DOI:
10.1039/C8RA03273D
(Paper)
RSC Adv., 2018,
8, 22046-22052
Mixed metal CoII1−xZnIIx–organic frameworks based on chains with mixed carboxylate and azide bridges: magnetic coupling and slow relaxation†
Received
17th April 2018
, Accepted 3rd June 2018
First published on 14th June 2018
Abstract
A series of isomorphous three-dimensional metal–organic frameworks [CoII1−xZnIIx(L)(N3)]·H2O (x = 0.26, 0.56 and 0.85) based on bimetallic CoII1−xZnIIx (x = 0.26, 0.56 and 0.85) chains with random metal sites have been synthesized and magnetically characterized. The CoII1−xZnIIx series, which intrinsically feature random anisotropic/diamagnetic sites, shows complex magnetic interactions. By gradually introducing the diamagnetic ZnII ions into the pure anisotropic CoII single-chain magnets system, the ferromagnetic interactions between CoII ions are gradually diluted. Moreover, the slow magnetic relaxation behaviour of the mixed metal CoII1−xZnIIx systems also changes. In this bimetallic series CoII1−xZnIIx, the Co-rich materials exhibit slow relaxation processes that may arise from SCM mechanism, while the ZnII-rich materials show significantly low slow magnetic relaxation. A general trend is that the activation energy and the blocking temperature decrease with the increase in diamagnetic ZnII content, emphasizing the importance of anisotropy for slow relaxation of magnetization.
Introduction
Recently, single-chain magnets (SCMs) have attracted significant attention due to their unique physical behaviours and potential applications in information storage and quantum computing between chemistry and physics.1 Slow magnetic relaxation is characteristic for SCMs intrinsic to individual chains, which can lead to blocking of magnetization below a certain temperature. Raising the blocking temperature (TB) is a great challenge in the study of SCMs. The general strategy is to increase the barrier of magnetic relaxation by increasing magnetic anisotropy and/or intrachain magnetic interaction. Therefore, it is of great importance for the choice of spin carriers with large magnetic anisotropy and bridging ligands between them. Although some homospin CoII,2 MnII,3 FeII4 and LnIII5 SCMs have been reported, heterospin SCMs such as mixed-valence,6 metal-radical,7 bimetallic (3d–3d,8 3d–4d,9 3d–5d,10 or d–f11) and even tri-spin compounds12 are more common because the magnetic interactions and anisotropy are easier to tune in heterospin systems.
In this context, we performed a series of systematic studies and successfully obtained several homospin SCMs. In these compounds, CoII or FeII ions are bridged by simultaneous azide-carboxylate,13 azide-tetrazolate,14 or azide-carboxylate-tetrazolate bridges,15 and the bridges transmit ferromagnetic coupling (FO), where the formation of the particular bridging systems benefited from the use of zwitterionic pyridinium carboxylate ligands. It is worth mentioning that a family of isomorphous three-dimensional (3D) MnII, FeII, CoII and NiII metal–organic frameworks (MOFs) has been obtained from different transition metal ions, azide ions and a simple zwitterionic ligand (L) (Scheme 1). The MnII MOF shows typical 1D antiferromagnetism (AF), while the anisotropic FeII, CoII and NiII compounds behave as FO SCMs in 3D MOFs. Inspired by the isomorphism of these MOFs and with the aim of tuning the SCM behaviours, we focused on the magnetic behaviour of isomorphous mixed-metal systems where two different anisotropic metal ions are randomly distributed over structurally equivalent sites in variable ratios. This metal-mixing strategy has been widely used in materials science to tune the properties of materials, and the study of random mix-metal magnetic materials such as metallic alloys, oxides, halides, and some rare molecular systems has revealed unusual magnetic phenomena.16 To the best of our knowledge, random bimetallic SCM systems remain largely unexplored.17 Recently, we have reported four mixed-metal systems, namely, Fe1−xMnx,18 Co1−xNix,19 Fe1−xCox and Fe1−xNix.13a The Fe1−xMnx system shows a gradual AF-to-FO evolution in overall behaviours as the Fe(II) content increases. Interestingly, we found that a Co-rich Co1−xNix system can exhibit higher TB than both parent materials, whereas the bimetallic Fe1−xCox system demonstrates a significantly lower TB than both parent FeII and CoII materials. Furthermore, the Fe1−xNix materials show direct composition dependence, where the TB decreases monotonically as FeII is replaced by the less anisotropic NiII. Herein, as a continuation of the research on mixed metal systems, we examine the Co1−xZnx series (x = 0.26, 0.56 and 0.85), which intrinsically features random anisotropic (CoII) and diamagnetic sites (ZnII), which is manifested through complex magnetic interactions. By the gradual introduction of diamagnetic ZnII ions into the pure anisotropic CoII single-chain magnets system, the FO interaction between CoII ions is gradually diluted. Moreover, the slow magnetic relaxation behaviour is also changed. In this bimetallic series CoII1−xZnIIx, the Co-rich materials show slow relaxation behaviour that may arise in typical SCMs, and the slow magnetic relaxation is significantly reduced with the increase in diamagnetic ZnII content. A general trend is that the activation energy and TB decrease with the increase in ZnII content.
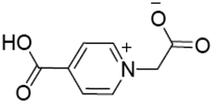 |
| Scheme 1 1-Carboxymethylpyridinium-4-carboxylate (HL). | |
Experimental section
Materials and physical measurements
The reagents were obtained from commercial sources and used without further purification. 1-Carboxymethylpyridinium-4-carboxylate (HL) was prepared according to a method reported in the literature. Elemental analyses (C, H, and N) were performed on a Perkin-Elmer 2400 CHN elemental analyzer. FT-IR spectra were recorded in the range 500–4000 cm−1 on a Nicolet NEXUS 670 spectrophotometer using KBr pellets. The X-ray diffraction (XRD) patterns were collected on a Rigaku Ultima IV X-ray diffractometer using Mo-Kα radiation (λ = 0.71073 Å) at 35 kV and 25 mA. Inductively coupled plasma (ICP) analysis was carried out on an IRIS Intrepid II XSP spectrometer. Magnetic measurements were performed on a Quantum Design MPMS XL5 SQUID magnetometer. The experimental susceptibilities were corrected for the diamagnetism of the constituent atoms (Pascal's tables).
Caution! Although not encountered in our experiments, azido compounds of metal ions are potentially explosive. Only a small amount of the materials should be prepared, and it should be handled with care.
[Zn(L)(N3)]·H2O (1)
A mixture of Zn(ClO4)2·6H2O (0.2 mmol, 0.075 g), HL (0.1 mmol, 0.018 g) and NaN3 (0.40 mmol, 0.026 g) in water/ethanol (5/5 mL) was stirred for 10 min at room temperature. Slow evaporation of the solution at room temperature yielded light orange block crystals after one day. Yield: 65% based on HL. Elem anal. calcd (%) for C8H8ZnN4O5: C, 32.40; H, 2.38; N, 18.89. Found: C, 32.13; H, 2.72; N, 19.36%. IR bands (KBr, cm−1): ν(N3) 2082 vs., ν(COO) 1627s, 1563 m and 1384s.
[CoII1−xZnIIx(L)(N3)]·H2O (x = 0.26, 0.56 and 0.85)
ZnNO3·6H2O and CoNO3·6H2O in ratios 13
:
37, 14
:
11 and 17
:
3 (the total quantity of the metal salts is 0.4 mmol), were dissolved in methanol (4 mL). An aqueous solution (2 mL) of HL (0.072 g, 0.40 mmol) was added, and the clear solution was heated to reflux for 10 minutes. The addition of sodium azide (0.10 g, 1.6 mmol) to the solution under stirring led to polycrystalline precipitates. After refluxing for 3 h, the solid was collected by filtration, washed with water and methanol, and dried in air. Yields: 50–85% based on metal salts. The metal ratios in the products were determined by the inductively coupled plasma (ICP) atomic emission spectrometry. The C/H/N elemental analysis results of these compounds are identical within experimental errors. The compounds exhibit almost identical IR spectra and similar PXRD patterns.
Crystal data collection and refinement
Diffraction data for 1 was collected at 293 K on a Bruker Apex II CCD area detector equipped with graphite-monochromated Mo Kα radiation (λ = 0.71073 Å). Empirical absorption corrections were applied using the SADABS program.20 The structures were solved by the direct method and refined by the full-matrix least-squares method on F2 using the SHELXL program, with anisotropic displacement parameters for all non-hydrogen atoms.21 All of the hydrogen atoms attached to carbon atoms were placed in calculated positions and refined using the riding model. The water hydrogen atoms attached to O3 were located from the difference Fourier map and refined with restrained O–H and H⋯H distances (3 restrains using the DFIX instruction). A summary of the crystallographic data, data collection, and refinement parameters for compound 1 is provided in Table S1,† and the selected bond lengths and angles for compound 1 are listed in Table S2.† The ccdc number for compound 1 is 1832808.
Results and discussion
Synthesis
A series of mixed-metal MOFs, [Co1−xZnx(L)(N3)], was synthesized as polycrystalline solids through the reactions of the corresponding metal salts mixed in various ratios with the zwitterionic ligand HL and NaN3 in aqueous methanol. The compounds show almost identical IR spectra, with strong absorptions at about 2068, 1625, and 1383 cm−1 that are characteristic of νas(N3), νas(COO), and νs(COO), respectively. The powder X-ray diffraction (PXRD) profiles are also very similar and consistent with those simulated from the single-crystal data of the ZnII-only and CoII-only compounds (Fig. 1), indicative of the isomorphism and phase purity across these series.
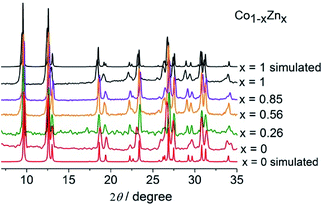 |
| Fig. 1 PXRD patterns of the CoII1−x ZnIIx compounds. | |
Crystal structure
The single crystal X-ray analyses indicated that the structure of 1 was isomorphous with our previously reported compounds [Co(L)(N3)]·H2O19 and [Mn(L)(N3)]·H2O,18 which was a chain-based network. As shown in Fig. 2a, the unique Zn(II) assumes the centrosymmetric trans-octahedral [N2O4] geometry defined by two azide nitrogen atoms (N1 and N1A) and four carboxylate oxygen atoms (O1, O1C, O2B and O2D). The Zn–O and Zn–N distances fall in the range of 2.096(2)–2.152(3) Å (Table S1, ESI†). Adjacent Zn ions with Zn⋯Zn = 3.641(1) Å are bridged by an azide in the end-on mode and two carboxylate bridges in the syn–syn mode to yield a formally anionic chain ([Zn(μ-N3)(μ-OCO)2]nn−) along the crystallographic b direction. The adjacent octahedrons along the chain share the bridging nitrogen atom with Zn–N–Zn = 120.5(5)° and are tilted towards each other with a dihedral angle of 54.3° between the [MO4] equatorial planes. All the bond lengths and angles are comparable with the values of isomorphous Mn(II) and Co(II) compounds.18,19
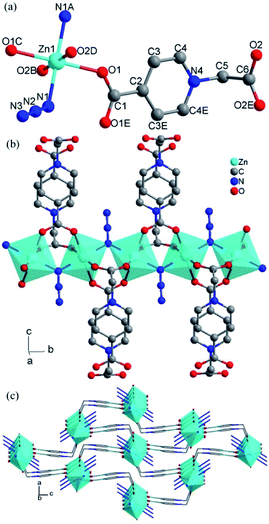 |
| Fig. 2 (a) Local coordination environment of the Zn2+ center in 1 (hydrogen atoms are omitted for clarify). Symmetry code: (A) 1 − x, y − 0.5, 1 − z; (B) x − 0.5, y, 1.5 − z; (C) 1 − x, −y, 1 − z; (D) 1.5 − x, −y, z − 0.5; (E) x, 0.5 − y, z. (b) Chain with mixed carboxylate and azide bridges. (c) 3D network. | |
Each [Zn(μ-N3)(μ-OCO)2]nn− chain is linked with four others by the cationic N-methylenepyridinium tethers of the L ligands to produce a neutral 3D MOF. The interchain space is divided by the L ligands bent into small channels, in which free water molecules are enclosed.
Magnetic properties of the CoII1−xZnIIx series
The magnetic susceptibilities of the Co1−xIIZnxII mixed-metal compounds were measured under 1000 Oe in the temperature range 2–300 K and shown as χT–T plots in Fig. 3 (top), in which, for convenience of comparison, the data for the CoII (x = 0) parent compound is also included.
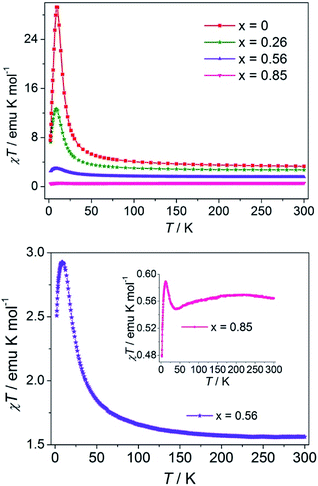 |
| Fig. 3 Temperature dependence of χT of the CoII1−x ZnIIx series at 1000 Oe (top). The enlarged view of Co1−xZnx (x = 0.56 and 0.85) (bottom). | |
The χT values (0.56–2.71 emu mol−1 K) of the mixed-materials at 300 K are below the value of CoII compound (3.30 emu mol−1 K) and decrease with Zn(II) content. For x = 0.26 and 0.56, the trend of χT varying with T is similar to that for 1, showing a maximum at low temperatures. The maximum χT value and the corresponding temperature decrease with Zn(II) content (Fig. 3 and Table 1). The increase in χT with the decrease in temperature demonstrates FO interactions between Co(II) ions transmitted by the (OCO)2(EO-N3) triple bridges. The decrease in χT at low temperature is mainly due to the saturation effect of the relatively high applied field, a common phenomenon for ferromagnetic systems. The χ−1 versus T plots above 20 K for compounds with x = 0.26 and 30 K for compounds with x = 0.56 follow the Curie–Weiss law. As shown in Table 1, both the Curie constant and the Weiss temperature (Θ) decrease with the increase in Zn(II) content. The rapid decrease in Θ does not suggest weakening of the ferromagnetic coupling between Co(II) ions because the bridging moieties are the same in these compounds. Instead, the decrease in Θ is because the ferromagnetic correlation along the chain is interrupted by the diamagnetic Zn(II) ions. The trend indicates that Co(II) and Zn(II) are mixed within individual chains. If the compound was a mixture of Co(II) chains and Zn(II) chains (i.e., Co(II) and Zn(II) are in separate chains), Θ would not vary with Zn(II) content (the Curie constant always decreases).
Table 1 Selected magnetic data for the CoII1−x ZnIIx compounds
χT value at room temperature. Temperature at which χT shows a maximum at 1 kOe. Temperature at which χ′′ at 1 kHz shows a peak. Magnetization value at 50 kOe. |
x |
0.85 |
0.56 |
0.26 |
0 |
χT/emu mol−1 Ka |
0.56 |
2.03 |
2.71 |
3.30 |
Tmax(χT)/Kb |
12.1 |
6.4 |
8.9 |
9.5 |
C/emu mol−1 K |
0.6 |
1.51 |
2.6 |
3.03 |
Θ/K |
−0.8 |
8.9 |
13.8 |
27.2 |
Δτ/K |
— |
46.1 |
50.5 |
49.7 |
Tp(χ′′)/Kc |
— |
3.2 |
3.3 |
3.9 |
Md |
0.43 |
1.15 |
1.92 |
2.05 |
The χT–T plot of the ZnII-rich compound with x = 0.85 is slightly more complicated. Upon cooling, after a very broad maximum at around 200 K, χT decreases to a broad minimum at 40 K, then increases rapidly to a sharp maximum at 12 K, and finally drops rapidly. The complex temperature dependence is the consequence of the interplay and competition of several effects, including the magnetic interactions between Co(II) ions, the spin-orbital coupling and symmetry-distortion on single Co(II) sites, and also the field effect.22 The decrease in χT from 200 to 40 K is because the single-ion effects overcompensate the ferromagnetic interactions between Co(II) ions, and the situation is reversed at lower temperatures. The final drop should be mainly due to the field-induced saturation effect. The single-ion effects are also present in the compounds with x = 0, 0.26 and 0.56, but obscured by the ferromagnetic Co(II)–Co(II) interactions. With an increase in x, increasing numbers of Co(II) ions are isolated by Zn(II) ions and finally, the ferromagnetic effect gives way to the single-ion effects.
The magnetic behaviors of the x = 0.56 and 0.85 compounds confirm that the metal ions are not uniformly but randomly distributed along the chain. The uniform distribution implies that Co(II) ions would be completely isolated by diamagnetic Zn(II) ions. For x = 0.85, two neighboring Co(II) ions would be separated by five to six Zn(II) ions with a distance of >21 Å. The magnetic isolation would not allow for the ferromagnetic interactions observed in the compounds.
As shown in Fig. 4, the isothermal magnetization data at 2 K indicates that the larger the Co(II) content, the more rapidly the low-field magnetization increases, and the larger the magnetization at 50 kOe. There is no indication of saturation in the high-field region and the magnetization values are in the range of 0.44–2.07 Nβ (Fig. 4, top). No appreciable hysteresis was detected for the mixed-metal compounds (Fig. 4, bottom).
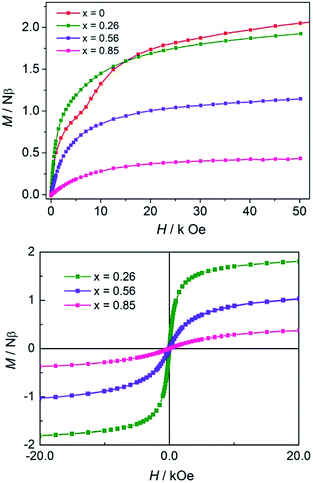 |
| Fig. 4 Virgin magnetization curves of the CoII1−x ZnIIx compounds (x = 0, 0.26, 0.56 and 0.85) at 2 K (top). Hysteresis loops of compounds (x = 0.26, 0.56 and 0.85) at 2 K (bottom). | |
The thermal ac magnetic measurements of the mixed-metal compounds (x = 0.26 and 0.56) at different frequencies are shown in Fig. 5. The two compounds show non-zero out-phase signals below a certain temperature, which shifts to higher temperature as the Co(II) content increases. Both χ′and χ′′ data of the two compounds show frequency dependence. Nevertheless, the specific situation is different: for the compound (x = 0.26), χ′ and χ′′ data both show maxima above 2 K, while for the compound (x = 0.56), the χ′ data shows no maxima when χ′′ data shows maxima above 2 K. This phenomenon indicates that slow relaxation of magnetization occurs and the blocking temperature (TB) increases with the increase in the FO and anisotropic components. The parameter φ = (ΔTp/Tp)/Δ(log
f) (Tp is the peak temperature of χ′′) has been used as a measure of the frequency dependence.23 For compound (x = 0.26), φ = 0.14 and for compound (x = 0.56), φ = 0.13; both these values are in the range for superparamagnets.23,24 The relaxation time τ(T) data obtained from the χ′′(T) data of two compounds follow the Arrhenius law τ(T) = τo
exp(Δτ/T) (Fig. S1, ESI†) with τo = 4.86 = 10−11 s and Δτ = 50.5 K for compound (x = 0.26) and τo = 1.28 = 10−10 s and Δτ = 46.2 K for compound (x = 0.56), suggesting a thermally activated relaxation.18,22 The Δτ and τo values lie in the usual range for superparamagnets including SCMs.1c
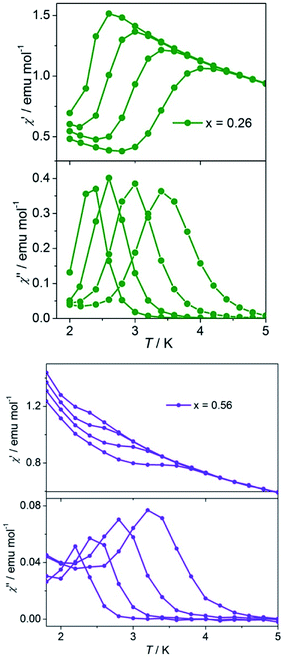 |
| Fig. 5 Ac susceptibilities of the mixed compounds at frequencies 1, 10, 100, and 1000 Hz (from left to right) with Hdc = 0 and Hac = 3.5 Oe for compounds x = 0.26 (top) and x = 0.56 (bottom). | |
Frequency dependent measurements at 2.6 K for compounds x = 0.26 and x = 0.56 were performed. For compound with x = 0.26 (more CoII component), frequency dependent measurements produced a semicircle Cole–Cole diagram (Fig. 6), which was fitted to the generalized Debye model25 with α = 0.27, indicating a distribution of relaxation time and lies in the wide range (0.01–0.7) for reported SCMs. Compound x = 0.56 (less CoII component) shows the dissymmetric shape of the Cole–Cole diagram (Fig. 6), which is consistent with the broad and dissymmetric χ′′–T peaks and indicates the presence of additional relaxation processes and hence, cannot be fitted to the generalized Debye model. One possible origin of the additional relaxation is the spin-glass dynamics, which could be associated with the inherent site randomness.
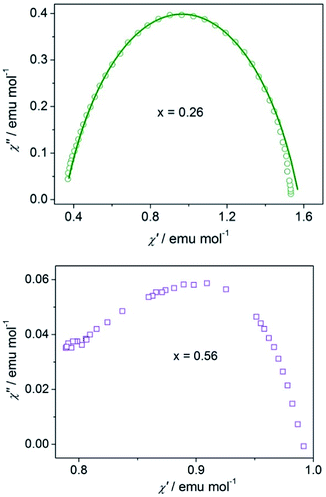 |
| Fig. 6 Cole–Cole diagrams for the compounds with x = 0. 26 (top) and x = 0.56 (bottom). | |
Conclusions
In conclusion, we have studied the magnetic properties of a family of isomorphous bimetallic Co1−xZnx MOFs, in which carboxylate-azide bridged chains with random CoII/ZnII sites are interlinked by N-methylene pyridinium groups. It has been demonstrated that through the gradual introduction of diamagnetic ZnII ions into the anisotropic CoII single-chain magnets system, the FO interaction between CoII ions are gradually diluted, and the slow magnetic relaxation behaviour also changes. For the bimetallic CoII1−xZnIIx system, the Co-rich materials show slow relaxation behaviour, a mechanism related to SCMs, while the slow magnetic relaxations are significantly reduced with the increase in diamagnetic ZnII content.
Conflicts of interest
There are no conflicts to declare.
Acknowledgements
We are thankful for the financial support from NSFC (21761022, 21301087, 21173083) and the Inner Mongolia autonomous region natural science fund project (2017MS0204).
Notes and references
-
(a) K. Liu, X. Zhang, X. Meng, W. Shi, P. Cheng and A. K. Powell, Chem. Soc. Rev., 2016, 45, 2423 RSC;
(b) C. Coulon, V. Pianet, M. Urdampilleta and R. Clerac, Struct. Bonding, 2014, 164, 143 CrossRef;
(c) H. L. Sun, Z. M. Wang and S. Gao, Coord. Chem. Rev., 2010, 254, 1081 CrossRef;
(d) W. J. Jiang, C. Q. Jiao, Y. S. Meng, L. Zhao, Q. Liu and T. Liu, Chem. Sci., 2018, 9, 617 RSC;
(e) R. Li, Y. Xiao, S. H. Wang, X. M. Jiang, Y. Y. Tang, J. G. Xu, Y. Yan, F. K. Zheng and G. C. Guo, J. Mater. Chem. C, 2017, 5, 513 RSC;
(f) X. H. Zhao, L. D. Deng, Y. Zhou, D. Shao, D. Q. Wu, X. Q. Wei and X. Y. Wang, Inorg. Chem., 2017, 56, 8058 CrossRef PubMed.
-
(a) Y. L. Wu, F. S. Guo, G. P. Yang, L. Wang, J. C. Jin, X. Zhou, W. Y. Zhang and Y. Y. Wang, Inorg. Chem., 2016, 55, 6592 CrossRef PubMed;
(b) X. Ma, Z. Zhang, W. Shi, L. Li, J.Zou and P. Cheng, Chem. Commun., 2014, 50, 6340 RSC;
(c) M. H. Zeng, Z. Yin, Y. X. Tan, W. X. Zhang, Y. P. He and M. Kurmoo, J. Am. Chem. Soc., 2014, 136, 4680 CrossRef PubMed;
(d) Z. S. Cai, M. Ren, S. S. Bao, N. Hoshino, T. Akutagawa and L. M. Zheng, Inorg. Chem., 2014, 53, 12546 CrossRef PubMed;
(e) K. Chakarawet, P. C. Bunting and J. R. Long, J. Am. Chem. Soc., 2018, 140, 2058 CrossRef PubMed;
(f) X. Y. Liu, L. Sun, H. L. Zhou, P. P. Cen, X. Y. Jin, G. Xie, S. P. Chen and Q. L. Hu, Inorg. Chem., 2015, 54, 8884 CrossRef PubMed;
(g) G. Brunet, D. A. Safin, J. Jover, E. Ruiz and M. Murugesu, J. Mater. Chem. C, 2017, 5, 835 RSC.
-
(a) W. X. Zhang, T. Shiga, H. Miyasaka and M. Yamashita, J. Am. Chem. Soc., 2012, 134, 6908 CrossRef PubMed;
(b) X. Chen, S. Q. Wu, A. L. Cui and H. Z. Kou, Chem. Commun., 2014, 50, 2120 RSC;
(c) Y. Z. Zhang, H. H. Zhao, E. Funck and K. R. Dunbar, Angew. Chem., Int. Ed., 2015, 54, 5583 CrossRef PubMed;
(d) T. T. Wang, M. Ren, S. S. Bao, B. Liu, L. Pi, Z. S. Cai, Z. H. Zheng, Z. L. Xu and L. M. Zheng, Inorg. Chem., 2014, 53, 3117 CrossRef PubMed;
(e) M. Ding, B. W. Wang, Z. M. Wang, J. L. Zhang, O. Fuhr, D. Fenske and S. Gao, Chem.–Eur. J., 2012, 18, 915 CrossRef PubMed.
-
(a) J. Boeckmann, M. Wriedt and C. Näther, Chem.–Eur. J., 2012, 18, 5284 CrossRef PubMed;
(b) D. Shao, S. L. Zhang, X. H. Zhao and X. Y. Wang, Chem. Commun., 2015, 51, 4360 RSC;
(c) Y. Z. Zheng, W. Xue, M. L. Tong, X. M. Chen, F. Grandjean and G. J. Long, Inorg. Chem., 2008, 47, 4077 CrossRef PubMed.
-
(a) E. Bartolomé, J. Bartolomé, A. Arauzo, J. Luzón, L. Badía, R. Cases, F. Luis, S. Melnic, D. Prodius, S. Shova and C. Turta, J. Mater. Chem. C, 2016, 4, 5038 RSC;
(b) D. T. Thielemann, M. Klinger, T. J. A. Wolf, Y. Lan, W. Wernsdorfer, M. Busse, P. W. Roesky, A. N. Unterreiner, A. K. Powell, P. C. Junk and G. B. Deacon, Inorg. Chem., 2011, 50, 11990 CrossRef PubMed;
(c) Y. Z. Zheng, Y. Lan, W. Wernsdorfer, C. E. Anson and A. K. Powell, Chem.–Eur. J., 2009, 15, 12566 CrossRef PubMed.
-
(a) T. Liu, H. Zheng, S. Kang, Y. Shiota, S. Hayami, M. Mito, O. Sato, K. Yoshizawa, S. Kanegawa and C. Duan, Nat. Commun., 2013, 4, 2826 CrossRef;
(b) C. Papatriantafyllopoulou, S. Zartilas, M. J. Manos, C. Pichon, R. Clerac and A. J. Tasiopoulos, Chem. Commun., 2014, 50, 14873 RSC;
(c) A. Escuer, G. Vlahopoulou and F. A. Mautner, Inorg. Chem., 2011, 50, 2717 CrossRef PubMed.
-
(a) M. G. F. Vaz, R. A. A. Cassaro, H. Akpinar, J. A. Schlueter, P. M. Lahti and M. A. Novak, Chem.–Eur. J., 2014, 20, 5460 CrossRef PubMed;
(b) T. Han, W. Shi, Z. Niu, B. Na and P. Cheng, Chem.–Eur. J., 2013, 19, 994 CrossRef PubMed;
(c) Z. Tomkowicz, M. Rams, M. Balanda, S. Foro, H. Nojiri, Y. Krupskaya, V. Kataev, B. Buchner, S. K. Nayak, J. V. Yakhmi and W. Haase, Inorg. Chem., 2012, 51, 9983 CrossRef PubMed;
(d) K. Bernot, L. Bogani, A. Caneschi, D. Gatteschi and R. Sessoli, J. Am. Chem. Soc., 2006, 128, 7947 CrossRef PubMed.
-
(a) N. Hoshino, F. Iijima, G. N. Newton, N. Yoshida, T. Shiga, H. Nojiri, A. Nakao, R. Kumai, Y. Murakami and H. Oshio, Nat. Chem., 2012, 4, 921 CrossRef PubMed;
(b) L. M. Toma, C. Ruiz-Perez, J. Pasan, W. Wernsdorfer, F. Lloret and M. Julve, J. Am. Chem. Soc., 2012, 134, 15265 CrossRef PubMed;
(c) M. Rams, E. V. Peresypkina, V. S. Mironov, W. Wernsdorfer and K. E. Vostrikova, Inorg. Chem., 2014, 53, 10291 CrossRef PubMed;
(d) H. Miyasaka, T. Madanbashi, A. Saitoh, N. Motokawa, R. Ishikawa, M. Yamashita, S. Bahr, W. Wernsdorfer and R. Clerac, Chem.–Eur. J., 2012, 18, 3942 CrossRef PubMed.
-
(a) D. P. Zhang, L. F. Zhang, Y. T. Chen, H. L. Wang, Z. H. Ni, W. Wernsdorfer and J.
Z. Jiang, Chem. Commun., 2010, 46, 3550 RSC;
(b) J. F. Guo, X. T. Wang, B. W. Wang, G. C. Xu, S. Gao, L. Szeto, W. T.Wong, W. Y. Wong and T. C. Lau, Chem.–Eur. J., 2010, 16, 3524 CrossRef PubMed.
-
(a) X. Feng, J. Liu, T. D. Harris, S. Hill and J. R. Long, J. Am. Chem. Soc., 2012, 134, 7521 CrossRef PubMed;
(b) Y. Q. Zhang, C. L. Luo, X. B. Wu, B. W. Wang and S. Gao, Inorg. Chem., 2014, 53, 3503 CrossRef PubMed;
(c) E. V. Peresypkina, A. M. Majcher, M. Rams and K. E. Vostrikova, Chem. Commun., 2014, 50, 7150 RSC.
-
(a) V. Mougel, L. Chatelain, J. Hermle, R. Caciuffo, E. Colineau, F. Tuna, N. Magnani, A. de Geyer, J. Pecaut and M. Mazzanti, Angew. Chem., Int. Ed., 2014, 53, 819 CrossRef PubMed;
(b) J. W. Zhang, X. M. Kan, B. Q. Liu, G. C. Liu, A. X. Tian and X. L. Wang, Chem.–Eur. J., 2015, 21, 16219 CrossRef PubMed;
(c) L. Chatelain, F. Tuna, J. Pecaut and M. Mazzanti, Chem. Commun., 2015, 51, 11309 RSC.
-
(a) Z. X. Wang, X. Zhang, Y. Z. Zhang, M. X. Li, H. Zhao, M. Andruh and K. R. Dunbar, Angew. Chem., Int. Ed., 2014, 53, 11567 CrossRef PubMed;
(b) M. X. Yao, Q. Zheng, K. Qian, Y. Song, S. Gao and J. L. Zuo, Chem.–Eur. J., 2013, 19, 294 CrossRef PubMed;
(c) D. Visinescu, A. M. Madalan, M. Andruh, C. Duhayon, J. P. Sutter, L. Ungur, W. Van den Heuvel and L. F. Chibotaru, Chem.–Eur. J., 2009, 15, 11808 CrossRef PubMed.
-
(a) Y. Q. Wang, Q. Yue and E. Q. Gao, Chem.–Eur. J., 2017, 23, 896 CrossRef PubMed;
(b) Y. Q. Wang, W. W. Sun, Z. D. Wang, Q. X. Jia, E. Q. Gao and Y. Song, Chem. Commun., 2011, 47, 6386 RSC;
(c) Y. Q. Wang, X. M. Zhang, X. B. Li, B. W. Wang and E. Q. Gao, Inorg. Chem., 2011, 50, 6314 CrossRef PubMed;
(d) J. Y. Zhang, K. Wang, X. B. Li and E. Q. Gao, Inorg. Chem., 2014, 53, 9306 CrossRef PubMed;
(e) Q. X. Jia, H. Tian, J. Y. Zhang and E. Q. Gao, Chem.–Eur. J., 2011, 17, 1040 CrossRef PubMed;
(f) X. M. Zhang, Y. Q. Wang, K. Wang, E. Q. Gao and C. M. Liu, Chem. Commun., 2011, 47, 1815 RSC.
- X. B. Li, G. M. Zhuang, X. Wang, K. Wang and E. Q. Gao, Chem. Commun., 2013, 49, 1814 RSC.
- X. B. Li, J. Y. Zhang, Y. Q. Wang, Y. Song and E. Q. Gao, Chem.–Eur. J., 2011, 17, 13883 CrossRef PubMed.
-
(a) A. N. Pirogov, J. G. Park, A. S. Ermolenko, A. V. Korolev, A. G. Kuchin, S. Lee, Y. N. Choi, J. Park, M. Ranot, J. Yi, E. G. Gerasimov, Y. A. Dorofeev, A. P. Vokhmyanin, A. A. Podlesnyak and I. P. Swainson, Phys. Rev. B, 2009, 79, 174412 CrossRef;
(b) G. C. DeFotis, G. S. Coker, J. W. Jones, C. S. Branch, H. A. King, J. S. Bergman, S. Lee and J. R. Goodey, Phys. Rev. B, 1998, 58, 12178 CrossRef.
- J. P. Zhao, B. W. Hu, X. F. Zhang, Q. A. Yang, M. S. El Fallah, J. Ribas and X. H. Bu, Inorg. Chem., 2010, 49, 11325 CrossRef PubMed.
- Y. Q. Wang, Q. Yue, Y. Qi, K. Wang, Q. Sun and E. Q. Gao, Inorg. Chem., 2013, 52, 4259 CrossRef PubMed.
- Y. Q. Wang, A. L. Cheng, P. P. Liu and E. Q. Gao, Chem. Commun., 2013, 49, 6995 RSC.
- G. M. Sheldrick, Program for Empirical Absorption Correction of Area Detector Data, University of Göttingen, Germany, 1996 Search PubMed.
- G. M. Sheldrick, SHELXTL Version 5.1, Bruker Analytical X-ray Instruments Inc., Madison, Wisconsin, USA, 1998 Search PubMed.
-
(a) O. Kahn, Molecular Magnetism, Wiley-VCH, Weinheim, 1993 Search PubMed;
(b) R. L. Carlin, Magnetochemistry, Springer, Berlin, 1986 CrossRef;
(c) F. Lloret, M. Julve, J. Cano, R. Ruiz-García and E. Pardo, Inorg. Chim. Acta, 2008, 361, 3432 CrossRef.
- J. A. Mydosh, Spin Glasses: An Experimental Introduction, Taylor & Francis, London, 1993 Search PubMed.
-
(a) T. F. Liu, D. Fu, S. Gao, Y. Z. Zhang, H. L. Sun, G. Su and Y. J. Liu, J. Am. Chem. Soc., 2003, 125, 13976 CrossRef PubMed;
(b) E. Coronado, J. R. Galán-Mascarós and C. Martí-Gastaldo, J. Am. Chem. Soc., 2008, 130, 14987 CrossRef PubMed.
- K. S. Cole and R. H. Cole, J. Chem. Phys., 1941, 9, 341 CrossRef.
Footnote |
† Electronic supplementary information (ESI) available. CCDC 1832808. For ESI and crystallographic data in CIF or other electronic format see DOI: 10.1039/c8ra03273d |
|
This journal is © The Royal Society of Chemistry 2018 |