 K−2 at ∼675 K, while that at x = 0.05 is 5.39 μW cm−1 K−2 at ∼800 K, increasing by 16%. Owing to the degradation in electrical conductivity at high temperatures as Sb content increases, it is believable that the enhancement in power factor above ∼700 K is mainly attributed to the increased α values.
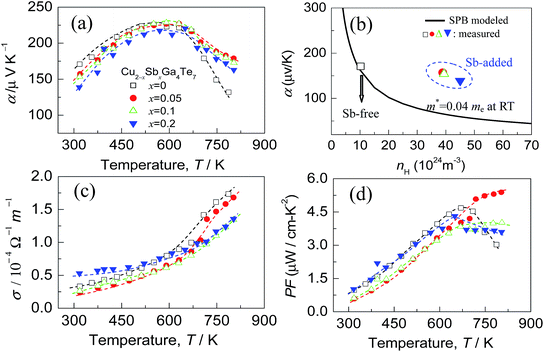 |
| Fig. 5 (a) Seebeck coefficients (α) of the compounds Cu2−xSbxGa4Te7 (x = 0.05, 0.1 and 0.2) as a function of temperature, and that for x = 0 is presented for comparison; (b) the experimentally determined Seebeck coefficients (α) at the corresponding Hall carrier concentrations, labeled by . The solid line represents the Pisarenko relation at RT; (c) electrical conductivities (σ) as a function of temperature for different materials (x values); (d) power factor PF, PF = α2σ, for different materials (x values). | |
Shown in Fig. 6(a) are the lattice thermal conductivities (κL) against temperature for Cu2−xSbxGa4Te7 (x = 0–0.2). The κL values reduce with temperature increasing, roughly obeying the T−1 relation. The κL value at x = 0 is higher than those at x = 0.05 and 0.1 over the whole temperature range, as shown in Fig. 6(a) as an inset. The total thermal conductivities (κ) at x = 0 remain high compared with those of the Sb-incorporated samples (Fig. 6(b)), partly due to high electronic contributions (κe). In addition, the m*/me value increases with increasing x value until x = 0.05, and then it starts to decrease, as shown in Fig. 6(c). However, the quality factor B (B = μH(m*/me)3/2T5/2/κL)33 exhibits an opposite trend to the effective mass. The B value decreases with increasing Sb content until x = ∼0.07, and then increases rapidly. Combined with the three physical parameters (α, σ, and κ) measured, we attained the TE figure of merit (ZT), as shown in Fig. 6(d). At x = 0.05 the highest ZT value is 0.58 at ∼803 K, which is about 0.21 higher than that of the pristine Cu2Ga4Te7 (ZT = 0.37).
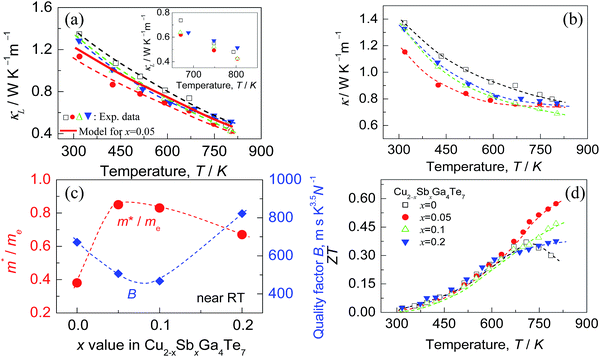 |
| Fig. 6 (a) Lattice thermal conductivities (κL) as a function of temperature for different materials (x values). The solid red line represents the fitted results for the sample at x = 0.05 using the Callaway and Klemens model. The inset is a close-up view of the κL values at high temperatures; (b) total thermal conductivities (κ) as a function of temperature for different materials (x values); (c) the m*/me and quality factor B values as a function of Sb content (x value); (d) TE figure of merit (ZT) as a function of temperature for different materials (x values). | |
In brief, the improvement in TE performance is attributed to two main aspects: increased Seebeck coefficient, and reduced lattice thermal conductivity.
In general, at a temperature far above the Debye temperature, all phonon modes are activated. In the present nominal compounds Cu2−xSbxGa4Te7 with chalcopyrite structure, the lattice thermal conductivity is governed by complex scattering mechanisms, such as, the lattice disorder scattering, Umklapp scattering, phonon–electron scattering, and the extra scattering caused by the crystal structure distortion. However, the scattering caused by the crystal structure distortion should be decreased, because the distortion parameter η has an increasing tendency (approaching 1.0) (see Fig. 3), based on the previous investigations.37–39 While the extra scattering resulted from the created SbCu defect should be larger, since the regular arrangement of the one-seventh cation vacancies in the Cu2Ga4Te7 system28 suffers disturbance when Sb resides in the Cu site (Table 2), attributed to the differences in atomic size and electronegativity between Sb (1.53 Å, 2.05) and Cu (1.57 Å, 1.9).40 That is why we have observed a general reduction in κL as the Sb content increases. The high κL values at x = 0.2 at high temperatures might be due to the donor SbCu defect along with the visible Sb impurity neutralizing the inherent p-type cation vacancy, thus reducing the vacancy scattering centers of phonons.27
In order to substantiate the general reduction in κL as Sb content increases, we estimated the κL values by means of the Callaway and Klemens model41–43 to outline the contributions from the Umklapp and point defect scatterings.34,44 When estimating κL using this model, the ratio of the modeled lattice thermal conductivity of the crystal with Sb substitution for Cu, κLm, to the lattice thermal conductivity of the pure crystal, κLp, is given below,
|
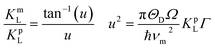 | (1) |
where
u and
Γ are the disorder scaling parameter and the disorder scattering parameter respectively. Here we use the factor
Γ below to predict the
κL values for the Cu–Ga–Te based chalcogenides,
34 |
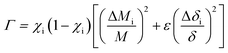 | (2) |
where
χi, Δ
Mi/
M and Δ
δi/
δ are the molar fraction of Sb, relative change of atomic mass due to the replacement of Cu by Sb, and the local change in lattice parameter.
ε = 2(
W + 6.4
γ)
2 is determined by using the Grüneisen parameter
γ = 1.46 and
W = 3.
34 The other related parameters are presented in
Table 3.
Table 3 Parameters used for estimating the lattice thermal conductivity (κL) at x = 0.05 using the Callaway and Klemens model
Symbol |
Representation |
ΘD |
Debye temperature, 222 K (ref. 28) |
νm |
Average sound velocity, 2227 m s−1 (ref. 25 and 45) |
ℏ |
Planck constant |
Ω |
Volume per atom |
The fitting results for the sample at x = 0.05 using the above model are shown in Fig. 6(a) as a red solid curve. Roughly, the estimated κL values follow the same decreasing trend as the experimental data over the whole temperature range, which confirms the importance of the Umklapp and point defect scattering mechanisms. However, it is worth noting that the estimated κL values are a little higher than the measured ones, suggesting that the Umklapp and point defect scattering are not enough to account for the reduction in κL, although the fitting may introduce some error using 222 K as the Debye temperature.28 It is therefore believed that there exists another phonon scattering mechanism, that is, the phonon–electron scattering, due to an enhanced carrier concentration upon Sb incorporation. This scattering plays a major role in further reducing κL. Here it should be pointed out that we did not estimate the κL values of the samples at x = 0.1 and 0.2, because the Debye temperature (222 K) of these materials might change significantly. Therefore, it is not suitable for further estimations to be made.
4. Conclusions
The Cu2−xGa4SbxTe7 ternary compounds with Sb substituted for Cu were prepared and their TE properties examined. Rietveld refinement reveals that these compounds (x = 0, 0.05 and 0.1) actually crystallize in a crystal structure of CuGaTe2, and the real compositions are Cu0.714GaTe2, Cu0.696Sb0.018GaTe2 and Cu0.68Sb0.034GaTe2 respectively. Besides, Sb is incorporated into the Cu site, which is responsible for the enhancement in Hall carrier concentration (nH) and the decrease in mobility (μ). In addition, the Seebeck coefficient increases above ∼700 K, due to an increase of the effective carrier mass. The reduction in lattice thermal conductivity (κL) is closely related to the increase in point defect and phonon–electron scattering as the Sb content increases. As a consequence, the highest ZT value of 0.58 is reached at ∼803 K for the Cu1.95Ga4Sb0.05Te7 sample, which is about 0.21 higher than that of the pristine Cu2Ga4Te7 (ZT = 0.37).
Conflicts of interest
There are no conflicts to declare.
Acknowledgements
This work was supported by the National Natural Science Foundation of China (51671109, 51171084). We are also grateful for some useful suggestions from Prof. Qinsen Meng of the Taiyuan University of Technology.
Notes and references
- K. F. Hsu, S. Loo, F. Guo, W. Chen, J. S. Dyck, C. Uher, T. Hogan, E. K. Polychroniadis and M. G. Kanatzidis, Science, 2004, 303, 818 CrossRef PubMed
. - B. Poudel, Q. Hao, Y. Ma, Y. Lan, A. Minnich, B. Yu, X. Yan, D. Wang, A. Muto, D. Vashaee, X. Chen, J. Liu, M. S. Dresselhaus, G. Chen and Z. Ren, Science, 2008, 320, 634 CrossRef PubMed
. - J. He, S. N. Girard, M. G. Kanatzidis and V. P. Dravid, Adv. Funct. Mater., 2010, 20, 764 CrossRef
. - Y. Pei, X. Shi, A. LaLonde, H. Wang, L. Chen and G. J. Snyder, Nature, 2011, 473, 66 CrossRef PubMed
. - J. P. Heremans, V. Jovovic, E. S. Toberer, A. Saramat, K. Kurosaki, A. Charoenphakdee, S. Yamanaka and G. J. Snyder, Science, 2008, 321, 554 CrossRef PubMed
. - S. Lin, W. Li, Z. Chen, J. Shen, B. Ge and Y. Pei, Nat. Commun., 2016, 7, 10287 CrossRef PubMed
. - H. Liu, X. Shi, F. Xu, L. Zhang, W. Zhang, L. Chen, Q. Li, C. Uher, T. Day and G. J. Snyder, Nat. Mater., 2012, 11, 422 CrossRef PubMed
. - H. Liu, X. Yuan, P. Lu, X. Shi, F. Xu, Y. He, Y. Tang, S. Bai, W. Zhang, L. Chen, Y. Lin, L. Shi, H. Lin, X. Gao, X. Zhang, H. Chi and C. Uher, Adv. Mater., 2013, 25, 6607 CrossRef PubMed
. - W. Zhao, Z. Liu, P. Wei, Q. Zhang, W. Zhu, X. Su, X. Tang, J. Yang, Y. Liu, J. Shi, Y. Chao, S. Lin and Y. Pei, Nat. Nanotechnol., 2017, 12, 55 CrossRef PubMed
. - W. Zhao, Z. Liu, Z. Sun, Q. Zhang, P. Wei, X. Mu, H. Zhou, C. Li, S. Ma, D. He, P. Ji, W. Zhu, X. Nie, X. Su, X. Tang, B. Shen, X. Dong, J. Yang, Y. Liu and J. Shi, Nature, 2017, 549, 247 CrossRef PubMed
. - Y. Pei, Z. M. Gibbs, A. Gloskovskii, B. Balke, W. G. Zeier and G. J. Snyder, Adv. Energy Mater., 2014, 4, 1400486 CrossRef
. - T. Plirdpring, K. Kurosaki, A. Kosuga, T. Day, S. Firdosy, V. Ravi, G. J. Snyder, A. Harnwunggmoung, T. Sugahara, Y. Ohishi, H. MuTa and S. Yamanaka, Adv. Mater., 2012, 24, 3622 CrossRef PubMed
. - J. Zhang, R. Liu, N. Cheng, Y. Zhang, J. Yang, C. Uher, X. Shi, L. Chen and W. Zhang, Adv. Mater., 2014, 26, 3848 CrossRef PubMed
. - J. Shen, X. Zhang, Z. Chen, S. Lin, J. Li, W. Li, S. Li, Y. Chen and Y. Pei, J. Mater. Chem. A, 2017, 5, 5314 RSC
. - S. B. Zhang, S. Wei and A. Zunger, Phys. Rev. B: Condens. Matter Mater. Phys., 1998, 57, 9642 CrossRef
. - J. E. Jaffe and A. Zunger, Phys. Rev. B: Condens. Matter Mater. Phys., 1983, 28, 5822 CrossRef
. - H. Neumann, W. Kissinger and R. D. Tomlinson, Mater. Lett., 1986, 4, 57 CrossRef
. - Y. Qin, P. Qiu, R. Liu, Y. Li, F. Hao, T. Zhang, D. Ren, X. Shi and L. Chen, J. Mater. Chem. A, 2016, 4, 1277 RSC
. - F. Ahmed, N. Tsujii and T. Mori, J. Mater. Chem. A, 2017, 5, 7545 RSC
. - J. Zhang, X. Y. Qin, D. Li, H. X. Xin, C. J. Song, L. L. Li, Z. M. Wang, G. L. Guo and L. Wang, J. Alloys Compd., 2014, 586, 285 CrossRef
. - J. Zhu, Y. Luo, G. Cai, X. Liu, Z. Du, F. Tang and J. Cui, J. Mater. Chem. A, 2017, 5, 24199 RSC
. - J. Cui, Y. Li, Z. Du, Q. Meng and H. Zhou, J. Mater. Chem. A, 2013, 1, 677 RSC
. - T. Plirdpring, K. Kurosaki, A. Kosuga, M. Ishimaru, A. Harnwunggmoung, T. Sugahara, Y. Ohishi, H. Muta and S. Yamanaka, Mater. Trans., 2012, 53, 1212 CrossRef
. - A. Congiu, L. Garbato and P. Manca, Mater. Res. Bull., 1973, 8, 293 CrossRef
. - Z. Ye, J. Cho, M. M. Tessema, J. R. Salvador, R. A. Waldo, H. Wang and W. Cai, J. Solid State Chem., 2013, 201, 262 CrossRef
. - G. J. Snyder and E. S. Toberer, Nat. Mater., 2008, 7, 105 CrossRef PubMed
. - S. Chen, W. Ren, Q. Meng, X. Liu, J. Yang and J. Cui, Phys. Status Solidi A, 2014, 211, 618 CrossRef
. - T. Plirdpring, K. Kurosaki, A. Kosuga, M. Ishimaru, A. Harnwunggmoung, T. Sugahara, Y. Ohishi, H. Muta and S. Yamanaka, Appl. Phys. Lett., 2011, 98, 172104 CrossRef
. - M. Gao, J. Zhang and D. M. Rowe, Thermoelectric conversion and its applications (in Chinese), The Publishing House of Ordnance Industry, 1996, p. 53 Search PubMed
. - E. Guedez, L. Mogollón, G. Marcano, S. M. Wasim, G. Sánchez Pérez and C. Rincón, Mater. Lett., 2017, 186, 155 CrossRef
. - E. S. Toberer, A. F. May and G. J. Snyder, Chem. Mater., 2010, 22, 624 CrossRef
. - A. F. May, J.-P. Fleurial and G. J. Snyder, Chem. Mater., 2010, 22, 2995 CrossRef
. - X. Shi, L. Chen and C. Uher, Int. Mater. Rev., 2016, 61, 379 CrossRef
. - J. Shen, Z. Chen, S. Lin, L. Zheng, W. Li and Y. Pei, J. Mater. Chem. A, 2016, 4, 15464 RSC
. - V. K. Gudelli, V. Kanchana, G. Vaitheeswaran, A. Svan and N. E. Christensen, J. Appl. Phys., 2013, 114, 223707 CrossRef
. - D. Zou, S. Xie, Y. Liu, J. Lin and J. Li, J. Alloys Compd., 2013, 570, 150 CrossRef
. - W. Wu, Y. Li, Z. Du, Q. Meng, Z. Sun, W. Ren and J. Cui, Appl. Phys. Lett., 2013, 103, 011905 CrossRef
. - J. Cui, Z. Sun, Z. Du and Y. Chao, J. Mater. Chem. C, 2016, 4, 8014 RSC
. - Y. Lu, S. Chen, W. Wu, Z. Du, Y. Chao and J. Cui, Sci. Rep., 2017, 7, 40224 CrossRef PubMed
. - M. Li. Concise Handbook of Chemical Data (In Chinese), Chemical Industry Press, Beijing, 2002, p. 9 Search PubMed
. - P. G. Klemens, Thermal resistance due to point defects at high temperatures, Phys. Rev., 1960, 119, 507 CrossRef
. - J. Callaway and H. C. von Baeyer, Phys. Rev., 1960, 120, 1149 CrossRef
. - C. P. Heinrich, C. P. Heinrich, T. W. Day, W. G. Zeier, G. J. Snyder and W. Tremel, J. Am. Chem. Soc., 2014, 136, 442 CrossRef PubMed
. - Z. Xiaa, G. Wang, X. Zhou and W. Wen, Ceram. Interfaces, 2017, 43, 16276 CrossRef
. - A. Rivero, M. Quintero, C. Power, J. Gonzalez, R. Tovar and J. Ruiz, J. Electron. Mater., 1997, 26, 1428 CrossRef
.
Footnote |
† Electronic supplementary information (ESI) available. See DOI: 10.1039/c8ra03704c |
|
This journal is © The Royal Society of Chemistry 2018 |