DOI:
10.1039/C8RA02546K
(Paper)
RSC Adv., 2018,
8, 15480-15486
A family of multi-spin rare-earth complexes based on a triazole nitronyl nitroxide radical: synthesis, structure and magnetic properties†
Received
23rd March 2018
, Accepted 12th April 2018
First published on 24th April 2018
Abstract
The combination of LnIII ions (GdIII, TbIII or DyIII) and a triazole nitronyl nitroxide radical (4-Me-3-NITtrz) as spin carriers results in two mononuclear and one binuclear compounds, namely, [Ln(hfac)3(4-Me-3-NITtrz)(H2O)] (Ln = Gd(1), Tb(2); hfac = hexafluoroacetylacetone; 4-Me-3-NITtrz = 2-[3-(4-methyl-l,2,4-triazolyl)]-4,4,5,5-tetramethylimidazoline-1-oxyl-3-oxide) and [Dy(hfac)2(4-Me-3-NITtrz)2][Dy(hfac)4]·CHCl3. Compounds 1 and 2 are isostructural and crystallize in the P
space group, whereas compound 3 crystallizes in the P21/n space group. In 1 and 2, the central LnIII ions are nine-coordinated (LnNO8) in a distorted spherical capped square antiprism geometry (C4v) finished by three bischelate hfac anions and one bidentate triazole radical and one aqua molecule. While 3 is a mixed-coordinated binuclear compound with Dy1 in a triangular dodecahedron (D2d) coordination sphere and Dy2 in a biaugmented trigonal prism (C2v) coordination sphere. Magnetic studies show that compound 2 exhibits field-induced single-molecule magnet (SMM) behavior.
Introduction
Low-dimensional molecular assemblies based on anisotropic metal ions that show slow relaxation of magnetization have attracted much attention.1,2 Such materials, named single-molecule magnets (SMMs) and single-chain magnets (SCMs), have latent applications in high-density data storage materials,3 quantum computations,4 and spintronic devices.5 For SMMs, reversal of magnetization arises from the combination of a large ground-state spin S and an Ising-type anisotropy. Recent studies show that rare-earth elements, especially heavy lanthanide ions such as terbium(III) and dysprosium(III), bearing huge magnetic moments and large intrinsic magnetic anisotropy, have become good candidates for the construction of SMMs.6 This has obviously increased the thermal energy barriers for the reversal of magnetization.6a,7–11 For example, the highest relaxation energy barrier 1837 K (twenty times higher than that in Mn6 (ref. 12)) was observed in a DyIII SMM.11 However, the naturally accompanying quantum tunneling effect (QTM) resulting from the hyperfine couplings and dipolar spin–spin interactions of 4f ions always lowers the relaxation energy barrier and induces the loss of remnant magnetization,13 which is the inherent drawback for 4f-SMMs which can be observed even at liquid helium temperature. Among the various chemical routes investigated to obtain and improve the characteristics of 4f-SMMs, a strategy involving lanthanide ions with organic radicals has proved very successful.13–16 Rare-earth-radical based SMMs are appealing candidates owing to the Ising anisotropy of their metallic centers and the strong magnetic interactions between the radical and the metal ion, which may effectively quench the QTM and prevent the loss of magnetization. In particular, the N23− radical-bridged TbIII complex exhibits hysteresis with a record blocking temperature up to 20 K.16d
Triazole ligands play a crucial role in the field of spin transition, and nitronyl nitroxide radicals are important in the fields of molecular magnets and nonlinear optics. Up to now, only four triazole nitronyl nitroxide radicals have been reported.17 All of the free radicals exhibit ferromagnetic interactions at low temperature, which proves that the π system and the N atoms in the triazole ring are good units for transferring effective magnetic interactions. Now we are interested in a nitronyl nitroxide radical based on a triazole ring, named 2-[3-(4-methyl-l,2,4-triazolyl)]-4,4,5,5-tetramethylimidazoline-1-oxyl-3-oxide (4-Me-3-NITtrz) (Scheme 1). So far, no rare-earth complexes with this radical have been reported. Herein we synthesize three lanthanide compounds based on radical 4-Me-3-NITtrz, namely, [Ln(hfac)3(4-Me-3-NITtrz)(H2O)] (Ln = Gd(1) and Tb(2)) and [Dy2(hfac)6(H2O)2]·1/2CH2Cl2 (3). Magnetic studies showed that complex 2 exhibits temperature-dependent ac susceptibility at low temperature, which suggests SMM behavior.
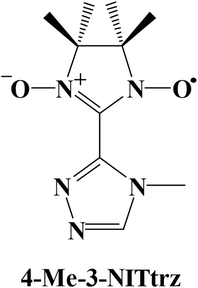 |
| Scheme 1 The structure of 4-Me-3-NITtrz. | |
Experimental details
Materials and physical measurements
All of the reagents used in the syntheses were of analytical grade, except n-heptane and dichloromethane which were distilled after drying with Na and CaH2, respectively. Ln(hfac)3·2H2O was synthesized according to the method in the literature.14d The radical 4-Me-3-NITtrz was prepared based on the procedure in the literature.17a Elemental analyses for carbon, hydrogen, and nitrogen were performed on a PerkinElmer 240 elemental analyzer. Infrared spectra were recorded from KBr pellets in the 4000–400 cm−1 region on a Bruker TENOR 27 spectrometer. Powder X-ray diffraction measurements were recorded on a D/Max-2500 X-ray diffractometer using Cu-Kα radiation. Direct-current (dc) magnetic susceptibilities of crystalline samples were measured on an MPMS-7 SQUID magnetometer in the temperature range of 2–300 K with a 1000 Oe applied magnetic field. Diamagnetic corrections were made with Pascal's constants for all the constituent atoms and sample holders. Alternating-current (ac) susceptibilities were performed on the same magnetometer under a 0 or 3000 Oe dc field with an oscillation of 3.5 Oe.
X-ray crystallography
Diffraction intensities were collected by using the φ–ω scan technique at 113 (for 1 and 2) or 157 K (for 3) on an Agilent SuperNova (Dual, Cu at zero, AtlasS2, CCD) diffractometer equipped with mirror-monochromated Cu-Kα radiation (λ = 1.54184 Å). Semiempirical multiscan absorption corrections were applied by SCALE3 ABSPACK, and the program CrysAlisPro18 was used for integration of the diffraction profiles. The structures were solved by direct methods and refined with the full-matrix least-squares technique using the ShelXT and ShelXL programs.19 Anisotropic thermal parameters were assigned to all non-H atoms. Some restraints, such as ISOR, DFIX, EADP, SADI, were applied to restrain the fluorine atoms and carbon atoms so as to avoid ADP problems on them. The organic hydrogen atoms were geometrically generated. H atoms attached to water molecules were located from difference maps and refined with isotropic temperature factors. Crystallographic data for the two compounds are listed in Table 1. Selected bond lengths of 1–3 are listed in Tables S1–S3.† CCDC 1831067, 1831068, and 1831069 contain the supplementary crystallographic data for compounds 1–3, respectively.
Table 1 Crystallographic data and structure refinement details for 1–3
|
1 |
2 |
3 |
Formula |
C25H21F18GdN5O9 |
C25H21F18N5O9Tb |
C51H39Cl3Dy2F36N10O16 |
Mr |
1034.72 |
1036.39 |
2163.27 |
Crystal system |
Triclinic |
Triclinic |
Monoclinic |
Space group |
P![[1 with combining macron]](https://www.rsc.org/images/entities/char_0031_0304.gif) |
P![[1 with combining macron]](https://www.rsc.org/images/entities/char_0031_0304.gif) |
P21/n |
a (Å) |
10.0094(3) |
9.9958(3) |
17.4350(4) |
b (Å) |
12.3115(4) |
12.3076(4) |
21.3325(7) |
c (Å) |
16.2016(5) |
16.1878(6) |
23.0646(5) |
α (°) |
105.182(3) |
105.052(3) |
90 |
β (°) |
103.964(3) |
103.900(3) |
97.183(2) |
γ (°) |
99.368(3) |
99.476(3) |
90 |
V (Å3) |
1814.66(10) |
1811.3(2) |
8511.2(4) |
Z |
2 |
2 |
4 |
ρcalc (Mg m−3) |
1.894 |
1.900 |
1.688 |
μ (mm−1) |
1.975 |
10.990 |
11.432 |
F (000) |
1008 |
1010 |
4200 |
θ range(°) |
3.364–25.09 |
3.833–67.07 |
3.649–74.261 |
GOF on F2 |
1.030 |
1.042 |
1.060 |
R1/wR2 [I > 2σ(I)] |
0.0394, 0.0868 |
0.0470, 0.1160 |
0.0739, 0.1933 |
R1/wR2 (all data) |
0.0462, 0.0913 |
0.0522, 0.1205 |
0.0897, 0.2060 |
Preparation of complexes of 1–3
Complexes 1 and 2 were obtained by the same procedure. A solution of Ln(hfac)3·2H2O (0.1 mmol) in dry heptane (15 mL) remained refluxing for 3 h and then cooled down to 60 °C, to which 4-Me-3-NITtrz (24 mg, 0.1 mmol) in CH2Cl2 (5 mL) was added. The resulting solution was stirred with refluxing for 1 h and then cooled to room temperature. After filtration, the final solution was stored in a refrigerator at 0–4 °C for about ten days to give blue-violet crystals, which were suitable for X-ray analysis.
[Gd(hfac)3(4-Me-3-NITtrz)(H2O)] (1). Yield 0.050 g, 48%. Elemental analysis calcd (%) for 1 (C25H21F18GdN5O9: 1034.72): C, 29.02; H, 2.04; N, 6.77. Found: C, 28.74; H, 1.98; N, 6.61.
[Tb(hfac)3(4-Me-3-NITtrz)(H2O)] (2). Yield 0.054 g, 52%. Elemental analysis calcd (%) for 2 (C25H21F18N5O9Tb: 1036.39): C, 28.97; H, 2.04; N, 6.76. Found: C, 28.68; H, 2.14; N, 6.53.Complex 3 was obtained by the following method. A suspension of 0.1 mmol Dy(hfac)3·2H2O in dry heptane (10 mL) remained refluxing for 1 h and then cooled to 75 °C, to which 4-Me-3-NITtrz (24 mg, 0.1 mmol) in CHCl3 (5 mL) was added. The mixture was stirred with refluxing for 0.5 h and then cooled to room temperature. After filtration, slow evaporation of the final solution for about two weeks in a refrigerator at 0–4 °C gave violet strip crystals suitable for single-crystal X-ray analysis.
[Dy(hfac)2(4-Me-3-NITtrz)2][Dy(hfac)4]·CHCl3 (3). Yield 0.032 g, 23%. Elemental analysis calcd (%) for 3 (C51H39Cl3Dy2F36N10O16: 2163.27): C, 28.31; H, 1.82; N, 6.48. Found: C, 28.22; H, 1.92; N, 6.26.
Results and discussion
Crystal structure
Structure of 1 and 2. Single-crystal X-ray diffraction indicates that complexes 1 and 2 are isomorphous and belong to the triclinic P
space group with Z = 2. Complex 2 is regarded as representative to describe the crystal structure. In 2, the asymmetric unit contains one crystallographically independent molecule with the central terbium ion in the TbNO8 coordination sphere. As shown in Fig. 1, Tb1 is surrounded by three bischelate hfac anions, one 4-Me-3-NITtrz radical ligand and one aqua molecule with a slightly distorted capped square antiprism (C4v) polyhedron configuration. Each MeTrzNIT acts as a bidentate chelate ligand and is coordinated to the same TbIII ions through one NO group and one nitrogen atom of the triazole ring. The Tb–O bond length associated with the NO group is 2.357(2) Å with the corresponding Tb–O–N angle of 130.3(3)°. The Tb–N distance (2.698(4) Å) is a little longer than normal Tb–N bonds. Other selected bond lengths and angles are listed in the ESI (Table S2†). When applying the C4v symmetry to the TbNO8 site, the CSM method gives the minimal derivation value from the ideal model with S = 0.394. The neighboring molecules are connected by weak hydrogen-bonding C–H⋯F interactions into a 3D supermolecular network with the shortest O–O (NO) contact of 9.996 Å (O2⋯O2#1) (Fig. S3 and S4, ESI†).
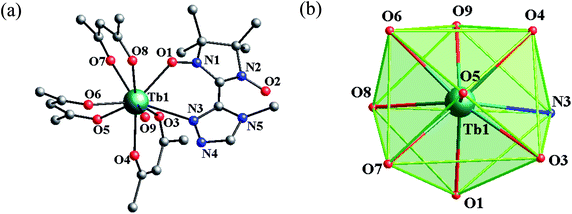 |
| Fig. 1 (a) Simplified view of the crystal structures of 2. Fluorine, hydrogen, and some carbon atoms are omitted for clarity. (b) Polyhedral configuration of the terbium atom in complex 2. | |
Structure of 3. Compound 3 belongs to the monoclinic P21/n space group and consists of one mononuclear [Dy(hfac)2(4-Me-3-NITtrz)2] anion, one [Dy(hfac)4] counter cation and a free chloroform. The asymmetric unit and the polyhedral of the DyIII ions are shown in Fig. 2. Dy1 is in the DyN2O6 coordinating environment completed by four oxygen atoms from two bischelate hfac, one two bidentate 4-Me-3-NITtrz radical with the NO group and a triazole nitrogen atom. Dy2 is surrounded by eight oxygen atoms from four hfac anions. The Dy–O(hfac) distances range from 2.304(5) to 2.400(5) Å, and the two Dy–Orad bond lengths are 2.307(5) and 2.311(5) Å, respectively. Dy1 is estimated as a triangular dodecahedron (D2d) coordination sphere with the deviation parameter S = 1.223, while Dy2 is in a biaugmented trigonal prism (C2v) coordination sphere with S = 0.932.
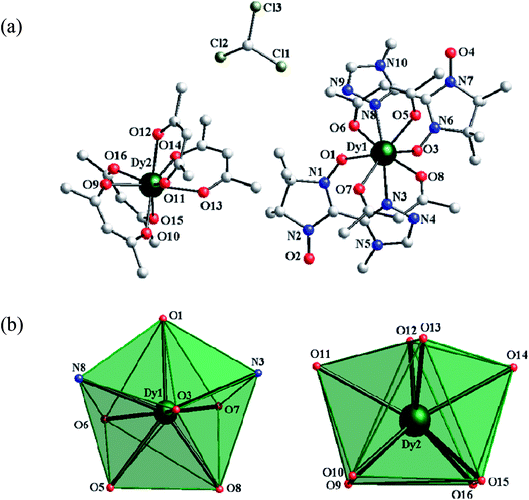 |
| Fig. 2 (a) The asymmetric coordination unit in complex 3. Fluorine, hydrogen, and some carbon atoms are omitted for clarity. (b) Polyhedral configurations of Dy1 and Dy2 in complex 3. | |
Static magnetic properties. The temperature-dependent magnetic susceptibilities of complexes 1–3 were performed under 1 kOe in the temperature range 2–300 K. The phase purity of the bulk samples was confirmed by XRD analyses and the experimental and simulated curves are shown in Fig. S5 and S6 (ESI).† The magnetic behaviors of the three complexes were studied and are shown in Fig. 3.
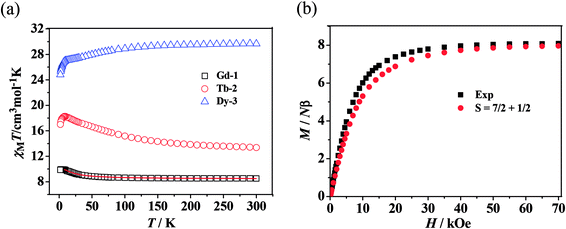 |
| Fig. 3 (a) χMT vs. T plots for 1–3 where the solid lines represent the theoretical values based on the corresponding equations. (b) Field dependence of the magnetization at 2 K for complex 1. | |
For 1, the value of χMT at 300 K is 8.52 cm3 K mol−1, a little higher than the theoretical value of 8.15 cm3 K mol−1 for an uncoupled GdIII ion (8S7/2, g = 2) plus an organic radical (S = 1/2, g = 2). Following a decrease in temperature, the value of χMT increases slightly up to 55 K, and then shows an abrupt increase to 9.94 cm3 K mol−1 at 2.5 K. The overall magnetic behavior indicates the presence of ferromagnetic coupling between the GdIII ion and the nitroxide radical.
The system was regarded as a bi-spin unit, and the magnetic simulation was carried out by using the spin Hamiltonian: H = −2JGd–RadŜGdŜRad, in which JGd–Rad represented the exchange coupling of the GdIII–radical. The mean-field approximation (zj′) was introduced to indicate the possible intermolecular interactions. The magnetic data were analyzed with the following equations.
|
 | (1) |
|
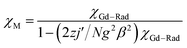 | (2) |
The experimental curve is better reproduced (Fig. 3) by the approximate eqn (1) and (2), giving fitting parameters of g = 2.02, JRad–Gd = 4.35 cm−1, and zj′ = −0.006 cm−1. The positive value of JGd–Rad reveals the presence of ferromagnetic interaction between Gd(III) and the radical, which is very common in Gd(III)–radical systems.1a,20 This ferromagnetic interaction is also corrected by the magnetization measurement at 2 K, where the M(H) curve remains above the Brillouin curve for an isolated spin of S = 1/2 + 7/2 (Fig. 3(b)). A magnetization of 8.01 Nβ is reached at 50 kOe, in agreement with the 7.98 Nβ for the ferromagnetic arrangement of the bi-spins.
As shown in Fig. 3, the observed room-temperature χMT value for complex 2 is 12.31 cm3 K mol−1, which is very close to the expected value of 12.10 cm3 K mol−1 for one free Tb(III) ion and one radical. Upon cooling, the χMT value continuously increased until it reached a maximum of 18.22 cm3 K mol−1 at 9 K, and then decreased to 16.97 cm3 K mol−1 at 2.0 K. The overall behavior indicates that there exists a ferromagnetic interaction between the Tb(III) ion and the radical, which is observed in other Tb–radical compounds.20b,21 The decrease in χMT below 9 K may be attributed to intermolecular magnetic coupling. For 3, the room temperature value of χMT is 29.66 cm3 K mol−1, a little higher than the theoretical value of 29.09 cm3 K mol−1 for two free DyIII ions plus two isolated radicals. As the temperature is lowered, the χMT value decreases slightly until 10 K, when it begins to decrease quickly as the temperature is lowered further and reaches the lowest value of 24.82 cm3 K mol−1 at 2 K. In the high-temperature range, the decrease in χMT value is ascribed to the depopulation of the LnIII stark sublevels and/or the Ln–radical interactions. In the low-temperature range, the decrease in χMT value can be attributed to the antiferromagnetic LnIII–radical interaction.
For both the complexes, the field-dependent magnetization value shows a rapid increase at low fields (Fig. S7 and 8, ESI†). For 2, M increases up to 7.17 Nβ at 70 kOe, which is much lower than the saturation value of 10 Nβ (9 Nβ for each TbIII ion for J = 6 and g = 3/2, plus 1 Nβ for one organic radical) (Fig. S7, see ESI†). For 3, the magnetization increases up to 15.09 Nβ at 70 kOe with an increase in the applied field, which also does not reach the expected saturation value of 22 N (Fig. S8, see ESI†). Taking into account the strong spin–orbit coupling in LnIII ions, the large gaps between experimental data and theoretical saturation values for compounds 2 and 3 can be attributed to the magnetic anisotropy and/or low-lying excited states in the systems.22
Dynamic magnetic properties. In order to examine the spin dynamics of compounds 2 and 3, alternating current (ac) measurements were carried out under a zero dc field or 3000 Oe with an oscillation of 3.5 Oe. For the mononuclear complex 2, temperature-dependent ac signals are observed in both in-phase and out-of-phase components with no peaks observed, which suggests that a very fast magnetization relaxation process may exist (Fig. S9, see ESI†). It is generally known that magnetization can reverse via a quantum mechanical tunneling process in lanthanide SMMs within the lowest energy doublet. However, the application of a static magnetic field can suppress QTM significantly. Here a dc field of 3000 Oe is used to probe the dynamic behavior of ac magnetic susceptibility (Fig. 4). Accordingly, well-resolved peaks emerge, which mean that the relaxation process is slowed by the external field. The relaxation time τ is extracted from the maximums of the imaginary component of the ac susceptibility based on the temperature-dependent data. The plot of ln
τ versus T−1 displays linear dependence, indicating that the relaxation follows a thermally activated Orbach mechanism (Fig. 5(a)). An effective energy gap of 16 K and a pre-exponential τ0 of 6.7 × 10−8 s were simulated from the Arrhenius law (τ = τ0
exp(Δeff/kBT)), which fall within the range of SMMs.13–16 As shown in Fig. 5(b), Cole–Cole plots exhibit nearly semicircular shapes. The generalized Debye model is used to extract the distribution parameters α and gives values of 0.32 (2 K) and 0.23 (2.6 K), indicating a single relaxation process. For complex 3, no apparent out-of-phase signals are observed above 2 K, which indicates that no slow relaxation exists (Fig. S11, see ESI†).
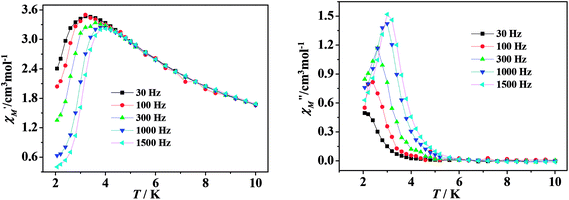 |
| Fig. 4 Temperature dependence of the real (left) and imaginary (right) components of ac magnetic susceptibility for 2 under a 3000 Oe dc field. | |
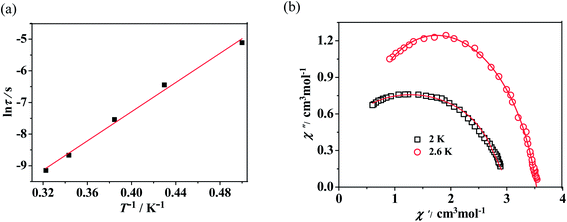 |
| Fig. 5 (a) Magnetization relaxation time, ln τ vs T−1 plot for 2 under 3000 Oe dc field. The red line is the fitting result based on the Arrhenius law. (b) Cole–Cole plots measured at 2 K and 2.6 K under 3000 Oe for 2; the solid lines are the best fit to the experimental data by using the Debye model. | |
For the lanthanide-containing complexes, the crystal field created by the surrounding ligands dramatically influenced the local anisotropy of the LnIII ions, which is crucial to the slow relaxation of magnetization in SMMs. As in this work, the TbIII compound exhibits field-induced SMM behavior, while the DyIII compound does not show any obvious signals in the out-of-phase ac magnetic susceptibility. Considering the Kramers nature of DyIII ions, the double degeneracy of the ground state, ±mJ, is always ensured in a zero applied dc field.23 The absence of slow relaxation of magnetization might result mostly from the small energy gap between the ground state and the first excited state in a certain local symmetry. Whereas for the non-Kramers TbIII ion, the spin-coupled ground state J = 6 can be split by the axial symmetry crystal field, leading to the lowest substate of mJ = ±6 having a large gap (more than 400 cm−1) from the second-lowest substate. However, the low axial symmetry of C4v geometry may remove the double degeneracy to some extent and reduce the energy barrier required for spin reversal. In addition, the quantum tunneling inducted by a transverse field and/or dipole–dipole interactions also decreases the final Ueff observed.
Conclusions
In conclusion, a triazole nitronyl nitroxide radical (4-Me-3-NITtrz) and three new compounds [Ln(hfac)3(4-Me-3-NITtrz)(H2O)] (Ln = Gd (1), Tb(2)) and [Dy(hfac)2(4-Me-3-NITtrz)2][Dy(hfac)4]·CHCl3 have been synthesized. For complex 1, the fitted results from the magnetic susceptibility reveal the presence of ferromagnetic interactions between Gd(III) and the radical, with JRad–Gd = 4.35 cm−1. The TbIII compound exhibits field-induced SMM behavior with a Ueff of 16 K and τ0 of 6.7 × 10−8 s under a 3000 Oe external field, while the DyIII compound shows no obvious out-of-phase ac signal. The results demonstrated that the local symmetry of central lanthanide ions is an important factor for magnetic anisotropy and for the rational design of new lanthanide–radical SMMs.
Conflicts of interest
There are no conflicts to declare.
Acknowledgements
This work was financially supported by the National Natural Science Foundation of China (21371133).
References
-
(a) C. Benelli, A. Caneschi, D. Gatteschi, L. Pardi, P. Rey, D. P. Shum and R. L. Carlin, Inorg. Chem., 1989, 28, 272 CrossRef;
(b) O. Kahn, Molecular Magnetism, VCH Publishers, New York, 1993 Search PubMed;
(c) C. Benelli, A. Caneschi, D. Gatteschi and R. Sessoli, J. Appl. Phys., 1993, 73, 5333 CrossRef CAS;
(d) M. L. Kahn, J. P. Sutter, S. Golhen, P. Guionneau, L. Ouahab, O. Kahn and D. Chasseau, J. Am. Chem. Soc., 2000, 122, 3413 CrossRef CAS;
(e) O. Evans and W. Lin, Chem. Mater., 2001, 13, 3009 CrossRef CAS;
(f) M. T. Lemaire, Pure Appl. Chem., 2004, 76, 277 CrossRef CAS.
-
(a) G. St-Pierre, A. Chagnes, N. A. Bouchard, P. D. Harvey, L. Brossard and H. Menard, Langmuir, 2004, 20, 6365 CrossRef CAS PubMed;
(b) A. B. Descalzo, K. Rurack, H. Weisshoff, R. Martinez-Manez, M. D. Marcos, P. Amoros, K. Hoffmann and J. Soto, J. Am. Chem. Soc., 2005, 127, 184 CrossRef CAS PubMed;
(c) D. Luneau and P. Rey, Coord. Chem. Rev., 2005, 249, 2591 CrossRef CAS;
(d) M. A. Aldamen, J. M. Clemente-Juan, E. Coronado, C. Marti-Gastaldo and A. Gaita-Arino, J. Am. Chem. Soc., 2008, 130, 8874 CrossRef CAS PubMed.
-
(a) R. Sessoli, D. Gatteschi, A. Caneschi and M. A. Novak, Nature, 1993, 365, 141 CrossRef CAS;
(b) R. Wang, D. Song and S. Wang, Chem. Commun., 2002, 368 RSC;
(c) M. Romanelli, G. A. Kumar, T. J. Emge, R. E. Riman and J. G. Brennan, Angew. Chem., Int. Ed., 2008, 47, 6049 CrossRef CAS PubMed;
(d) M. Mannini, F. Pineider, P. Sainctavit, C. Danieli, E. Otero, C. Sciancalepore, A. M. Talarico, M. A. Arrio, A. Cornia, D. Gatteschi and R. Sessoli, Nat. Mater., 2009, 8, 194 CrossRef CAS PubMed;
(e) S. V. Eliseeva and J. C. G. Bünzli, Chem. Soc. Rev., 2010, 39, 189 RSC.
-
(a) M. N. Leuenberger and D. Loss, Nature, 2001, 410, 789 CrossRef CAS PubMed;
(b) M. J. Graham, J. M. Zadrozny, M. Shiddiq, J. S. Anderson, M. S. Fataah, S. Hill and D. E. Freedman, J. Am. Chem. Soc., 2014, 136, 7623 CrossRef CAS PubMed.
-
(a) W. Liang, M. P. Shores, M. Bockrath, J. R. Long and H. Park, Nature, 2002, 417, 725 CrossRef CAS PubMed;
(b) L. Sorace, C. Benelli and D. Gatteschi, Chem. Soc. Rev., 2011, 40, 3092 RSC;
(c) D. N. Woodruff, R. E. P. Winpenny and R. A. Layfield, Chem. Rev., 2013, 113, 5110 CrossRef CAS PubMed;
(d) S. Thiele, R. Vincent, M. Holzmann, S. Klyatskaya, M. Ruben, F. Balestro and W. Wernsdorfer, Phys. Rev. Lett., 2013, 111, 037203 CrossRef CAS PubMed.
-
(a) Y. C. Chen, J. L. Liu, L. Ungur, J. Liu, Q. W. Li, L. F. Wang, Z. P. Ni, L. F. Chibotaru, X. M. Chen and M. L. Tong, J. Am. Chem. Soc., 2016, 138, 2829 CrossRef CAS PubMed;
(b) D. N. Woodruff, R. E. P. Winpenny and R. A. Layfield, Chem. Rev., 2013, 113, 5110 CrossRef CAS PubMed;
(c) F. Habib and M. Murugesu, Chem. Soc. Rev., 2013, 42, 278 RSC.
-
(a) M. A. AlDamen, J. M. Clemente-Juan, E. Coronado, C. Marti-Gastaldo and A. Gaita-Arino, J. Am. Chem. Soc., 2008, 130, 8874 CrossRef CAS PubMed;
(b) C. R. Ganivet, B. Ballesteros, G. D. L. Torre, J. M. Clemente-Juan, E. Coronado and T. Torres, Chem.–Eur. J., 2012, 19, 1457 CrossRef PubMed;
(c) R. J. Blagg, L. Ungur, F. Tuna, J. Speak, P. Comar, D. Collison, W. Wernsdorfer, E. J. L. McInnes, L. F. Chibotaru and R. E. P. Winpenny, Nat. Chem., 2013, 5, 673 CrossRef CAS PubMed;
(d) P. Zhang, L. Zhang, C. Wang, S. Xue, S. Y. Lin and J. Tang, J. Am. Chem. Soc., 2014, 136, 4484 CrossRef CAS PubMed;
(e) L. Ungur, J. J. Le Roy, I. Korobkov, M. Murugesu and L. F. Chibotaru, Angew. Chem., Int. Ed., 2014, 53, 4413 CrossRef CAS PubMed;
(f) A. Upadhyay, S. K. Singh, C. Das, R. Mondol, S. K. Langley, K. S. Murray, G. Rajaraman and M. Shanmugam, Chem. Commun., 2014, 50, 8838 RSC;
(g) J. Liu, Y. C. Chen, J. L. Liu, V. Vieru, L. Ungur, J. H. Jia, L. F. Chibotaru, Y. H. Lan, W. Wernsdorfer, S. Gao, X. M. Chen and M. L. Tong, J. Am. Chem. Soc., 2016, 138, 5441 CrossRef CAS PubMed.
-
(a) Y. N. Guo, G. F. Xu, W. Wernsdorfer, L. Ungur, Y. Guo, J. Tang, H. J. Zhang, L. F. Chibotaru and A. K. Powell, J. Am. Chem. Soc., 2011, 133, 11948 CrossRef CAS PubMed;
(b) X. J. Zhang, V. Vieru, X. W. Feng, J. L. Liu, Z. J. Zhang, B. Na, W. Shi, B. W. Wang, A. K. Powell, L. F. Chibotaru, S. Gao, P. Cheng and J. R. Long, Angew. Chem., Int. Ed., 2015, 54, 9861 CrossRef PubMed;
(c) W. Huang, F. X. Shen, S. Q. Wu, L. Liu, D. Y. Wu, Z. Zheng, J. Xu, M. Zhang, X. C. Huang, J. Jiang, F. F. Pan, Y. Li, K. Zhu and O. Sato, Inorg. Chem., 2016, 55, 5476 CrossRef CAS PubMed.
-
(a) J. H. I. Tang, N. T. Madhu, G. Chastanet, W. Wernsdorfer, C. E. Anson, C. Benelli, R. Sessoli and A. K. Powell, Angew. Chem., Int. Ed., 2006, 45, 1729 CrossRef CAS PubMed;
(b) S. Xue, L. Zhao, Y. N. Guo, P. Zhang and J. K. Tang, Chem. Commun., 2012, 48, 8946 RSC.
-
(a) J. W. Sharples, Y. Z. Zheng, F. Tuna, E. J. L. McInnes and D. Collison, Chem. Commun., 2011, 47, 7650 RSC;
(b) R. J. Blagg, C. A. Muryn, E. J. L. McInnes, F. Tuna and R. E. P. Winpenny, Angew. Chem., Int. Ed., 2011, 50, 6530 CrossRef CAS PubMed;
(c) S. Y. Lin, W. Wernsdorfer, L. Ungur, A. K. Powell, Y. N. Guo, J. Tang, L. Zhao, L. F. Chibotaru and H. J. Zhang, Angew. Chem., Int. Ed., 2012, 51, 12767 CrossRef CAS PubMed;
(d) S. Das, S. Hossain, A. Dey, S. Biswas, J. P. Sutter and V. Chandrasekhar, Inorg. Chem., 2014, 53, 5020 CrossRef CAS PubMed;
(e) G. J. Wang, Y. Q. Wei and K. C. Wu, Dalton Trans., 2016, 45, 12734 RSC;
(f) Y.-S. Ding, N. F. Chilton, R. E. P. Winpenny and Y.-Z. Zheng, Angew. Chem., Int. Ed., 2016, 55, 16071 CrossRef CAS PubMed;
(g) S. K. Gupta, T. Rajeshkumar, G. Rajaraman and R. Murugavel, Chem. Sci., 2016, 7, 5181 RSC.
-
(a) F. S. Guo, B. M. Day, Y. C. Chen, M. L. Tong, A. Mansikkamäki and R. A. Layfield, Angew. Chem., Int. Ed., 2017, 56, 11445 CrossRef CAS PubMed;
(b) C. A. P. Goodwin, F. Ortu, D. Reta, N. F. Chilton and R. A. Mills, Nature, 2017, 548, 439 CrossRef CAS PubMed.
- C. J. Milios, A. Vinslava, W. Wernsdorfer, S. Moggach, S. Parsons, S. P. Perlepes, G. Christou and E. K. Brechin, J. Am. Chem. Soc., 2007, 129, 2754 CrossRef CAS PubMed.
- J. D. Rinehart, M. Fang, W. J. Evans and J. R. Long, Nat. Chem., 2011, 3, 538 CrossRef CAS PubMed.
-
(a) A. Caneschi, D. Gatteschi, N. Lalioti, C. Sangregorio, R. Sessoli, G. Venturi, A. Vindigni, A. Rettori, M. G. Pini and M. A. Novak, Angew. Chem., Int. Ed., 2001, 40, 1760 CrossRef CAS;
(b) L. Bogani, C. Sangregorio, R. Sessoli and D. Gatteschi, Angew. Chem., Int. Ed., 2005, 44, 5817 CrossRef CAS PubMed;
(c) N. Ishii, T. Ishida and T. Nogami, Inorg. Chem., 2006, 45, 3837 CrossRef CAS PubMed;
(d) K. Bernot, L. Bogani, A. Caneschi, D. Gatteschi and R. Sessoli, J. Am. Chem. Soc., 2006, 128, 7947 CrossRef CAS PubMed;
(e) G. Poneti, K. Bernot, L. Bogani, A. Caneschi, R. Sessoli, W. Wernsdorferc and D. Gatteschi, Chem. Commun., 2007, 1807 RSC;
(f) N. Ishii, Y. Okamura, S. Chiba, T. Nogami and T. Ishida, J. Am. Chem. Soc., 2008, 130, 24 CrossRef CAS PubMed.
-
(a) X. L. Wang, L. C. Li and D. Z. Liao, Inorg. Chem., 2010, 49, 4735 CrossRef CAS PubMed;
(b) R. Liu, L. Li, X. Wang, P. Yang, C. Wang, D. Liao and J. P. Sutter, Chem. Commun., 2010, 46, 2566 RSC;
(c) R. Liu, Y. Ma, P. Yang, X. Song, G. Xu, J. Tang, L. Li, D. Liao and S. Yan, Dalton Trans., 2010, 39, 3321 RSC;
(d) T. Han, W. Shi, Z. Niu, B. Na and P. Cheng, Chem.–Eur. J., 2013, 19, 994 CrossRef CAS PubMed;
(e) Y. L. Wang, Y. Ma, X. Yang, J. K. Tang, P. Cheng, Q. L. Wang, L. C. Li and D. Z. Liao, Inorg. Chem., 2013, 52, 7380 CrossRef CAS PubMed;
(f) P. Hu, X. Wang, Y. Ma, Q. Wang, L. Li and D. Liao, Dalton Trans., 2014, 43, 2234 RSC;
(g) L. L. Li, S. Liu, H. Li, W. Shi and P. Cheng, Chem. Commun., 2015, 51, 10933 RSC.
-
(a) J. D. Rinehart, M. Fang, W. J. Evans and J. R. Long, J. Am. Chem. Soc., 2011, 133, 14236 CrossRef CAS PubMed;
(b) M. Ballesteros-Rivas, H. Zhao, A. Prosvirin, E. W. Reinheimer, R. A. Toscano, J. Valdés-Martínez and K. R. Dunbar, Angew. Chem., Int. Ed., 2012, 51, 5124 CrossRef CAS PubMed;
(c) S. Demir, J. M. Zadrozny, M. Nippe and J. R. Long, J. Am. Chem. Soc., 2012, 134, 18546 CrossRef CAS PubMed;
(d) S. Demir, M. I. Gonzalez, L. E. Darago, W. J. Evans and J. R. Long, Nat. Commun., 2018, 8, 2144 CrossRef PubMed.
-
(a) Y. Pei, O. Kalzn, M. A. Aebersold, L. Ouahab, F. L. Berre, L. Pardi and J. L. Tholence, Adv. Mater., 1994, 6, 681 CrossRef CAS;
(b) A. Lang, Y. Pei, L. Ouahab and O. Kahn, Adv. Mater., 1996, 8, 60 CrossRef CAS;
(c) J. P. Sutter, A. Lang, O. Kahn, C. Paulsen, L. Ouahab and Y. Pei, J. Magn. Magn. Mater., 1997, 171, 147 CrossRef CAS;
(d) J. P. Sutter, M. L. Kahn, S. Golhen, L. Ouahab and O. Kahn, Chem.–Eur. J., 1998, 4, 571 CrossRef CAS;
(e) B. Gillon, M. A. Aebersold, O. Kahn, L. Pardi and B. Delley, Chem. Phys., 1999, 250, 23 CrossRef CAS;
(f) N. Daro, J. P. Sutter, M. Pink and O. Kahn, J. Chem. Soc., Perkin Trans. 2, 2000, 1087 RSC.
-
(a) SCALE3 ABSPACK: Empirical absorption correction, CrysAlis-Software package, Oxford Diffraction Ltd, Oxford, 2006 Search PubMed;
(b) CrysAlisPro, Agilent Technologies, Yarnton, Oxfordshire, England, 2010 Search PubMed.
- G. Sheldrick, Acta Crystallogr., Sect. A: Fundam. Crystallogr., 2008, 64, 112 CrossRef CAS PubMed.
-
(a) J. P. Sutter, M. L. Kahn, S. Golhen, L. Ouahab and O. Kahn, Chem.–Eur. J., 1998, 4, 571 CrossRef CAS;
(b) X. L. Mei, R. N. Liu, C. Wang, P. P. Yang, L. C. Li and D. Z. Liao, Dalton Trans., 2012, 41, 2904 RSC;
(c) Y. L. Wang, Y. Y. Gao, Y. Ma, Q. L. Wang, L. C. Li and D. Z. Liao, CrystEngComm, 2012, 14, 4706 RSC;
(d) C. Lescop, G. Bussiere, R. Beaulac, H. Beslisle, E. Beloeizky, P. Rey, C. Reber and D. Luneau, J. Phys. Chem. Solids, 2004, 65, 773 CrossRef CAS;
(e) S. Y. Zhou, X. Li, T. Li, L. Tian, Z. Y. Liu and X. G. Wang, RSC Adv., 2015, 5, 17131 RSC.
-
(a) H. X. Tian, R. N. Liu, X. L. Wang, P. P. Yang, Z. X. Li, L. C. Li and D. Z. Liao, Eur. J. Inorg. Chem., 2009, 4498 CrossRef CAS;
(b) N. Zhou, Y. Ma, C. Wang, G. F. Xu, J. K. Tang, J. X. Xu, S. P. Yan, P. Cheng, L. C. Li and D. Z. Liao, Dalton Trans., 2009, 8489 RSC;
(c) X. L. Mei, Y. Ma, L. C. Li and D. Z. Liao, Dalton Trans., 2012, 41, 505 RSC.
-
(a) K. Bernot, J. Luzon, L. Bogani, M. Etienne, C. Sangregorio, M. Shanmugam, A. Caneschi, R. Sessoli and D. Gatteschi, J. Am. Chem. Soc., 2009, 131, 5573 CrossRef CAS PubMed;
(b) P. H. Lin, T. J. Burchell, R. Clérac and M. Murugesu, Angew. Chem., Int. Ed., 2008, 47, 8848 CrossRef CAS PubMed;
(c) Y. Ma, G. F. Xu, X. Yang, L. C. Li, J. K. Tang, S. P. Yan, P. Cheng and D. Z. Liao, Chem. Commun., 2010, 46, 8264 RSC;
(d) S. Osa, T. Kido, N. Matsumoto, N. Re, A. Pochaba and J. Mrozinski, J. Am. Chem. Soc., 2004, 126, 420 CrossRef CAS PubMed;
(e) Y. M. Bing, N. Xu, W. Shi, K. Liu and P. Cheng, Chem.–Asian J., 2013, 8, 1412 CrossRef CAS PubMed.
-
(a) D. N. Woodruff, R. E. P. Winpenny and R. A. Layfield, Chem. Rev., 2013, 113, 5110 CrossRef CAS PubMed;
(b) H. L. C. Feltham and S. Brooker, Coord. Chem. Rev., 2014, 276, 1 CrossRef CAS;
(c) J. D. Rinehart and J. R. Long, Chem. Sci., 2011, 2, 2078 RSC.
Footnote |
† Electronic supplementary information (ESI) available. CCDC 1831067–1831069. For ESI and crystallographic data in CIF or other electronic format see DOI: 10.1039/c8ra02546k |
|
This journal is © The Royal Society of Chemistry 2018 |
Click here to see how this site uses Cookies. View our privacy policy here.