DOI:
10.1039/C8RA02991A
(Paper)
RSC Adv., 2018,
8, 20712-20718
Continuous hydrogen production from glucose/xylose by an anaerobic sequential batch reactor to maximize the energy recovery efficiency
Received
7th April 2018
, Accepted 18th May 2018
First published on 6th June 2018
Abstract
Fermentation of both glucose and xylose is essential to realize efficient bioconversion of renewable and abundant lignocellulosic biomass to hydrogen. In this study, a mixture of glucose and xylose at different ratios was used as a substrate for biological hydrogen production by an anaerobic sequential batch reactor (ASBR). An average glucose and xylose consumption of 80% and 50% with a high hydrogen production rate of 7.1 ± 0.9 mmol L−1 h−1 was obtained, respectively. Hydraulic retention time (HRT) played a critical role in hydrogen production at high glucose to xylose ratios. A maximum hydrogen production rate of 8.9 mmol L−1 h−1 was achieved at an optimized HRT of 12 h with a high glucose and xylose consumption of 92.2% and 82.2%, respectively. Upon further energy conversion analysis, continuous hydrogen production by ASBR provided the maximum energy conversion efficiency of 21.5%. These results indicate that ASBR can effectively accelerate the hydrogen production rate, improve substrate consumption regardless of the glucose to xylose ratio, and thus provides a new direction for efficient hydrogen production from lignocellulosic feedstock.
Introduction
Replacement of fossil fuels with biofuels has become the fundamental and growing part of the world's ongoing energy transformation.1 Currently, cost-effective lignocellulosic biological hydrogen gas (bio-H2) is a popular target. On the one hand, H2 has high energy content, and as a biofuel, it does not cause CO2 emission;1 on the other hand, as a substrate for bio-H2 production, lignocellulosic feedstock is abundant, renewable and does not compete for land used for food or feed production.2 In the past few decades, efforts made on the biological conversion of lignocellulose to H2 were mainly focused on breaking the recalcitrant heteropolymeric structure of lignocellulose as well as liberating monomeric sugars (mainly a mixture of glucose and xylose despite the variations in the carbohydrate composition of different lignocelluloses) embedded in cellulose and hemicellulose to the maximum. However, when both glucose and xylose are present, most microorganisms generally metabolize glucose only because of the carbon catabolite repression effect.1,3,4 Consequently, during pretreatment and hydrolysis procedures, process efficiency, capital investment, energy consumption, inhibited product generation, and environmental impact should be considered, and whether the sugar composition can satisfy the following bio-H2 fermentation also needs to be taken into account; this greatly restricts the selection of pretreatment and hydrolysis technology.5
To cope with this issue, bacteria that can naturally ferment both glucose and xylose for H2 production have been isolated and investigated.6–8 However, current research on glucose and xylose co-fermentation for H2 production is mainly focused on batch cultivation. From an application perspective, continuous H2 production from both glucose and xylose is more commercially and practically suitable to realize highly efficient biological conversion of lignocellulosic biomass to H2.9 Compared to H2 production through batch tests, continuous H2 production can reduce human labour such as medium replacement.10 Moreover, owing to the real-time emission of soluble volatile acids produced during the fermentation process, the inhibitory effect on H2 producing bacteria can be significantly reduced; this results in high bacterial activity during the fermentation process.11,12 Currently, a continuous stirred tank reactor (CSTR) is widely used for H2 production; however, its performance is still limited by biomass loss, thus leading to low substrate consumption (especially xylose) again.13 To avoid metabolic by-product inhibition during batch fermentation as well as biomass loss during continuous H2 production by the CSTR, sequential batch fermentation carried out by an anaerobic sequential batch reactor (ASBR), a cultivation method between batch and continuous fermentation for H2 production, was proposed.14 This cultivation method decoupled the residence time of the H2 producing bacteria in the reactor and the hydraulic retention time, leading to the maintenance of a higher biomass density in the reactor. During the fermentation process, both the addition and discharge of the fermentation broth are carried out in a stage-wise manner. Consequently, substrate inhibition and metabolite repression can be effectively relieved, and this process is relatively simple and flexible to operate. Moreover, a higher biomass density can be held in the reactor, thus suggesting great potential for dual substrate H2 fermentation. However, current research on bio-H2 production using sequential batch fermentation is mainly focused on glucose alone,15 and no research is available regarding the quantitative analysis of substrates with xylose alone as well as glucose and xylose mixtures.
In this research, a previously isolated and characterized moderately thermophilic bacteria, Thermoanaerobacterium thermosaccharolyticum W16,16 was adopted to study the law of H2 production and substrate utilization by observing the sequential batch fermentation by an ASBR from glucose, xylose, as well as glucose and xylose mixtures. Moreover, the effect of hydraulic retention time (HRT) on the substrate utilization efficiency and H2 production performance at high glucose to xylose mass ratios was investigated. Finally, the energy conversion efficiencies obtained using various carbon sources and operational configurations were evaluated and compared. This is the first time that an ASBR has been employed for the evaluation of continuous H2 production from a glucose/xylose mixture with different mass ratios.
Material and methods
Inoculum
The H2 producing bacteria Thermoanaerobacterium thermosaccharolyticum W16 used in this study was isolated from hot springs and cultured on a liquid medium containing glucose 10 g L−1, NH4Cl 1.0 g L−1, K2HPO4 3.0 g L−1, KH2PO4 1.5 g L−1, MgCl2·6H2O 0.5 g L−1, NaCl 1.0 g L−1, KCl 0.2 g L−1, cysteine–HCl 0.5 g L−1, yeast extract 2.0 g L−1, and peptone 2.0 g L−1.16 The pH of the culture medium was adjusted to 7.0 ± 0.2. Anaerobic bottles (100 mL) containing 60 mL medium autoclaved at 121 °C for 15 min were adopted for bacterial growth. Cultures were then maintained at 60 °C in an orbital shaker with a rotation speed of 130 rpm, and cells at the mid-exponential growth phase would be used as inoculum to the reactors.
Reactor setup and operation
Sequential-batch H2 production was conducted in an 800 mL lab scale ASBR as described previously.17 Prior to inoculum, the reactors were filled with 500 mL medium and flushed with high purity nitrogen gas (99.99%) for 30 min to maintain anaerobic conditions; then, the sterilized reactor was seeded with 2% (v/v) inoculum of the total reactor working volume. The temperature of the reactors was kept constant at 60 °C, and liquid medium in the reactors was homogeneously mixed by a magnetic stirrer at 130 rpm. During the whole fermentation process, pH was maintained automatically within 6.5–7.2 using a 1 N NaOH solution. Reactors were operated in the batch mode for 12 h to accumulate biomass at first and then turned into sequential batch operation. After the steady state was obtained, the effects of different carbon sources and HRT on substrate consumption profile and H2 production performance were investigated sequentially.
Effect of carbon source on the performance of H2 production
To determine the effect of different carbon sources on the H2 production performance of sequential batch fermentation, the carbon source in the influent medium (as described in Inoculum section) was replaced with 10 g L−1 glucose (G), 8 g L−1 glucose and 2 g L−1 xylose (GX-4), 5 g L−1 glucose and 5 g L−1 xylose (GX-1), 2 g L−1 glucose and 8 g L−1 xylose (GX-1/4), and 10 g L−1 xylose (X). At this stage, the system HRT was kept constant at 8 h according to the fermentation characteristic of T. thermosaccharolyticum W16.16 The fill, react, settle, and decanting period were controlled by computer precisely at 15 min, 3 h, 30 min, and 15 min, respectively. The volume of the feed and decant was 250 mL, half working volume of the reactor. Under each condition, the reactor was operated for enough time until steady-state condition (based on constant volumetric H2 production rates ranging from 5 to 10% over 15 cycles) was achieved.
Effect of HRT on the performance of H2 production
To ensure a maximum H2 production yield at high glucose and xylose mass ratios, H2 production and substrate consumption profile were examined at different HRTs of 6, 12, 18, and 24 h, and the detailed fill, react, settle, and decanting times are shown in Table 1. All other operating conditions were the same as described above. At each HRT, the reactor was operated for enough time until steady-state conditions (based on constant volumetric H2 production rates ranging from 5 to 10% over 15 cycles) were obtained.
Table 1 Operation parameters of ASBR at different HRTs
Parameters |
HRT (h) |
6 |
12 |
18 |
24 |
Cycle period (h) |
3 |
6 |
9 |
12 |
Fill period (min) |
15 |
15 |
15 |
15 |
React period (h) |
2 |
5 |
8 |
11 |
Settle period (min) |
30 |
30 |
30 |
30 |
Decanting period (min) |
15 |
15 |
15 |
15 |
Fill & decanting volume (mL) |
250 |
250 |
250 |
250 |
Analytical methods
Liquid samples obtained from the reactors at certain time intervals were filtered through a 0.22 μm membrane before analysis. Glucose and xylose in the influent medium and effluent culture broth were detected by high-performance liquid chromatography (HPLC, LC-10A, Shimadzu Corporation, Kyoto, Japan) equipped with an Aminex HPX-87P column (Bio-Rad, Hercules, CA) at 80 °C with 5 mmol L−1 H2SO4 as the mobile phase at a flow rate of 0.4 mL min−1, as described by Zhao et al., 2017.18 The concentrations of the volatile fatty acids (VFAs) and alcohols, including ethanol and butanol, were analysed using a gas chromatograph (Agilent 7890 A, Agilent Technologies, USA) equipped with a flame ionization detector. The detailed operational parameters were the same as described by Zhao et al., 2014.19 The percentage of H2 in biogas was determined by a gas chromatograph (GCSC2, Shanghai Analytical Apparatus, Shanghai, China) equipped with a thermal conductivity detector and a 2.0 m stainless column packed with TDS-01 (60/80 mesh). The operational temperatures of the injection port, oven and detector were 110, 40 and 70 °C, respectively. N2 was used as the carrier gas at a flow rate of 70 mL min−1. By multiplying the total gas volume by the ratio of H2 content, the H2 production volume was determined. The cell dry weight (CDW) of T. thermosaccharolyticum W16 in the reactor was determined from the effluent using the steady state value of the optical densities at each HRT using the equation CDW (g L−1) = (0.258 × OD600) + 0.001.17
Data analysis
The H2 production rate (H) data were fitted to a modified Gompertz equation20 for describing the H2 production capacity in sequential batch experiment:
where Hm is the potential H2 production rate (mmol L−1 h−1), Rm is the maximum H2 production accelerated rate (mmol L−1 h−2), λ is the lag phase (d), t is the fermentation time (d), and e is 2.71828. Curve fitting and model parameters were solved by the software MATLAB 8.3.
Results and discussion
H2 production from a mixture of glucose/xylose by an ASBR
The H2 production obtained at various glucose and xylose compositions at an HRT of 8 h by an ASBR is shown in Fig. 1. The H2 production rate (HPR) values were influenced by the variations in the glucose and xylose composition. It could be noted that the ASBR operated with glucose alone had maximal production performance with a peak HPR of 7.86 mmol L−1 h−1. Considering the glucose and xylose mixture, the production trend has shown some fluctuations: maximal HPR achieved at GX-4, GX-1, and GX-1/4 are 7.75 mmol L−1 h−1, 7.12 mmol L−1 h−1, and 6.69 mmol L−1 h−1, respectively. The minimal HPR of 6.13 mmol L−1 h−1 was obtained with solely xylose as the substrate. During the whole fermentation process, high level glucose consumption between 82.5% and 93.5% was observed with glucose concentration in the substrate ranging from 10 g L−1 and 2 g L−1, as shown in Fig. 2. Compared to the glucose consumption profile, in the absence and at a low concentration of glucose (2 g L−1) in the substrate, the xylose consumption ratio can maintain a high level of 81.3% and 80.6%, which is significantly higher than that obtained in the continuous H2 production process.17 However, a further increase in the glucose concentration to 5 g L−1 reduced the xylose consumption to 66.6%. When the glucose concentration reached 8 g L−1, the xylose consumption dropped to 55.2%.
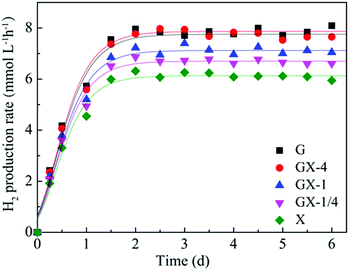 |
| Fig. 1 Profile of HPR from a mix of glucose/xylose by the ASBR. ((G) 10 g L−1 glucose; (GX-4) 8 g L−1 glucose and 2 g L−1 xylose; (GX-1) 5 g L−1 glucose and 5 g L−1 xylose; (GX-1/4) 2 g L−1 glucose and 8 g L−1 xylose; (X) 10 g L−1 xylose). | |
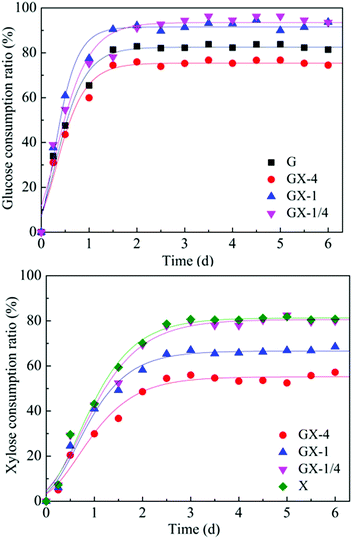 |
| Fig. 2 Profile of substrate consumption from a mix of glucose/xylose by ASBR. ((G) 10 g L−1 glucose; (GX-4) 8 g L−1 glucose and 2 g L−1 xylose; (GX-1) 5 g L−1 glucose and 5 g L−1 xylose; (GX-1/4) 2 g L−1 glucose and 8 g L−1 xylose; (X) 10 g L−1 xylose). | |
From the abovementioned results, it can be concluded that throughout the ASBR-operated H2 fermentation process with different mass ratios of glucose and xylose, the H2 production rate can be maintained at a high level of 7.1 ± 0.9 mmol L−1 h−1, and it fluctuates in a small range. Compared with the case of the batch test for H2 fermentation, the maximum HPR of 7.75 mmol L−1 h−1 from a mix of glucose and xylose (GX-4) was 13.1% lower, but this HPR was more stable. Moreover, compared with anaerobic continuous-flow stirred tank reactors (CSTRs) for continuous H2 fermentation, H2 production by ASBR could generate 8.3% more H2 with more complete substrate utilization.21 However, because of the cultivation characteristics of ASBR, during the reaction, the glucose consumption rate for T. thermosaccharolyticum W16 was always higher than that of xylose; thus, glucose was consumed rapidly. After a certain period of time, a large amount of fresh medium filled into the reactor will lead the bacteria to always keep a high glucose utilization state, whereas xylose utilization will be inhibited to some extent. Moreover, it was found that in both the CSTR and ASBR-operated H2 fermentation processes, the xylose consumption rate was always higher than that in batch fermentation. It is speculated that the end-products produced during fermentation still possess a negative impact on xylose utilization. Therefore, efforts need to be made on how to increase the xylose utilization rate in the presence of a large amount of glucose in the substrate and thus to realize highly efficient lignocellulose bioconversion to H2.
H2 production by the ASBR from a mixture containing high glucose to xylose ratios at different HRTs
HRT is considered to be a major factor influencing the continuous H2 production.1 Appropriate control of HRT will determine the reaction time between microorganisms and substrates as well as the bacterial density in the bioreactor.22 Depending on different bacterial growth characteristics and substrate types, HRTs ranging from 4 h to 24 h were usually required for dark fermentative H2 production.23 In this research, the reactor was operated at the HRT of 6 h, 12 h, 18 h, and 24 h according to the H2 producing characteristic of T. thermosaccharolyticum W16 (ref. 24 and 25) at a high glucose and xylose ratio (8 g L−1 glucose and 2 g L−1 xylose) to evaluate if xylose conversion efficiency could be improved by the HRT control strategy. The results are shown in Fig. 3; under steady state conditions, a maximum H2 production rate of 8.9 mmol L−1 h−1, equivalent to a cumulative H2 volume of 2450 mL L−1, is obtained at an HRT of 12 h. A decrease in the HRT to 6 h led to a decline in the H2 production rate to 6.8 mmol L−1 h−1. The H2 production rate further decreased from 5.7 to 3.8 mmol L−1 h−1 with an increase in the HRT from 18 h to 24 h. During continuous H2 fermentation process, the H2 production rate can represent the H2 producing ability of the reactor, and this value is subject to variation in the HRT. It has been stated in many studies that a decrease in HRT can enhance the production performances due to the availability of more substrate to the microorganisms.26 However, it will also result in incomplete substrate utilization and massive loss of biomass in the reactor.27 The biomass concentration of only 0.4 g-CDW L−1 (Table 2) in the reactor at an HRT 6 h further confirmed this point. At higher HRTs, although substrate within the ASBR stays longer and additional time can be provided for glucose and xylose to diffuse from the bulk medium to cells, the reduction of essential nutrients and the large amount of fermentation by-products accumulated in the reactor exert an inhibiting effect on the H2-producing bacteria. During the entire fermentation process, the maximum H2 content (61% v/v) was obtained at an HRT of 12 h, and only a small amount of CO2 was detected. Compared to the H2 production rate profile, the H2 yield remained constant with an increase in HRT from 6 h to 24 h. As an important parameter of H2 production process, H2 yield indicates whether carbohydrates in the reactor are favourable for H2 production or not. The constant H2 yield of the reactor suggested that the substrate consumption was in direct proportion to H2 generation. Moreover, the substrate utilization and product formation in the reactor were relatively stable. From the abovementioned results, it can be concluded that 12 h should be the optimum HRT for H2 production from a high glucose to xylose mass ratio of 4.
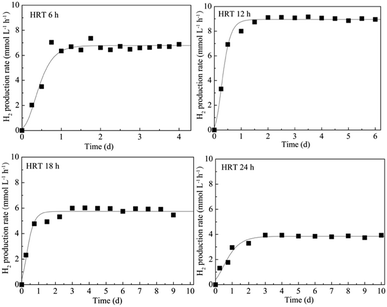 |
| Fig. 3 HPR from a mixture with high glucose to xylose ratios by the ASBR at different HRTs. | |
Table 2 Characteristics of H2 production from a mixture containing high glucose to xylose ratios at different HRTs by the ASBR
|
HRT (6 h) |
HRT (12 h) |
HRT (18 h) |
HRT (24 h) |
Acetic acid (mmol L−1) |
19.5 ± 0.2 |
23.1 ± 0.1 |
35.6 ± 0.7 |
50.3 ± 0.3 |
Butyric acid (mmol L−1) |
13.8 ± 0.1 |
15.1 ± 0.4 |
19.3 ± 0.5 |
24.5 ± 0.3 |
Ethanol (mmol L−1) |
6.3 ± 0.2 |
7.2 ± 0.2 |
10.6 ± 0.3 |
15.2 ± 0.3 |
Butanol (mmol L−1) |
0.6 ± 0.1 |
0.9 ± 0.09 |
1.2 ± 0.05 |
1.5 ± 0.04 |
Acetone (mmol L−1) |
0.3 ± 0.01 |
0.5 ± 0.02 |
0.6 ± 0.02 |
1.0 ± 0.01 |
pH |
6.1 ± 0.2 |
5.8 ± 0.1 |
5.5 ± 0.1 |
4.9 ± 0.1 |
H2 yield (mol H2 mol−1 substrate) |
1.5 ± 0.2 |
1.9 ± 0.2 |
1.8 ± 0.1 |
1.6 ± 0.1 |
Cell dry weight (g-CDW L−1) |
0.4 ± 0.09 |
0.7 ± 0.1 |
0.9 ± 0.1 |
1.0 ± 0.1 |
An ideal H2 fermentation process not only provides a high H2 production rate, but also realizes rational utilization of the substrate. Therefore, the glucose and xylose consumption profiles as a function of time at different HRTs were also monitored, and the results are shown in Fig. 4. At an HRT higher than 6 h, the glucose consumption ratios were over 90%, and the corresponding xylose utilization rate increased from 23.9% at an HRT of 6 h to 86.4% at an HRT of 24 h. This result further confirmed that glucose was more readily utilized by T. thermosaccharolyticum W16, which was consistent with the results obtained from the batch and continuous fermentation H2 production process. Throughout the ASBR fermentation process, in addition to a substantial decline in HRT, only a small amount of sugar can be detected in the effluent; this indicates that ASBR enables reasonable and effective use of both glucose and xylose for H2 production even at a high mass ratio. A similar trend was observed for biomass concentration in the reactor; the maximum values of 0.9 g-CDW L−1 and 1.0 g-CDW L−1 were obtained at the HRTs of 18 h and 24 h, respectively (Table 2). Although a higher HRT was more favourable for xylose fermentation in the mixed substrate, the H2 production rate did not increase further. Under all the operating conditions, acetic acid and butyric acid were the main fermentation end products, accompanied by a small amount of ethanol, butanol, and acetone. With an increase in HRT and the accumulation of metabolic end products, the corresponding pH decreased from 6.1 to 4.9. Considering both H2 production efficiency and substrate consumption of ASBR operated at high glucose to xylose mass ratios at different HRTs, the H2 production capacity reached maximum at an HRT of 12 h. Moreover, under this condition, the glucose and xylose consumption ratio reached 92.2% and 82.2%, respectively. Therefore, it could be concluded that continuous H2 production from a mixture containing a high glucose to xylose mass ratio through ASBR by HRT control was an effective strategy to elevate both the substrate consumption and H2 production rate.
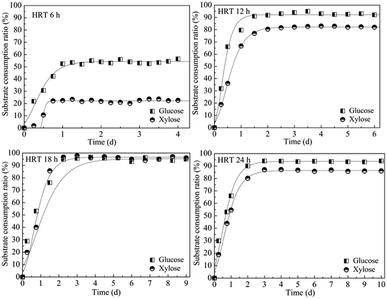 |
| Fig. 4 Substrate consumption at high glucose to xylose ratios by the ASBR at different HRTs. | |
Energy conversion analysis of H2 production from glucose/xylose
To further evaluate the overall H2 production performance of ASBR, energy conversion efficiency (ECE) was analysed in this research. By comparing the total specific energy of H2 produced by the total specific energy of glucose and xylose consumed by the bacteria,28 the energy conversion efficiency (ECE) was calculated by the equation shown below:29
The higher heating values (HV) of H2, glucose and xylose were 142 kJ g−1, 15.6 kJ g−1, and 16.72 kJ g−1, respectively.30
With respect to ECE, it is difficult to compare the results obtained herein with others described in the literature. Since the ECE value is subject to the type and composition of substrate and the aim of this study was to provide an operational strategy for highly efficient lignocellulosic biomass conversion to H2, the results obtained by the ASBR were only compared with those obtained by CSTR, which was carried out under the same conditions previously.21
The amount of energy transformed and the energy conversion efficiency obtained from CSTR and ASBR at different glucose to xylose mass ratios are shown in Table 3. It can be clarified that ECEs obtained by the ASBR from solely glucose and a mixture of glucose and xylose with the mass ratios of 4
:
1 and 1
:
1 as substrates exhibit higher energy conversion efficiency, and 50 ± 6% of theoretical value has been achieved; a similar value of the energy conversion efficiency has been obtained from a mixture of glucose and xylose with a mass ratio of 1
:
4 and xylose alone as substrates in both CSTR and ASBR. For either CSTR or ASBR operated in this research, the low xylose consumption and H2 production under high glucose to xylose mass ratio condition can be solved by the HRT control strategy. At the HRT of 12 h in ASBR, the maximum ECE of 21.5% obtained was 71.6% of the theoretical value, which was 5.8% higher than that obtained at 8 h and even 4.6% higher than the highest value obtained by CSTR operated at a 10 h HRT.
Table 3 Energy conversion efficiencies of H2 production from glucose/xylose under different operational configurations
Reactors |
HRT |
Substrate |
H2 yield (mL H2/g substrate) |
HV of H2 (kJ) |
ECE (%) |
Theoretical ECE (%) |
Ref. |
CSTR |
8 |
G |
164.4 |
2.1 |
13.3 |
30.2 |
Zhao et al., 2013 (ref. 18) |
8 |
GX-4 |
179.9 |
2.3 |
14.5 |
30.0 |
8 |
GX-1 |
149.7 |
1.9 |
12.1 |
29.9 |
8 |
GX-1/4 |
146.2 |
1.9 |
11.8 |
29.7 |
8 |
X |
135.5 |
1.7 |
10.9 |
29.6 |
10 |
GX-4 |
209.6 |
2.7 |
16.9 |
30.0 |
ASBR |
8 |
G |
170.8 |
2.2 |
13.8 |
30.2 |
This study |
8 |
GX-4 |
194.8 |
2.5 |
15.7 |
30.0 |
8 |
GX-1 |
161.4 |
2.1 |
13.0 |
29.9 |
8 |
GX-1/4 |
144.3 |
1.8 |
11.7 |
29.7 |
8 |
X |
135.2 |
1.7 |
10.9 |
29.6 |
12 |
GX-4 |
266.5 |
3.4 |
21.5 |
30.0 |
From the results obtained, fermentation of a mix of glucose and xylose irrespective of ratio by ASBR combined with HRT control strategy is an effective way to increase the energy conversion efficiency. To date, conventional H2 production from fossil fuel is recognized as the cheapest H2 production process, and an energy conversion efficiency less than 10% as compared to that obtained in this process is regarded as non-competitive.31 Nowadays, H2 production from renewable resources is a hot topic, e.g. the maximum solar to H2 energy conversion efficiency is only 1.1%.32 In this research, the energy conversion efficiency was increased to 21.5% by adopting the ASBR system by HRT control, suggesting an attractive biological H2 production process from lignocellulose. To the best of our knowledge, this is the first report in which the energy conversion efficiency of the proposed system using different glucose to xylose ratios has been investigated. Compared with CSTR, the ASBR system produced more H2 with more complete substrate consumption and higher energy conversion efficiency and thus could be considered as an efficient and promising H2 fermentation process.
The aforementioned results clearly indicated the feasibility of using ASBR for continuous H2 production from lignocellulose hydrolysate regardless of the pretreatment and saccharification method; for industrial application, scaled-up and long-term studies using real lignocellulosic hydrolysate as an influent are essential to further investigate the stability and productivity of H2 through the ASBR system. In addition, to further enhance the energy conversion efficiency, future studies can be conducted to convert fermentation metabolic products (mainly volatile fatty acids) to H2 or CH4 by subsequent photo fermentation or anaerobic digestion to make this system more available for practical application.
Conclusions
In this study, a mixture of glucose and xylose at different ratios was used as a substrate for bio-H2 production by the ASBR. The results showed that glucose and xylose consumptions were above 80% and 50%, respectively, with a high HPR of 7.11 ± 0.9 mmol L−1 h−1. HRT had a profound impact on the performance of H2 production at high glucose to xylose ratios. The maximum H2 production rate of 8.9 mmol L−1 h−1 was obtained from the ASBR at the optimal HRT of 12 h with glucose and xylose consumption of 92.2% and 82.2%, respectively, which was 36.8% higher than that obtained by ASBR operated at an 8 h HRT. By retaining 1.9 g L−1 biomass in the reactor, a maximum energy conversion efficiency of 21.5% could be achieved. These results showed great potential and viability of the ASBR system for application in biological lignocellulose hydrolysate conversion to H2.
Conflicts of interest
There are no conflicts to declare.
Acknowledgements
This research was supported by the Open Project of State Key Laboratory of Urban Water Resource and Environment, the Harbin Institute of Technology (No. ES201810-01) and the Fundamental Research Funds for the Central Universities (Grant No. HIT. NSRIF. 201859), China. Lei Zhao acknowledges the UQ Fellowship (2016000091) and the UQ Early Career Research Grant (2017003152) from The University of Queensland, Australia.
References
- S. G. Won and A. K. Lau, Bioresour. Technol., 2011, 102, 6876–6883 CrossRef PubMed.
- I. Valdez-Vazquez and A. Sanchez, Biofuels, Bioprod. Biorefin., 2018, 12, 56–67 CrossRef.
- H. H. P. Fang and H. Liu, Bioresour. Technol., 2002, 82, 87–93 CrossRef PubMed.
- C. H. Hung, K. S. Lee, L. H. Cheng, Y. H. Huang, P. J. Lin and J. S. Chang, Appl. Microbiol. Biotechnol., 2007, 75, 693–701 CrossRef PubMed.
- S. R. Kim, S. J. Ha, N. Wei, E. J. Oh and Y. S. Jin, Trends Biotechnol., 2012, 30, 274–282 CrossRef PubMed.
- H. S. Jayasinghearachchi, P. M. Sarma and B. Lal, Int. J. Hydrogen Energy, 2012, 37, 5569–5578 CrossRef.
- S. Subudhi, T. Nayak, N. R. Kumar, P. Vijayananth and B. Lal, Int. J. Hydrogen Energy, 2013, 38, 2728–2737 CrossRef.
- D. An, Q. Li, X. Q. Wang, H. H. Yang and L. J. Guo, Int. J. Hydrogen Energy, 2014, 39, 19928–19936 CrossRef.
- G. C. Zhang, J. J. Liu, I. I. Kong, S. Kwak and Y. S. Jin, Curr. Opin. Chem. Biol., 2015, 29, 49–57 CrossRef PubMed.
- C. F. Crespo, M. Badshah, M. T. Alvarez and B. Mattiasson, Bioresour. Technol., 2012, 103, 186–191 CrossRef PubMed.
- S. Brethauer and C. E. Wyman, Bioresour. Technol., 2010, 101, 4862–4874 CrossRef PubMed.
- O. Mizuno, R. Dinsdale, F. R. Hawkes, D. L. Hawkes and T. Noike, Bioresour. Technol., 2000, 73, 59–65 CrossRef.
- C. Y. Lin, C. C. Wu and C. H. Hung, Int. J. Hydrogen Energy, 2008, 33, 43–50 CrossRef.
- S. R. Chaganti, B. Pendyala, J. A. Lalman, S. S. Veeraoalli and D. D. Heath, Int. J. Hydrogen Energy, 2013, 38, 2212–2220 CrossRef.
- L. Shizas and D. M. Bagley, Water Res., 2002, 36, 363–367 CrossRef PubMed.
- N. Q. Ren, G. L. Cao, A. J. Wang, D. J. Lee, W. Q. Guo and Y. H. Zhu, Int. J. Hydrogen Energy, 2008, 33, 6124–6132 CrossRef.
- L. Zhao, G. L. Cao, A. J. Wang, H. Y. Ren and N. Q. Ren, Int. J. Hydrogen Energy, 2014, 39, 19311–19316 CrossRef.
- L. Zhao, G. L. Cao, T. Sheng, H. Y. Ren, A. J. Wang, J. Zhang, Y. J. Zhong and N. Q. Ren, Bioresour. Technol., 2017, 243, 548–555 CrossRef PubMed.
- L. Zhao, G. L. Cao, A. J. Wang, H. Y. Ren, K. Zhang and N. Q. Ren, Biotechnol. Biofuels, 2014, 7, 178 CrossRef PubMed.
- J. J. Lay, Y. J. Lee and T. Noike, Water Res., 1999, 33, 2579–2586 CrossRef.
- L. Zhao, G. L. Cao, A. J. Wang, H. Y. Ren and N. Q. Ren, Int. J. Hydrogen Energy, 2013, 38, 15100–15104 CrossRef.
- M. A. R. Aguilar, L. A. Fdez-Guelfo, C. J. Alvarez-Gallego and L. I. R. Garcia, Chem. Eng. J., 2013, 219, 443–449 CrossRef.
- H. S. Shin, J. H. Youn and S. H. Kim, Int. J. Hydrogen Energy, 2004, 29, 1355–1363 CrossRef.
- L. Zhao, G. L. Cao, A. J. Wang, H. Y. Ren, C. J. Xu and N. Q. Ren, GCB Bioenergy, 2013, 5, 591–598 CrossRef.
- L. Zhao, G. L. Cao, A. J. Wang, W. Q. Guo, H. Y. Ren and N. Q. Ren, Bioresour. Technol., 2013, 145, 103–107 CrossRef PubMed.
- G. Kumar, J. H. Park, M. S. Kim, D. H. Kim and S. H. Kim, Int. J. Hydrogen Energy, 2014, 39, 20625–20631 CrossRef.
- F. Y. Chang and C. Y. Lin, Int. J. Hydrogen Energy, 2004, 29, 33–39 CrossRef.
- V. M. Corona and E. Razo-Flores, Bioresour. Technol., 2018, 249, 334–341 CrossRef PubMed.
- H. Y. Ren, F. Y. Kong, J. Ma, L. Zhao, G. J. Xie, D. F. Xing, W. Q. Guo, B. F. Liu and N. Q. Ren, Bioresour. Technol., 2018, 252, 110–117 CrossRef PubMed.
- A. Xia, J. Cheng, L. K. Ding, R. C. Lin, W. L. Song, H. B. Su, J. H. Zhou and K. F. Cen, Appl. Energy, 2015, 139, 9–16 CrossRef.
- J. O. Bockris, Int. J. Hydrogen Energy, 1981, 6, 223–241 CrossRef.
- Q. Wang, T. Hisatomi, Q. X. Jia, H. Tokudome, M. Zhong, C. Z. Wang, Z. H. Pan, T. Takata, M. Nakabayashi, N. Shibata, Y. B. Li, I. D. Sharp, A. Kudo, T. Yamada and K. Domen, Nat. Mater., 2016, 15, 611–615 CrossRef PubMed.
|
This journal is © The Royal Society of Chemistry 2018 |