DOI:
10.1039/C8RA03075H
(Paper)
RSC Adv., 2018,
8, 17132-17138
Synthesis and photoluminescence properties of Eu3+-activated LiCa3ZnV3O12 white-emitting phosphors
Received
10th April 2018
, Accepted 4th May 2018
First published on 10th May 2018
Abstract
Single-component white-emitting phosphors are highly promising for applications in phosphor-converted white light-emitting diodes. In this paper, novel single-phase LiCa3(1−x)ZnV3O12:Eu3+ (x = 0–0.05) phosphors with tunable white emissions were prepared by a conventional solid-state reaction. The LiCa3ZnV3O12 (x = 0) phosphor showed an efficient self-activated bluish-green emission due to the V5+–O2− charge transfer transition of the [VO4]3− groups, and possessed an intense broad excitation spectrum in the 250–400 nm wavelength range. Together with the [VO4]3− emission, the red emission of Eu3+ ions was also observed in LiCa3(1−x)ZnV3O12:Eu3+ phosphors. The energy transfer from the [VO4]3− groups to the Eu3+ ions was studied. Importantly, the emission colors of LiCa3(1−x)ZnV3O12:Eu3+ phosphors varied from greenish-blue to whitish and then to red with increasing Eu3+ content and the white-light emission was realized in the single-phase phosphor of LiCa3ZnV3O12:0.003Eu3+ by combining the [VO4]3−-emission and the Eu3+-emission. The energy-transfer efficiency from [VO4]3− groups to Eu3+ ions in the LiCa3ZnV3O12:0.003Eu3+ sample was determined to be about 52% and the internal quantum efficiency of the LiCa3ZnV3O12:0.003Eu3+ phosphor was found to be about 41.5%. In addition, the CIE chromaticity coordinates of LiCa3ZnV3O12:0.003Eu3+ were (x = 0.3374, y = 0.3596), and the correlated color temperature was estimated to be about 5311 K.
1. Introduction
Solid-state lighting based on white light-emitting diodes (WLEDs) is considered as a next-generation lighting source for general illumination because it offers some particularly intriguing advantages over conventional lighting sources, including outstanding energy efficiency, long working lifetime, eco-friendliness, small size, and fast response time.1–10 To realize pure white emissions for WLEDs, a common strategy at present is coating phosphors onto the surface of LED chips. Such so-called phosphor-converted WLEDs are generally fabricated through three approaches: (1) combining a blue LED chip with a yellow phosphor (e.g., YAG:Ce3+) or a blend of green and red phosphors, (2) combining an ultraviolet (UV) LED chip with a blend of tricolor (blue, green, and red) phosphors, and (3) combining an UV LED chip with a single-phased white-emitting phosphor.11–14 Nowadays, the widely used WLEDs are fabricated by coating YAG:Ce3+ yellow phosphors on InGaN blue LED chips, however these WLEDs commonly suffer from high correlated color temperature (CCT) and low color rendering index (CRI) owing to the lack of a red component, limiting their applications in indoor lighting.15–21 On the other hand, the WLEDs made by multiple-emitting phosphors also have some drawbacks, such as intrinsic color balance, device complication and high cost.22–28 Compared to former two approaches, the latest approach is highly promising, due to its high luminescence efficiency, color repeatability, and low manufacturing costs.29–31 Therefore, it is urgent to develop high-efficiency single-component white-light-emitting materials.
A powerful way to realize tunable white emissions from single host materials is to control the energy transfer from the sensitizers to the activators.32 Based on the principle of energy transfer, various single-component white-emitting phosphors were developed recently. However, these white-emitting phosphors are usually fabricated with multiple dopants including Ce3+–Mn2+,33 Eu2+–Mn2+,34 Ce3+–Tb3+–Mn2+,24 and Eu2+–Tb3+–Mn2+,35,36 which require complicate sample-preparation procedures (such as reducing atmosphere) and thus result in significant increase in cost. In sharp contrast, so far there are still few reports on single-activator-doped white-emitting phosphors. In 2007, Li and Lin reported white emission from CaIn2O4:1.0%Eu3+ phosphors due to the 5D0,1,2,3 → 7FJ (J = 0, 1, 2, 3, 4) transitions, but its internal quantum efficiency (IQE) was very low (only 10%).37 In 2011, Shi and co-workers demonstrated white emission in K2YZr(PO4)3:Eu3+ phosphors by combining the Zr4+ greenish-blue emission and the Eu3+ red emission, while the value of IQE was not given.38 In 2013, Qian et al. reported white light-emitting Y2WO6:Sm3+ phosphor with low IQE of 21.65%, and the white emission was achieved by combining the green emission from the host with the orange-red emission from the Sm3+ dopant.39
Recently, vanadates with the self-activated photoluminescence have gained much attention for potential in near-UV-excited white LEDs, as they possess broad and intense charge transfer (CT) absorption bands in the near-UV wavelength range and can exhibit broadband visible emissions corresponding to the V5+–O2− CT transition.40–50 Recently, several groups reported white-light emissions from Eu3+-activated vanadate-based phosphors, such as Sr2.91V2O8:0.06Eu3+,51 and Sr2V2O7:Eu3+.41 However, no value of the IQE was given. Just recently, Hasegawa and co-workers reported the bluish white emission from the LiCa3ZnV3O12 (abbreviated as: LCZV) host with high IQE.52 To the best of our knowledge, the luminescence properties of Eu3+-doped LCZV have not been reported in the literature.
In this paper, we reported the synthesis and luminescence properties of a novel high-efficiency single-component white-emitting LCZV:Eu3+ phosphor. A series of LCZV:Eu3+ phosphors with different Eu3+ doping concentrations were prepared by conventional solid-state reaction under air atmosphere (no need the reducing atmosphere). Amazingly, these phosphors have intense broad excitation bands in 250–400 nm range peaking at 343 nm due to the [VO4]3− groups. Moreover, energy transfer from the LCZV host to Eu3+ ions took place. Under 343 nm excitation, LCZV:Eu3+ phosphors gave rise to bright tunable white light by combining the broad bluish-green emission band peaking at 500 nm of LCZV host and the red emission lines of Eu3+ ions. Importantly, the LCZV:0.003Eu3+ white-light-emitting sample showed a high IQE of 41.5% along with CIE chromaticity coordinates of (x = 0.3374, y = 0.3596) and a CCT = 5311 K.
2. Experimental
LiCa3(1−x)ZnV3O12:xEu3+ (x = 0, 0.001, 0.003, 0.005, 0.01, 0.02, and 0.05) powder phosphors were prepared via a conventional high-temperature solid-state reaction technique. The raw materials of CaCO3 (analytical reagent, AR), Li2CO3 (AR), ZnO (AR), NH4VO3 (AR), and Eu2O3 (99.99%) were weighed based on stoichiometric ratios and ground in an agate mortar. Subsequently, the obtained mixtures were putted into the alumina crucibles and pre-sintered in a furnace at 750 °C for 6 h in air, and then the obtained powders were re-ground and sintered again at 850 °C for 6 h. The final products were ground into fine powders and used for characterizations.
The X-ray diffraction (XRD) patterns were recorded on a Bruker D8 X-ray diffractometer using Cu Kα radiation. The photoluminescence (PL) and photoluminescence excitation (PLE) spectra were recorded with an Edinburgh FS5 spectrometer equipped with a 150 W continued-wavelength xenon lamp as excitation source. The IQE of the phosphors was measured on an Edinburgh FS5 spectrometer equipped with a barium sulfate-coated integrating sphere.
3. Results and discussion
Fig. 1 presents the representative XRD patterns of LCZV:xEu3+ (x = 0.001, 0.005, and 0.05) samples. For the samples doped with low Eu3+ concentrations (x = 0.001 and 0.005), all the diffraction peaks can be indexed to standard data of LiCa3MgV3O12 (JCPDS no. 24-1212). However, for the LCZV:0.05Eu3+ sample, a small diffraction peak at 24.58° due to the EuVO4 impurity (JCPDS no. 15-0809) was presented. Since the radii of Ca2+ (1.12 Å, coordinate number (CN) = 8) and Eu3+ ions (1.06 Å, CN = 8) were similar,45 Eu3+ dopants in the LCZV:Eu3+ phosphors are likely to replace the Ca2+ sites. The LCZV:Eu3+ phosphors crystallized into the pure cubic phase with space group Ia
d.
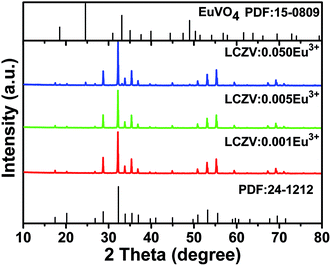 |
| Fig. 1 XRD patterns of LCZV:xEu3+ samples. | |
Fig. 2 shows the diffuse reflectance spectra of LCZV:xEu3+ (x = 0 and 0.003) phosphors. It can be seen that for both samples, a strong broad absorption band was presented in the 200–400 nm near-UV wavelength, which was assigned to the ligand to metal CT transition between V5+–O2− in the [VO4] tetrahedral.45,52 However, due to the low doping concentration of Eu3+ ions, the absorption peaks of Eu3+ ions were not observed.53
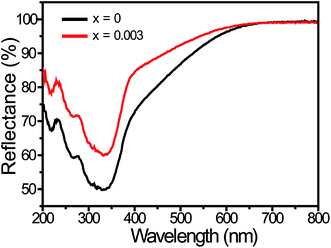 |
| Fig. 2 Diffuse reflectance spectra of the LCZV:xEu3+ (x = 0 and 0.003) phosphors. | |
The CLZV host belongs to a self-activated bluish-green emitting phosphor. Fig. 3 presents the PLE and PL spectra of the LCZV host. As shown in Fig. 3(a), the PLE spectrum monitoring at 520 nm exhibited an intense broad band in the 250–400 nm wavelength range with a maximum at 343 nm, which agreed well with the diffuse reflectance spectrum of the LCZV host. Moreover, by using Gaussian fitting, the PLE band consisted of two sub-bands corresponding to the 1A1 → 1T2 (Ex1; 309 nm) and 1A1 → 1T1 (Ex2; 346 nm) transitions of [VO4]3− groups.40,42,52 Upon 343 nm excitation, the CLMV host gave rise to a strong broad bluish-green emission band in the 370–750 nm wavelength region peaking at about 500 nm, as demonstrated in Fig. 3(b). Meanwhile, by using the Gaussian fitting, the PL band was composed of two sub-bands due to 3T2 → 1A1 (Em1; 484 nm) and 3T1 → 1A1 (Em2; 555 nm) transitions of [VO4]3− groups.40,42,52
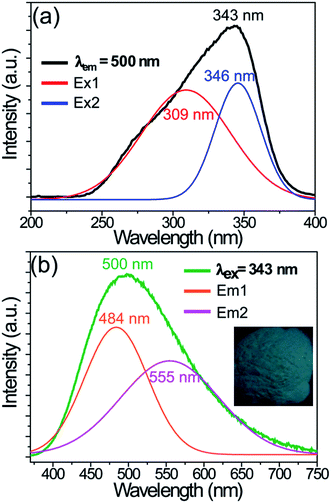 |
| Fig. 3 PLE (a) and PL (b) spectra of the LCZV host material. Inset shows the digital photo of the sample under a 365 nm UV lamp. | |
Fig. 4 shows the PLE and PL spectra of the LCZV:0.003Eu3+ phosphors. The PLE spectrum was monitored at 609 nm corresponding to the 5D0 → 7F2 transition of Eu3+ ions and it consisted of a strong broad PLE band in the 250–400 nm wavelength range due to V5+–O2− CT transition of [VO4]3− groups. Besides, a relatively weak sharp PLE peak at 394 nm corresponding to the 7F0 → 5L6 transition of Eu3+ ions was also presented in the PLE spectrum. These observations indicated that energy transfer from [VO4]3− groups to Eu3+ ions in LCZV:Eu3+ phosphors took place. Under 343 nm excitation, the LCZV:0.003Eu3+ showed bright white emission, as shown in Fig. 4. The obtained PL spectrum exhibited a broad emission band with a maximum at 500 nm due to the [VO4]3− groups and several sharp emission lines of Eu3+ ions (5D0 → 7F1 transition at 589 nm, 5D0 → 7F2 transition at 609 nm, 5D0 → 7F3 transition at 652 nm, and 5D0 → 7F4 transition at 708 nm).54–58 The energy transfer efficiency (ηET) of [VO4]3− → Eu3+ in LCZV:0.003Eu3+ sample can be calculated by using the equation below:59
|
 | (1) |
where
I0 and
I represent the PL intensities of LCZV host and LCZV:0.003Eu
3+ phosphors, respectively. Then, the
ηET was calculated to be about 52%.
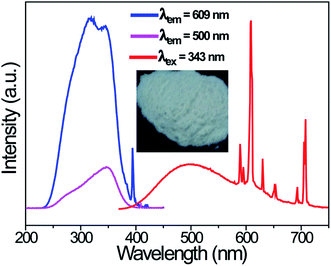 |
| Fig. 4 PLE (λem = 500 and 609 nm) and PL (λex = 343 nm) spectra of the LCZV:0.003Eu3+ phosphors. Inset shows the photograph of the sample under a 365 nm UV lamp. | |
Fig. 5 schematically shows the energy transfer process from [VO4]3− to Eu3+ ions in LCZV:Eu3+ phosphors. Upon 343 nm UV light excitation into the [VO4]3− groups, electrons are then excited from the ground state 1A1 into the 1T1 and 1T2 higher-excited levels. Subsequently, the excited electrons at the 1T1 and 1T2 levels relax to the 3T2 and 3T1 lower-excited levels via nonradiative relaxation processes and then return to the ground state, thus giving rise to the bluish-green emissions corresponding to the 3T2 → 1A1 and 3T1 → 1A1 transitions. Once Eu3+ ions are doped into LCZV host, partial excited energy of [VO4]3− groups at the 3T1 level would be transferred to the 5D0 level of nearby Eu3+ ions through the nonradiative resonant process, finally leading to the population of the 5D4 excited levels and subsequently the red emissions due to the 5D0 → 7F1,2,3,4 transitions. By controlling the Eu3+ doping concentration, the energy transfer efficiency from [VO4]3− to Eu3+ ions can be adjusted, and thus intensity ratios of bluish-green and red emissions could be manipulated. Accordingly, tunable white emissions would be achieved.
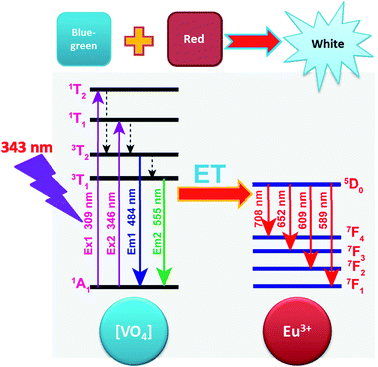 |
| Fig. 5 Energy transfer scheme for the tunable white emissions in LCZV:Eu3+ phosphors. ET denotes energy transfer. | |
In order to realize tunable white emissions, a series of Eu3+-activated LCZV phosphors with various Eu3+ doping concentrations were prepared. Fig. 6(a) shows the PL spectra of LCZV:xEu3+ (x = 0, 0.001, 0.003, 0.005, 0.01, 0.02, and 0.05) phosphors under 343 nm excitation. It can be seen that with increasing Eu3+ doping concentrations, the PL intensity of the blue-green emission from LCZV host gradually reduced, further confirming the energy-transfer from LCZV host to Eu3+ activators. In contrast, with the increasing Eu3+ concentrations in LCZV:xEu3+, the PL emission intensity of Eu3+ ions gradually increased first and reached the maximum value at x = 0.02; whereas further increasing the Eu3+ doping concentration resulted in reduced intensity of Eu3+ red emissions owing to the concentration quenching effect (see Fig. 6(b)). Generally, the occurrence of concentration quenching comes from the energy migration among the activators until energy sink in the host lattice is reached.60 The probability of energy migration is inversely proportional to Rn, where R is the distance between the activator ions and n is an integer. The critical average distance (Rc) among Eu3+ ions in the LCZV host lattice can be roughly calculated by using the following expression:61
|
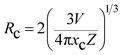 | (2) |
where
Rc is the critical distance,
V is the volume of the unit cell,
xc is the critical doping concentration, and
Z is the number of formula units per unit cell. In this present work, the
xc = 0.02;
V = 1926.86 Å
3; and
Z = 8.
52 The critical average distance
Rc for Eu
3+ ions in LCZV host material was determined to be about 28.44 Å.
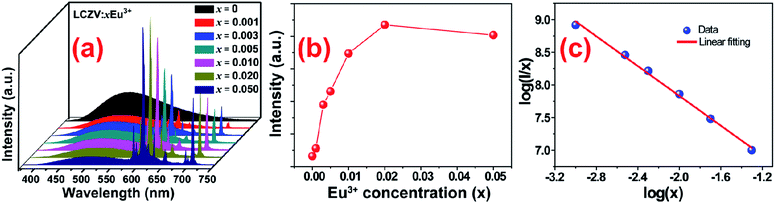 |
| Fig. 6 (a) PL spectra of LCZV:xEu3+ (x = 0, 0.001, 0.003, 0.005, 0.01, 0.02, and 0.05) phosphors under 343 nm excitation. (b) The intensity of 609 nm emission as a function of Eu3+ concentration in LCZV:xEu3+ phosphors. (c) The plot of log(I/x) vs. log(x) in LCZV:xEu3+ phosphors. | |
As well-known, nonradiative energy migration among luminescence activators can take place through exchange interaction or multipole–multipole interaction. The exchange interaction only works if the distance between activators is shorter than 5 Å. In our case, the Rc = 28.44 Å, and thus electric multipolar interaction would be the dominant energy transfer mechanism between Eu3+ ions in LCZV host. The type of interaction mechanism can be determined by the following equation:62
|
log(I/x) = A − (θ/3)log x
| (3) |
where
I is the PL intensity,
x is the dopant concentration,
A is concentration, and
θ = 6, 8 and 10 refers to dipole–dipole, dipole-quadrupole and quadrupole–quadrupole interactions, respectively.
Fig. 6(c) shows the plot of log(
I/
x)
vs. log(
x). The experimental data can be well-fitted into linear line with a slop of −1.14. Accordingly,
θ value was calculated to be 3.42, and this value was close to 3. This result indicated that the nonradiative energy transfer among the nearest-neighbor ions was the mechanism of Eu
3+ concentration quenching effect in LCZV:Eu
3+ phosphors.
63 Similar results also have been previously reported in Eu
3+-activated Li
3Gd
3Te
2O
12, Ba
3La(PO
4)
3, and Sr
1.7Zn
0.3CeO
4 phosphors.
64–66
The values of CIE chromaticity coordinates of LCZV:xEu3+ phosphors were shown in Fig. 7 and Table 1. Obviously, the emission colors of LCZV:xEu3+ phosphors can be readily tuned from bluish-green (x = 0.2648, y = 0.3608) to white (x = 0.3374, y = 0.3596), and then to red (x = 0.4673, y = 0.3639) by raising the Eu3+ doping concentrations, which can be attributed to the energy transfer from the [VO4]3− groups to Eu3+ ions. Particularly, by controlling the Eu3+ doping concentration, white emissions were achieved in single-phased LCZV:xEu3+ phosphors through the suitable combination of the [VO4]3− bluish-green emissions and the Eu3+ red emissions. Based on these values of CIE chromaticity coordinates, the CCTs for these tunable emissions were calculated by using the following equation:67
|
CCT = 437n3 + 3601n2 − 6861n + 5541.31
| (4) |
where
n = (
x −
xc)/(
y −
yc), and the (
xc,
yc) of chromaticity epicenter is (0.3320, 0.1858). The calculated CCT values were also listed in
Table 1. For the LCZV:0.003Eu
3+ white-emitting phosphors, the CCT was found to be about 5311 K.
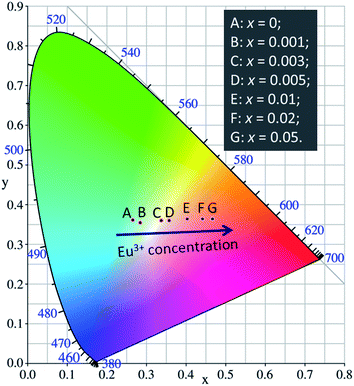 |
| Fig. 7 CIE chromaticity diagram for LCZV:xEu3+ phosphors with various doping concentrations of Eu3+ ions. | |
Table 1 CIE chromaticity coordinates and CCTs of LCZV:xEu3+ phosphors with different Eu3+ doping concentrations
Sample (x) |
CIE (x, y) |
CCT (K) |
0 |
(0.2648, 0.3608) |
8690 |
0.001 |
(0.2841, 0.3549) |
7749 |
0.003 |
(0.3374, 0.3596) |
5311 |
0.005 |
(0.3570, 0.3601) |
4612 |
0.01 |
(0.4022, 0.3634) |
3345 |
0.02 |
(0.4417, 0.3642) |
2551 |
0.05 |
(0.4673, 0.3639) |
2173 |
As well-known, the IQE is an important factor for evaluating the performance of inorganic luminescent materials. Thus, we have measured the IQEs of LCZV host material and the white-emitting LCZV:0.003Eu3+ phosphors (see Fig. 8), and their values were calculated by using the following expression,68,69
|
 | (5) |
Here
η is the IQE,
LS shows the emission spectrum of the studied samples,
ER and
ES present the PLE spectra of the excitation light with and without synthesized compounds, respectively. Accordingly, the IQEs of the LCZV host and white-emitting LCZV:0.003Eu
3+ phosphors were determined to be about 31.2% and 41.5% under 343 nm excitation. Significantly, the value of the IQE for LCZV:0.003Eu
3+ sample was much higher than several previous reported white-emitting phosphors, such as CaIn
2O
4:Eu
3+ (IQE: 10%),
37 and Y
2WO
6:Sm
3+ (IQE: 21.65%).
39 It should be noted that the IQE would be further enhanced by optimizing the synthesis process.
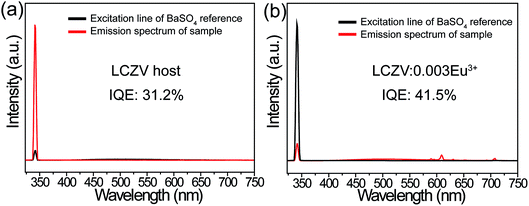 |
| Fig. 8 Excitation line of BaSO4 and the emission spectrum of LCZV host collected using an integrating sphere. (b) Excitation line of BaSO4 and the emission spectrum of LCZV:0.003Eu3+ phosphors collected using an integrating sphere. | |
4. Conclusions
In summary, in this work, self-activated LCZV host material and Eu3+ singly doped LCZV phosphors were prepared by a high-temperature solid-state reaction and their PL properties were investigated. It was found that the LCZV host was a self-activated phosphor. The PLE spectrum of LCZV host consisted of a broad band in the 250–400 nm range, while its PL spectrum excited at 343 nm included a broad band in the greenish-blue region peaking at 500 nm. Interestingly, doping Eu3+ ions into LCZV host induced tunable color emissions from LCZV:Eu3+ phosphors via raising Eu3+ doping concentrations and the white-light emission was realized by combining the [VO4]3− greenish-blue emission with the Eu3+ red emission. The LCZV:0.003Eu3+ phosphors emitted bright white light with CIE chromaticity coordinates of (x = 0.3374, y = 0.3596) and CCT = 5311 K. The energy transfer efficiency from [VO4]3− groups to Eu3+ ions in LCZV:0.003Eu3+ phosphors was calculated to be 52%. The IQE of LCZV:0.003Eu3+ phosphors was determined to be about 41.5%. Our results demonstrated these single-phased white-emitting phosphors would find potential applications in lighting and display systems.
Conflicts of interest
There are no conflicts to declare.
Acknowledgements
This work was supported by the National Natural Science Foundation of China (no. 51502190), the Program for the Outstanding Innovative Teams of Higher Learning Institutions of Shanxi, and the Open Fund of the State Key Laboratory of Luminescent Materials and Devices (South China University of Technology, no. 2017-skllmd-01).
References
- X. Zhang, B. Xu, J. Zhang, Y. Gao, Y. Zheng, K. Wang and X. W. Sun, Adv. Funct. Mater., 2016, 26, 4595–4600 CrossRef CAS.
- S. Pimputkar, J. S. Speck, S. P. DenBaars and S. Nakamura, Nat. Photonics, 2009, 3, 180–182 CrossRef CAS.
- P. Pust, P. J. Schmidt and W. Schnick, Nat. Mater., 2015, 14, 454 CrossRef CAS PubMed.
- R. Haitz and J. Y. Tsao, Phys. Status Solidi A, 2011, 208, 17–29 CrossRef CAS.
- X. Li, Y. Liu, X. Song, H. Wang, H. Gu and H. Zeng, Angew. Chem., Int. Ed., 2015, 54, 1759–1764 CrossRef CAS PubMed.
- X. Huang, B. Li and H. Guo, J. Alloys Compd., 2017, 695, 2773–2780 CrossRef CAS.
- X. Huang and H. Guo, Dyes Pigm., 2018, 152, 36–42 CrossRef CAS.
- J. Liang, P. Du, H. Guo, L. Sun, B. Li and X. Huang, Dyes Pigm., 2018, 157, 40–46 CrossRef CAS.
- J. Han, L. Li, M. Peng, B. Huang, F. Pan, F. Kang, L. Li, J. Wang and B. Lei, Chem. Mater., 2017, 29, 8412–8424 CrossRef CAS.
- J. Zhong, D. Chen, Y. Zhou, Z. Wan, M. Ding and Z. Ji, J. Eur. Ceram. Soc., 2016, 36, 1705–1713 CrossRef CAS.
- P. Pust, V. Weiler, C. Hecht, A. Tücks, A. S. Wochnik, A.-K. Henß, D. Wiechert, C. Scheu, P. J. Schmidt and W. Schnick, Nat. Mater., 2014, 13, 891–896 CrossRef CAS PubMed.
- X. Huang, Nat. Photonics, 2014, 8, 748–749 CrossRef CAS.
- R. Cao, W. Wang, J. Zhang, S. Jiang, Z. Chen, W. Li and X. Yu, J. Alloys Compd., 2017, 704, 124–130 CrossRef CAS.
- W. Dai, Y. Lei, J. Zhou, Y. Zhao, Y. Zheng, M. Xu, S. Wang and F. Shen, J. Am. Ceram. Soc., 2017, 100, 5174–5185 CrossRef CAS.
- H. Zhu, C. C. Lin, W. Luo, S. Shu, Z. Liu, Y. Liu, J. Kong, E. Ma, Y. Cao, R.-S. Liu and X. Chen, Nat. Commun., 2014, 5, 4312 CAS.
- S. S. Liang, M. M. Shang, H. Z. Lian, K. Li, Y. Zhang and J. Lin, J. Mater. Chem. C, 2016, 4, 6409–6416 RSC.
- M. Peng, X. Yin, P. A. Tanner, C. Liang, P. Li, Q. Zhang and J. Qiu, J. Am. Ceram. Soc., 2013, 96, 2870–2876 CrossRef CAS.
- M. Peng, X. Yin, P. A. Tanner, M. G. Brik and P. Li, Chem. Mater., 2015, 27, 2938–2945 CrossRef CAS.
- R. Cao, Y. Ye, Q. Peng, G. Zheng, H. Ao, J. Fu, Y. Guo and B. Guo, Dyes Pigm., 2017, 146, 14–19 CrossRef CAS.
- Y.-Y. Zhou, E.-H. Song, T.-T. Deng and Q.-Y. Zhang, ACS Appl. Mater. Interfaces, 2018, 10, 880–889 CAS.
- M. Zhu, Y. Pan, Y. Huang, H. Lian and J. Lin, J. Mater. Chem. C, 2018, 6, 491–499 RSC.
- Y. Jia, R. Pang, H. Li, W. Sun, J. Fu, L. Jiang, S. Zhang, Q. Su, C. Li and R.-S. Liu, Dalton Trans., 2015, 44, 11399–11407 RSC.
- F. Kang, Y. Zhang and M. Peng, Inorg. Chem., 2015, 54, 1462–1473 CrossRef CAS PubMed.
- X. Zhang and M. Gong, Dalton Trans., 2014, 43, 2465–2472 RSC.
- J. Ding, Q. Wu, Y. Li, Q. Long, C. Wang and Y. Wang, Dalton Trans., 2015, 44, 9630–9636 RSC.
- M. Jiao, Y. Jia, W. Lu, W. Lv, Q. Zhao, B. Shao and H. You, Dalton Trans., 2014, 43, 3202–3209 RSC.
- Y. Liu, G. Liu, J. Wang, X. Dong and W. Yu, Inorg. Chem., 2014, 53, 11457–11466 CrossRef CAS PubMed.
- A. Huang, Z. Yang, C. Yu, Z. Chai, J. Qiu and Z. Song, J. Phys. Chem. C, 2017, 121, 5267–5276 CAS.
- Y. Woo Seo, S. Heum Park, S. Hyoung Chang, J. Hyun Jeong, K. Ho Kim and J.-S. Bae, Ceram. Int., 2017, 43, 8497–8501 CrossRef.
- M. Jiao, Y. Jia, W. Lu, W. Lv, Q. Zhao, B. Shao and H. You, J. Mater. Chem. C, 2014, 2, 90–97 RSC.
- C. Zhou, Y. Tian, O. Khabou, M. Worku, Y. Zhou, J. Hurley, H. Lin and B. Ma, ACS Appl. Mater. Interfaces, 2017, 9, 40446–40451 CAS.
- K. Li, M. M. Shang, H. Z. Lian and J. Lin, J. Mater. Chem. C, 2016, 4, 5507–5530 RSC.
- G. Li, D. Geng, M. Shang, C. Peng, Z. Cheng and J. Lin, J. Mater. Chem., 2011, 21, 13334–13344 RSC.
- N. Guo, Y. Huang, M. Yang, Y. Song, Y. Zheng and H. You, Phys. Chem. Chem. Phys., 2011, 13, 15077–15082 RSC.
- W. Lü, Z. Hao, X. Zhang, Y. Luo, X. Wang and J. Zhang, Inorg. Chem., 2011, 50, 7846–7851 CrossRef PubMed.
- W.-R. Liu, C.-H. Huang, C.-W. Yeh, Y.-C. Chiu, Y.-T. Yeh and R.-S. Liu, RSC Adv., 2013, 3, 9023–9028 RSC.
- X. Liu, C. Lin and J. Lin, Appl. Phys. Lett., 2007, 90, 081904 CrossRef.
- L. Shi and H. J. Seo, Opt. Express, 2011, 19, 7147–7152 CrossRef CAS PubMed.
- H. Qian, J. Zhang and L. Yin, RSC Adv., 2013, 3, 9029–9034 RSC.
- T. Nakajima, M. Isobe, T. Tsuchiya, Y. Ueda and T. Manabe, J. Phys. Chem. C, 2010, 114, 5160–5167 CAS.
- W.-Q. Yang, H.-G. Liu, M. Gao, Y. Bai, J.-T. Zhao, X.-D. Xu, B. Wu, W.-C. Zheng, G.-K. Liu and Y. Lin, Acta Mater., 2013, 61, 5096–5104 CrossRef CAS.
- J. Zhou, F. Huang, J. Xu, H. Chen and Y. Wang, J. Materi. Chem. C, 2015, 3, 3023–3028 RSC.
- T. Nakajima, M. Isobe, Y. Uzawa and T. Tsuchiya, J. Materi. Chem. C, 2015, 3, 10748–10754 RSC.
- R. Yu, J. H. Jeong and Y.-F. Wang, J. Am. Ceram. Soc., 2017, 100, 5649–5658 CrossRef CAS.
- P. Du and J. S. Yu, Dyes Pigm., 2017, 147, 16–23 CrossRef CAS.
- X. Y. Huang, J. X. Wang, D. C. Yu, S. Ye, Q. Y. Zhang and X. W. Sun, J. Appl. Phys., 2011, 109, 113526 CrossRef.
- R. Cao, D. Peng, H. Xu, Z. Luo, H. Ao, S. Guo and J. Fu, Optik, 2016, 127, 7896–7901 CrossRef CAS.
- R. Cao, D. Peng, H. Xu, S. Jiang, T. Fu, W. Luo and Z. Luo, Spectrochim. Acta, Part A, 2015, 150, 465–469 CrossRef CAS PubMed.
- F. Kang, H. Zhang, L. Wondraczek, X. Yang, Y. Zhang, D. Y. Lei and M. Peng, Chem. Mater., 2016, 28, 2692–2703 CrossRef CAS.
- F. Kang, M. Peng, D. Y. Lei and Q. Zhang, Chem. Mater., 2016, 28, 7807–7815 CrossRef CAS.
- S. J. Yoon and K. Park, Opt. Mater., 2014, 36, 1305–1310 CrossRef CAS.
- T. Hasegawa, Y. Abe, A. Koizumi, T. Ueda, K. Toda and M. Sato, Inorg. Chem., 2018, 57, 857–866 CrossRef CAS PubMed.
- X. Zhou, L. Chen, S. Jiang, G. Xiang, L. Li, X. Tang, X. Luo and Y. Pang, Dyes Pigm., 2018, 151, 219–226 CrossRef CAS.
- X. Huang, Dyes Pigm., 2016, 130, 99–105 CrossRef CAS.
- X. Huang, B. Li, H. Guo and D. Chen, Dyes Pigm., 2017, 143, 86–94 CrossRef CAS.
- P. Du, X. Huang and J. S. Yu, Chem. Eng. J., 2018, 337, 91–100 CrossRef CAS.
- B. Li and X. Huang, Ceram. Int., 2018, 44, 4915–4923 CrossRef CAS.
- X. Huang, S. Wang, B. Li, Q. Sun and H. Guo, Opt. Lett., 2018, 43, 1307–1310 CrossRef PubMed.
- B. Li, X. Huang, H. Guo and Y. Zeng, Dyes Pigm., 2018, 150, 67–72 CrossRef CAS.
- C.-K. Chang and T.-M. Chen, Appl. Phys. Lett., 2007, 91, 081902 CrossRef.
- G. Blasse, Phys. Lett. A, 1968, 28, 444–445 CrossRef CAS.
- D. L. Dexter, J. Chem. Phys., 1953, 21, 836–850 CrossRef CAS.
- Q. Shao, H. Ding, L. Yao, J. Xu, C. Liang and J. Jiang, RSC Adv., 2018, 8, 12035–12042 RSC.
- H. Deng, Z. Gao, N. Xue, J. H. Jeong and R. Yu, J. Lumin., 2017, 192, 684–689 CrossRef CAS.
- R. Yu, H. M. Noh, B. K. Moon, B. C. Choi, J. H. Jeong, K. Jang, S. S. Yi and J. K. Jang, J. Alloys Compd., 2013, 576, 236–241 CrossRef CAS.
- H. Li, R. Zhao, Y. Jia, W. Sun, J. Fu, L. Jiang, S. Zhang, R. Pang and C. Li, ACS Appl. Mater. Interfaces, 2014, 6, 3163–3169 CAS.
- C. S. McCamy, Color Res. Appl., 1992, 17, 142–144 CrossRef.
- X. Huang, B. Li, P. Du, H. Guo, R. Cao, J. S. Yu, K. Wang and X. W. Sun, Dyes Pigm., 2018, 151, 202–210 CrossRef CAS.
- H. Guo, X. Huang and Y. Zeng, J. Alloys Compd., 2018, 741, 300–306 CrossRef CAS.
|
This journal is © The Royal Society of Chemistry 2018 |
Click here to see how this site uses Cookies. View our privacy policy here.