DOI:
10.1039/C8RA01827H
(Paper)
RSC Adv., 2018,
8, 19754-19764
Fabrication of Sb3+ sensor based on 1,1′-(-(naphthalene-2,3-diylbis(azanylylidene))bis(methanylylidene))bis(naphthalen-2-ol)/nafion/glassy carbon electrode assembly by electrochemical approach†
Received
1st March 2018
, Accepted 16th May 2018
First published on 29th May 2018
Abstract
A new Schiff base named 1,1′-(-(naphthalene-2,3-diylbis(azanylylidene))bis (methanylylidene))bis(naphthalen-2-ol) (NDNA) derived from 2,3-naphthalenediamine and 2-hydroxy-1-naphthaldehyde was synthesized by condensation reaction and then characterized by spectroscopic techniques for structure elucidation. In addition to spectroscopic techniques, the molecular structure of NDNA was clearly confirmed by single-crystal X-ray diffraction study. A thin film of NDNA was fabricated onto glassy carbon electrode (GCE) using 5.0% ethanolic nafion solution as a conducting binder in order to develop the cationic electrochemical sensor (NDNA/nafion/GCE) for the sensing of heavy-metal cations in aqueous systems by electrochemical technique. This newly designed sensor exhibited higher sensitivity and selectivity towards antimony (Sb3+) in the presence of other interfering heavy metal cations, as well as long-term stability. Fascinating analytical parameters such as limit of detection (LOD = 0.075 nM, SNR of 3), limit of quantification (LOQ = 0.25 nM) and sensitivity (12.658 × 10−4 μA μM−1 cm−2) were calculated from the calibration curve plot, which shows a linear dynamic range (LDR) of Sb3+ ion concentration from 0.1–10.0 mM. This work presents a new approach towards the development of sensitive, efficient as well as selective toxic cationic electrochemical sensors in the environmental and healthcare fields. Hence, this newly designed NDNA/nafion/GCE presents cost-effective and efficient outcomes and can be used as a practical substitute for the efficient detection and removal of Sb3+ ions from water samples.
1. Introduction
Heavy-metal ions badly affect the aquatic environment, consequently damaging human health. The pollution by heavy metals occurs via many routes, such as industrial effluents, refineries, waste-treatment plants, groundwater and rainwater.1 Therefore, studies are growing toward making suitable devices for sensing and detecting such traces in the environment.2–4 In this interest, the Schiff base, which is the condensation product of aldehyde (or ketone) and amine-containing compounds,5,6 seems to be a viable ligand for sensing metal ions.4,7,8 Schiff bases have shown several biological activities such as antibacterial, antifungal, antimalarial, anti-inflammatory, and antipyretic.9 These properties are mainly due to the imine group present in Schiff bases, which could also be altered by varying the substituents in the molecules.10–12 Furthermore, several studies have explored the properties of Schiff bases as catalysts,13–16 fluorescent materials,17–19 electroluminescent materials,20–22 etc. Since Schiff bases are known by their strong coordinative ability as a family of ligands, with the formation in most cases of 1
:
1 transition metal complexes,23 they have been used to develop various chemosensors.24–26 Chemosensors can be classified into three categories according to the nature of the signal emitted by the signaling subunit: (i) colorimetric sensors related to change in electronic properties in the form of intra/intermolecular charge transfer (ICT),27–29 (ii) fluorogenic sensors related to photoinduced electron transfer (PET),27,30 excited-state intramolecular proton transfer (ESIPT),31 fluorescence resonance energy transfer (FRET),32,33 bond energy transfer (TBET),34 excimer–exciplex formation,35 etc., and (iii) electrochemical sensors related to measurement of changes in redox potential or electrical responses. Because of the great worth and commercial applications of the Schiff bases, the intention of this study is to design an ion-selective cationic electrochemical sensor based on a newly synthesized, non-reported Schiff base as tetradentate ligand in order to probe heavy metal ions in an aqueous system by electrochemical approach. For this reason, 2,3-naphthalenediamine and 2-hydroxy-1-naphthaldehyde were selected as precursors to synthesize the novel Schiff base, named as NDNA. It was observed that the newly designed cationic electrochemical sensor based on the above Schiff base is selective for Sb3+ in the presence of other interfering heavy metal ions in aqueous system.
The toxicity of antimony on health includes keratitis, dermatitis, gastritis, and conjunctivitis, and long-term exposure can cause the development of cardiac problems and lung cancer.36,37 Aquatic environments are polluted by Sb through rock weathering, soil runoff and anthropologic activities.38,39 The toxicity of antimony strongly depends on its oxidation state, as (Sb3+) is about ten times more toxic than (Sb4+).40,41 The maximum permissible concentrations in drinking water as recommended by the U.S. Environmental Protection Agency (EPA) and World Health Organization (WHO) are 6 and 20 mg L−1, respectively.42,43 Even though concentrations as low as 1 mg L−1 Sb can be found in a non-polluted water sample,44 high concentrations of Sb can reach 100 mg L−1 in nearby anthropogenic sources.45 Therefore, the detection and monitoring of Sb have been compulsory. Various analytical approaches such as UV-Vis spectrophotometer,46,47 capillary electrophoresis,48 spectrofluorimetry,49 atomic absorption spectrometry,50–54 laser-induced fluorescence,55 inductively coupled plasma-optical emission spectrometry (ICP-OES),42,56 high-performance liquid chromatography coupled with hydride generation-atomic fluorescence spectrometry (HPLC-HG-AFS),57,58 etc. have been reported for the determination of antimony (Sb3+) in addition to the various electrochemical methods.59,60 Voltammetric well as potentiometric methods based on modified mercury electrodes as working electrodes have been reported for the detection of antimony.61,62 Many of the reported methods are not very effective with respect to their detection limit. If some are effective, then they are very complicated and not very cost-effective. So, it has become our need to develop a cost-effective, reliable and efficient method for the effective determination of Sb3+. In this research, current–voltage (I–V) technique, an electrochemical approach, was applied for the effective determination of Sb3+ by using a newly designed, selective and efficient electrochemical sensor (NDNA/nafion/GCE) in a lab-made electrochemical cell which accommodates aqueous solution. Glassy carbon electrode (GCE) was used as a working electrode/sensor for the trace determination of Sb3+. It was modified by the newly synthesized Schiff base (NDNA) coated onto its flat surface with 5% ethanolic nafion as a conducting binder. The modified electrode shows very sensitive and excellent transduction in phosphate buffer solution (PBS, 0.1 M at 7.0 pH) at the interface of the liquid and electrode surface. Per our knowledge, this is the first cationic sensing application based on the lab-made tetradentate NDNA Schiff base as chelating agent for the highly sensitive, selective and rapid determination of antimony(III) in aqueous solution, qualitatively and quantitatively, using I–V method with a short response time.
2. Experimental
2.1. Materials and methods
All solvents and reagents were purchased from Sigma-Aldrich Company and used as received. 1H and 13C NMR spectra were recorded in DMSO-d6 solutions on a Bruker Avance 850 MHz spectrometer. Infrared spectra were performed on a PerkinElmer spectrum 100 FTIR spectrometer. FT-IR spectra were recorded as neat on a Thermo Scientific NICOLET iS50 FT-IR spectrometer (Thermo Scientific, Madison, WI, USA). Melting points were determined in open capillary tubes in a Stuart Scientific melting point apparatus (SMP3) and are uncorrected.
2.2. X-ray crystallography of compound I (NDNA)
To observe the geometry of molecules and their interactions in the unit cell, we have crystalized the compound I (NDNA). Good-looking crystals were taken to the microscope for the final selection of sample for mounting on the diffractometer. The selected crystal was fixed over the tip of a thin glass fiber adsorbed in wax on a copper rod with a magnetic base. This holder was mounted on an Agilent SuperNova (dual source) Agilent Technologies Diffractometer, equipped with graphite-monochromatic Cu/Mo Kα radiation source for data collection. The data collection was accomplished using the CrysAlisPro software63 at 296 K under Cu Kα radiation. The structure solution was performed and refined by full-matrix least-squares methods on F2 using SHELXL-97,64 in-built with WinGX.65 All non-hydrogen atoms were refined anisotropically by full-matrix least-squares methods.64 Figures were drawn using PLATON66 and ORTEP-3.67
All the aromatic hydrogen atoms were positioned geometrically and treated as riding atoms with C–H = 0.93 Å and Uiso (H) = 1.2 Ueq (C) carbon atoms. The N–H = 0.99(6) Å and O–H = 0.93(6) Å; the hydrogen atoms were located with the difference Fourier map and refined with Uiso (H) = 1.2 Ueq (N) and Uiso (H) = 1.5 Ueq (O), respectively. The CIF of the NDNA has been submitted to the Cambridge Crystallographic Data Centre (CCDC).
2.3. Synthesis of NDNA
A solution of 2,3-naphthalenediamine (1.58 g, 10 mmol) and 2-hydroxy-1-naphthaldehyde (3.44 g, 20 mmol) in 200 mL of 50/50 v/v ethanol/methanol (absolute) was stirred for 24 h at room temperature and then refluxed for 8 h, then left to cool. The orange precipitate was filtered and washed several times with ethanol to yield 4.15 g product (90% yield), re-crystallized from chloroform/methanol to obtain the single crystal for further analysis. Mp: 324–326 °C. 1H NMR (DMSO-d6, 850 MHz, δ = ppm): 7.09 (2H, d, J = 8.5 Hz, Ar–H), 7.40 (2H, t, J = 6.8 Hz, Ar–H), 7.57 (2H, m, Ar–H), 7.60 (2H, td, J = 7.22, 0.85 Hz, Ar–H), 7.85 (2H, d, J = 7.65 Hz, Ar–H), 7.99 (2H, d, J = 9.35 Hz, Ar–H), 8.10 (2H, m, Ar–H), 8.31 (2H, s, Ar–H), 8.61 (2H, d, J = 8.5 Hz, H–Ar), 9.83 (2H, s, N
CH), 15.08 (2H, s, OH); 13C NMR (DMSO-d6, 213 MHz, δ = ppm): 19.01, 109.89, 117.17, 121.16, 122.01, 124.22, 126.81, 127.41, 128.15, 128.73, 129.54, 132.59, 133.50, 137.47, 138.78, 157.89, 158.89, 169.36; FT-IR (ATR, cm−1): 3675, 3054, 1618, 1607, 1593, 1568, 1542, 1490, 1350, 1307, 1162, 863, 739.
2.4. Fabrication of electrochemical sensor
A very easy and effortless method was applied to fabricate the GCE with newly synthesized non-reported Schiff base as chelating agent. A very small amount (approximately 1.5 mg) of NDNA was mixed with 0.1 mL of ethanol in order to make the slurry, which was then applied onto the flat surface of the GCE, with one drop of 5% ethanolic nafion as an adhesive conducting binder, Scheme 1. Herein, nafion was used as an adhesive conducting polymer in order to adhere NDNA to the flat surface of GCE and allow the conduction of electrons between NDNA and GCE against the potential (V) applied in the system during the detection of toxic cations. After coating, it was dried at room temperature for 45 minutes to obtain the completely dried, evenly coated and stable NDNA/nafion/GCE as an efficient and selective cationic electrochemical sensor for Sb3+. A Pt wire was also used as counter electrode in addition to the newly designed NDNA/nafion/GCE as working electrode to measure the I–V response. In this way, a lab-made electrochemical cell was formed which accommodates the two electrodes (working and counter electrodes) to dip into phosphate buffer solution (PBS) of pH = 7.0, in a 15 mL beaker. The volume of PBS was kept constant at 10 mL in this lab-made electrochemical cell throughout the study. The PBS was prepared by mixing 39 mL of 0.2 M Na2HPO4 and 61 mL of 0.2 M NaH2PO4 in a 200 mL measuring cylinder, diluting to the mark by deionized (DI) water. The stock solution of Sb3+ was used to make the different concentrations of Sb3+ (full concentration range: 0.1 nM to 0.1 M) in DI water for use as our target analyte. Linear dynamic range (LDR), regression coefficient r2, sensitivity, limit of detection (LOD) at S/3N, and limit of quantification (LOQ) for Sb3+ was calculated from the slope of the calibration curve (from current versus concentration plot). Keithley electrometer was used as a constant voltage source for I–V measurement in a simple two-electrode system.
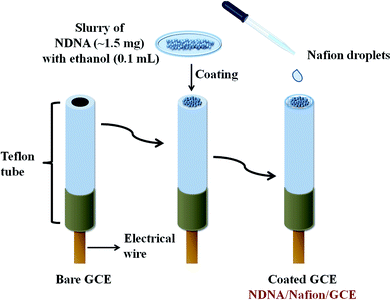 |
| Scheme 1 Fabrication of glassy carbon electrode modified by NDNA with the conducting binder nafion. | |
3. Results and discussion
3.1. Synthesis
The idea behind the present work is to have a bifunctional fused aromatic Schiff base that would be suitable for making a stable film on an electrochemical device. For this purpose, NDNA Schiff base was easily obtained (Scheme 2) in excellent yield by the condensation reaction of 2-hydroxynaphthaldehyde and 2,3-naphthalenediamine. ATR-FTIR, 1H NMR, 13C and single-crystal X-ray spectroscopy measurements were made to confirm the chemical structure of NDNA. The FTIR spectra show several characteristic peaks, for the azomethine group (CH
N) at 1618 and aromatic rings (C
C) at 1607, 1593, 1568, and 1542 cm−1. Additionally, C–H aromatic stretching vibration is clearly observed at 3054 cm−1. Other bands appear at 1490, 1350, and 1307 cm−1, attributed to C–H bending vibration, C–N stretching vibration and C–O stretching vibration, respectively. An ortho-di-substituted aromatic band appears at 739 cm−1. The phenolic O–H stretching vibration appears weak, with a broad peak centered at 3675 cm−1. This behavior might be due to intramolecular hydrogen bonding with the azomethine nitrogen atom. The proton NMR data show the imine and OH protons as singlet, far downfield at 9.83 and 15.08 ppm, respectively. This downfield effect is attributed to the formation of intramolecular hydrogen bonds, which results in further de-shielding of the OH group protons. Also, the creation of a six-membered ring as a result of intramolecular hydrogen bonds (see Scheme 3) may further impact the OH proton by the magnetic anisotropic effect. Previous studies indicated that intramolecular hydrogen bonds have a very significant effect on phenolic protons, with chemical shifts in the range of 4.5 to 19 ppm.22 Good correlation between doublet and triplet protons with their corresponding coupling constants are clearly indicative of the neighboring aromatic protons as presented in the experimental section. Additionally, 13C NMR recorded all carbons present in the NDNA, with a special attention to the characteristic peak of aromatic azomethine carbon at 169.36 ppm.
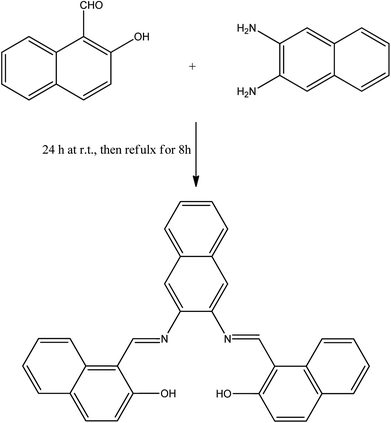 |
| Scheme 2 Synthesis of proposed NDNA compound. | |
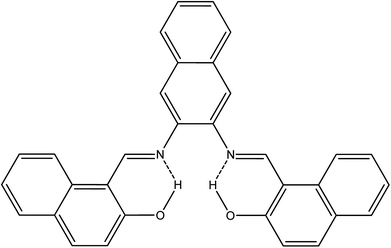 |
| Scheme 3 Expected intramolecular hydrogen bonds in NDNA. | |
3.2. Single-crystal X-ray description of synthesized compound
The CCDC number (1812136) obtained for the NDNA is mentioned in Table 1. There are three naphthalene rings (C1–C10), (C12–C21) and (C23–C32) in the molecule (Fig. 1). The root mean square (r.m.s.) deviations for the fitted atoms of these naphthalene ring systems are 0.0086 Å, 0.0222 Å and 0.0363 Å. Bond angles and bond lengths are shown in Tables S1 and S2.† Two of the naphthalene rings are almost planar with respect to each other, as the dihedral angle between the (C1–C10) and (C12–C21) is 4.060 (4)°, while the dihedral angle between the naphthalene rings (C1–C10) and (C23–C32) is 60.694 (8)°. The molecule exhibits inter- and intra-molecular hydrogen bonding interactions. There are N–H⋯O and O–H⋯N type intramolecular interactions from the six-membered ring motifs shown in Fig. 1.68 The ring motif generated through the atoms (O1/C13/C12/C11/N1/H1n) have the r.m.s. deviation of 0.0301 Å, and its dihedral angle with its fused naphthalene ring is 3.298 (9)°. The second ring motif generated through the atoms (N2/C22/C23/C24/O2/H1o) has almost the same r.m.s. deviation, i.e. 0.0325 Å, and is twisted at a dihedral angle of 7.727 (1)° with respect to the naphthalene ring (C23–C32). The intermolecular hydrogen bonding interactions observed in the crystal structure of compound I are weak, but they connect the molecules to generate the intricate network. Atom C(6) in the molecule occupied at (x, y, z) takes part in hydrogen bonding and acts as donor atom via H(6) to atom O(1) at (1 − X, 1 − Y, 1/2 + Z). This interaction connects the molecules along the c-axis to form infinite long chains (Fig. 2 and S1,† Table 2). On the other hand, the atom C(22) at (x, y, z) acts as donor via H(6) to atom O(1) at (X, 1 + Y, Z) and connects the molecules along the b-axis (Fig. 2 and S1,† Table 2). Both interactions produce the network along the bc plane.
Table 1 Crystal data and structure refinement for I
CCDC code |
1812136 |
Empirical formula |
C32H22N2O2 |
Formula weight |
566.51 |
Temperature/K |
296(2) |
Crystal system |
Orthorhombic |
Space group |
Pca21 |
a/Å |
20.7011(17) |
b Å |
6.2155(4) |
c/Å |
18.0683(13) |
α/° |
90 |
β/° |
90 |
γ/° |
90 |
Volume/Å3 |
2324.8(3) |
Z |
4 |
ρcalc mg mm−3 |
1.333 |
μ/mm−1 |
0.084 |
F(000) |
976.0 |
Crystal size/mm3 |
0.29 × 0.21 × 0.14 |
2θ range for data collection |
5.986 to 58.31° |
Index ranges |
−27 ≤ h ≤ 26, −8 ≤ k ≤ 5, −24 ≤ l ≤ 24 |
Reflections collected |
13 650 |
Independent reflections |
5313[R(int) = 0.0505] |
Data/restraints/parameters |
5313/1/332 |
Goodness-of-fit on F2 |
1.042 |
Final R indexes [I ≥ 2σ(I)] |
R1 = 0.0543, wR2 = 0.1103 |
Final R indexes [all data] |
R1 = 0.1126, wR2 = 0.1369 |
Largest diff. peak/hole/e Å−3 |
0.14/−0.14 |
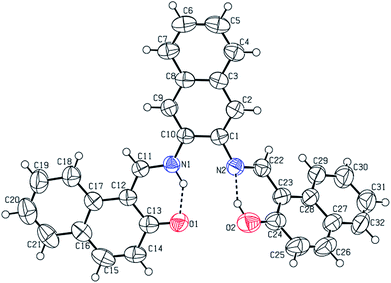 |
| Fig. 1 Labelled ORTEP diagram of molecule I (17082), where thermal ellipsoids were drawn at 50% probability level. | |
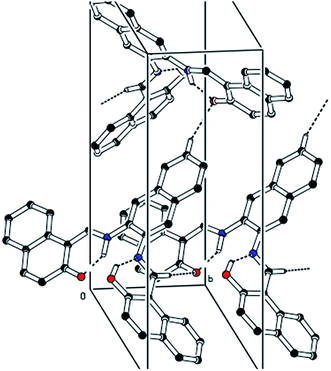 |
| Fig. 2 A unit cell view showing the inter- and intra-molecular hydrogen bonding using dashed lines. | |
Table 2 Hydrogen bonds for 17082
D |
H |
A |
d(D–H)/Å |
d(H–A)/Å |
d(D–A)/Å |
D–H–A/° |
1 − X, 1 − Y,1/2 + Z. +X, 1 + Y, +Z. |
C6 |
H6 |
O1a |
0.93 |
2.55 |
3.278(5) |
135.0 |
C22 |
H22 |
O1b |
0.93 |
2.50 |
3.363(5) |
155.4 |
N1 |
H1N |
O1 |
0.99(6) |
1.68(6) |
2.547(4) |
144(4) |
O2 |
H1O |
N2 |
0.93(6) |
1.76(6) |
2.580(5) |
146(6) |
3.3. Application: detection of Sb3+ ions with NDNA/nafion/GCE probe
The impressive application of NDNA is the electrochemical sensing of heavy metal cations in aqueous solution by using I–V approach. The fabrication of GCE with NDNA as chelating agent has been discussed in the experimental section. The creation of the NDNA/nafion/GCE as an efficient and selective cationic electrochemical sensor in a two-electrode system (working and counter electrodes) is a new report as per our knowledge, and no other reports are found in the literature. Moreover, the sensor was found to be very selective and sensitive for Sb3+ in the presence of other interfering heavy metal cations. The notable change in current response of the newly designed NDNA/nafion/GCE against applied potential was observed in the presence of our target analyte in aqueous solution upon being adsorbed on the surface of the working electrode. After the fruitful result of a change in the current response of the modified GCE, analytical parameters such as LDR, LOD, LOQ, etc. were also studied in order to optimize the newly designed NDNA/nafion/GCE as an efficient, sensitive and as well as selective cationic electrochemical sensor for Sb3+.
Initially, the change in current response with this newly designed NDNA/nafion/GCE against the applied potential was observed and compared with the bare GCE in the absence of target analyte. It was noticed that the fabricated GCE has a high current response as compared to the bare GCE, which reflects the high electron communication feature between the active site of NDNA and GCE (Fig. 3a). Subsequently, a selectivity study was carried out in the presence of various heavy metal cations such as Sb3+, As3+, Ce3+, Cr3+, Cu2+, Hg2+, Ni2+, Sn2+ and Y3+, and it was found to be very selective only for Sb3+ (Fig. 3b). For selectivity study, 25 μL of 0.1 μM concentration of toxic cations was used. Moreover, the current response in the presence and absence of Sb3+ was also observed in order to confirm the fabricated GCE's affinity with Sb3+, and it was found that it shows very good response in the presence of Sb3+ (Fig. 3c). It also reflects the very good adsorption and absorption capability of the Sb3+analytes onto the coated surface of the GCE. Similarly, the I–V response of nafion/GCE in the presence and absence of Sb3+ analytes was also observed in order to confirm that nafion, as conducting binder, also has no significant effect without NDNA for the sensing of Sb3+ in the system. It was noticed that the response of nafion/GCE without NDNA was almost same and has no significant effect in the presence and absence of Sb3+ (Fig. 3d). PBS solutions of different pH values were also investigated in order to optimize the operational condition of the newly designed GCE (Fig. S1 presented in ESI†). It was found that this newly designed GCE gives good response at pH 7.0 and 1.0 second as delay time in the presence of Sb3+.
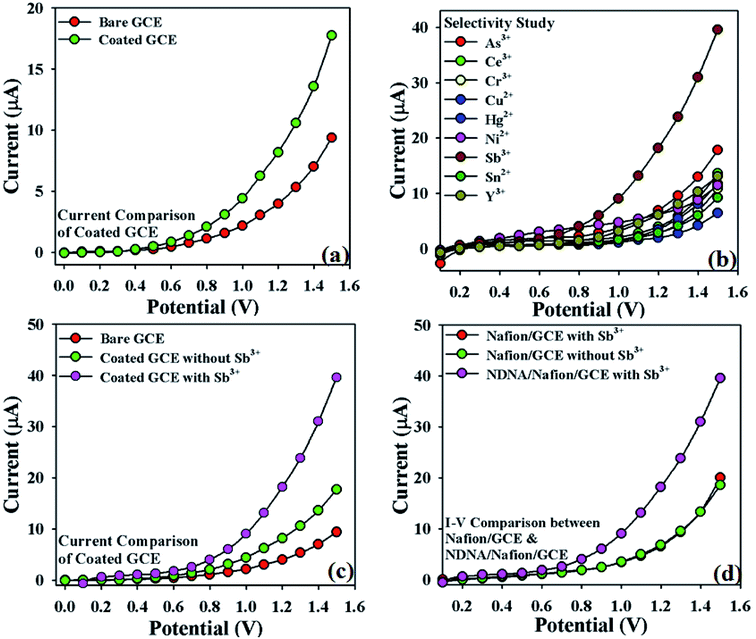 |
| Fig. 3 Selectivity study: (a) I–V response of bare and NDNA-coated GCE; (b) I–V response with various analytes (showing affinity with Sb3+), concentration of each analyte was taken at 0.1 μM, 25 μL; (c) comparison of I–V response of bare and NDNA/nafion/GCE with and without the target analyte Sb3+ at 0.1 μM, 25 μL; (d) comparison of I–V response of nafion/GCE and NDNA/nafion/GCE with and without the target analyte. | |
Similarly, statistical approach was also applied to study the interference effect of other heavy metal cations (As3+, Ce3+, Cr3+, Cu2+, Hg2+, Ni2+, Sn2+ and Y3+) on NDNA/nafion/GCE in the presence of Sb3+ analytes, in the form of I–V response at +1.0 V at normal condition, and results are presented in Fig. 4 and Table 3. The concentration and volume of all cations were kept constant, and the amounts were taken as 25 μL of 0.1 μM cation in PBS (7.0 pH). From the interference study, it was also concluded that NDNA/nafion/GCE is very selective toward Sb3+ detection. It does not exhibit any major change in current response towards the other interfering heavy metal cations compared to Sb3+. For the Sb3+, it has given a 3-fold current response compared to Ni2+, which gave the second highest current response.
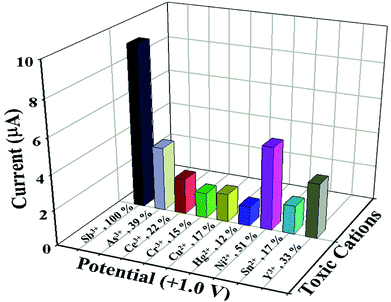 |
| Fig. 4 Interference study: comparison of I–V responses to interfering heavy metal cations at +1.0 V. Analyte concentrations were taken as 0.1 μM, delay time 1.0 s. | |
Table 3 Interference effect of various cations with NDNA/nafion/GCEa
Metal ions |
Observed current (μA) |
Interference effect (%) |
SD (n = 3) |
RSD (%) (n = 3) |
R1 |
R2 |
R3 |
Average |
Interference effect of Sb3+ is considered to be 100%; R = reading; SD = standard deviation; and RSD = relative standard deviation; n = number of readings. |
Sb3+ |
9.0214 |
9.2104 |
9.6461 |
9.2926 |
100 |
0.320 |
3.44 |
As3+ |
3.7026 |
3.1622 |
4.2134 |
3.6927 |
39 |
0.525 |
14.23 |
Ce3+ |
2.0452 |
2.5146 |
1.6454 |
2.0684 |
22 |
0.435 |
21.03 |
Cr3+ |
1.3736 |
1.5109 |
1.4631 |
1.4492 |
15 |
0.069 |
4.80 |
Cu2+ |
1.5437 |
1.3383 |
1.9694 |
1.6171 |
17 |
0.321 |
19.90 |
Hg2+ |
1.0972 |
0.9170 |
1.3465 |
1.1202 |
12 |
0.215 |
19.25 |
Ni2+ |
4.7218 |
5.0739 |
4.4944 |
4.7633 |
51 |
0.291 |
6.12 |
Sn2+ |
1.5799 |
1.4827 |
1.8366 |
1.6330 |
17 |
0.182 |
11.19 |
Y3+ |
3.1301 |
2.4540 |
3.6794 |
3.1301 |
33 |
0.613 |
19.87 |
The proposed mechanism of Sb3+ detection by using NDNA/nafion/GCE is shown in Scheme 4, with I–V graphical responses. I–V response of the newly designed NDNA/nafion/GCE in the presence of Sb3+ ions is functional in PBS at normal condition, and good response is observed. The current response of NDNA/nafion/GCE in the absence of Sb3+ is also presented in Scheme 4a in order to compare the current response in the presence of Sb3+ (Scheme 4b). When we add a small amount of Sb3+ into the system, there is a small increase in the current response of NDNA/nafion/GCE because a smaller surface of coated GCE is covered by the Sb3+ analytes (π–π* interaction). In this way, the surface reaction proceeds continuously but slowly and surely. Similarly, current response increases progressively with gradual increase of the concentration of Sb3+ analytes in the system (π–π* interaction). Subsequently, we can get a better idea about the increases in current response from the concentration variation plot (Fig. 5a). The surface reaction does not stop at this stage but increases progressively as a function of the concentration of our target analytes. Thus, we observe a greater current response of the NDNA/nafion/GCE-film as more surface is covered by our target analytes, along with increasing π–π* interaction of the functional groups of NDNA and Sb3+ (Scheme 4b). The π–π* interaction could be approached as inter-molecular and intra-molecular interactions of the functional compound. Similarly, the same phenomena have also been reported for the detection of toxic chemicals in the literature.2,69 Finally, Sb3+analytes on the NDNA/nafion/GCE surface reach the ideal saturated stage, which results in a regular increment of current response (Scheme 3c).
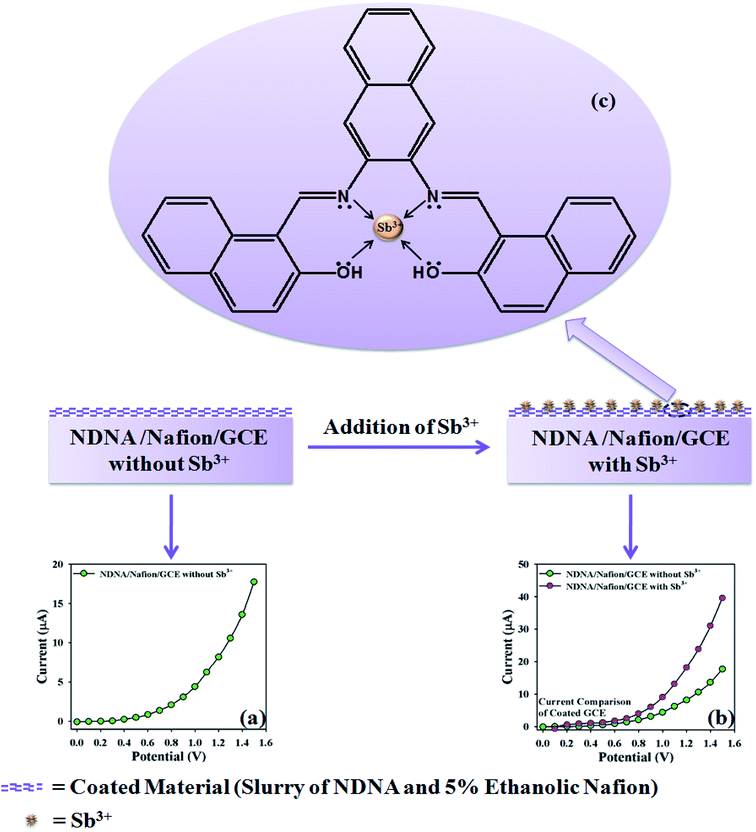 |
| Scheme 4 Proposed mechanism of the probable interaction of Sb3+ with NDNA/nafion/GCE, (a) I–V response without Sb3+, (b) comparison of I–V response with and without Sb3+, (c) probable π–π* interaction between Sb3+ ions and NDNA. | |
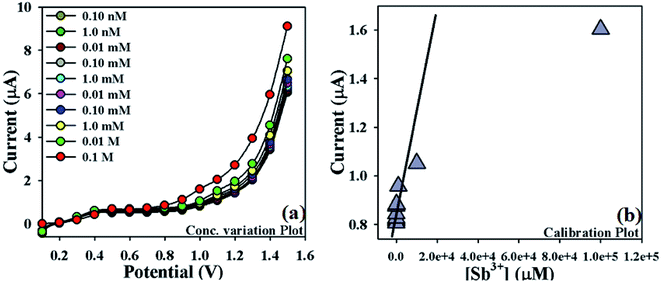 |
| Fig. 5 Optimization of newly designed Sb3+ sensor: (a) concentration variation plot of Sb3+ (0.1 M to 0.1 nM), (b) calibration plot (at +1.0 V) of NDNA/nafion/GCE. | |
In order to optimize the NDNA/nafion/GCE as an efficient cationic electrochemical sensor for Sb3+, current responses at different concentrations of Sb3+ analytes in aqueous system against the applied potential were also observed. It was noticed that current increases as a function of Sb3+ concentration from lower to higher values (0.1 nM to 0.1 M) at normal condition (Fig. 5a). Analytical parameters such as linear dynamic range (LDR), regression coefficient (r2), limit of detection (LOD) and limit of quantification (LOQ) as well as sensitivity were found by plotting the calibration curve at the potential of +1.0 V from various concentrations of Sb3+ analytes (Fig. 5b). From the plot, the linear dynamic range (LDR), regression coefficient (r2), sensitivity, and limits of detection (LOD) and quantification (LOQ) were calculated as 0.1 nM to 10.0 mM, (r2) = 0.9710, 12.658 × 10−4 μA μM−1 cm−2, 0.075 nM and 0.25 nM, respectively.
In order to validate this newly designed NDNA/nafion/GCE, the repeatability test was carried out at the concentration of 0.1 μM with a sequence of nine to ten successive measurements (Fig. 6a). No significant changes were observed in current responses in addition to the absence of electrode poisoning and erosion during the detection of Sb3+ at normal condition. I–V response remained almost the same as the initial response after washing for each experiment. On the other hand, Fig. 6b shows the plot of response time of the newly designed NDNA/nafion/GCE recorded as current (μA) vs. time (sec) (I–T) measurement. It took 10 to 13 seconds to reach the saturated steady-state current. This plot indicates that the newly designed NDNA/nafion/GCE is very efficient for the detection of Sb3+ analytes even at very low concentration and within a short response time.
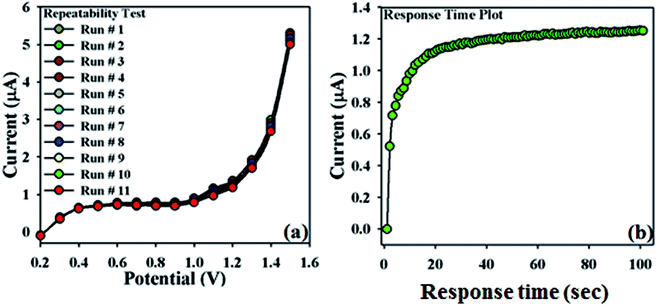 |
| Fig. 6 Repeatability and response time: (a) repeatability test (1 to 11 runs) with 25 μL of 0.1 μM Sb3+, (b) response time of 10 to 13 seconds to reach the saturated steady state. | |
The sensitivity of the newly designed NDNA/nafion/GCE is due to its outstanding absorption (assembly of NDNA-modified GCE) and adsorption (surface of NDNA/nafion/GCE) properties. In addition, high catalytic decomposition characteristics and high electron communication features in the active sites of NDNA and GCE make it sensitive. The sensitivity and detection limit of this cationic sensor are in good according with the previously reported methods for the detection of Sb3+. The NDNA/nafion/GCE system introduces a very simple and reliable method to detect toxic chemicals, and it also reveals a significant access to a large group of chemicals for wide-ranging applications in environmental and healthcare fields. This study is the initial report for the sensitive and selective detection of Sb3+ by I–V technique based on the NDNA/nafion/GCE as compared to other previously reported methods. Table 4 shows the evaluation of the proposed I–V technique for the detection of Sb3+ using NDNA/nafion/GCE compared with other previously reported analytical techniques. It indicates that this outstanding proposed method, with our designed NDNA/nafion/GCE as a sensor for probing the Sb3+, is more sensitive and efficient than previously reported methods.
Table 4 Comparison of proposed electrochemical method with different previously reported analytical methods for the detection of Sb3+a
Methods |
Material |
Sensitivity |
LDR |
LOD |
LOQ |
Ref. |
PLS = partial least squares; CPE = cloud point extraction; DCHNAQ-CTAB = 3-dichloro-6-(3-carboxy-2-hydroxy-1-naphthylazo)quinoxaline-cetyltrimethylammonium bromide; SPE-ETAAS = solid-phase extraction-electrothermal atomic absorption spectrometry; APDC = ammonium pyrrolidinedithiocarbamate; ICP-OES = inductively coupled plasma optical emission spectrometry; 2ClRAAP = 3-o-chlorophenyl-5-(2-arsenoxylphenylazo) rhodanine; HPLC-HG-AFS = high-performance liquid chromatography-hydride generation-atomic fluorescence spectrometry detection; SMDE = static mercury drop electrode; SPGE = screen printed graphite electrode; p-DMPF = p-dimethyl-aminophenyl-fluorone; HMDE = hanging mercury drop electrode; NDNA = 1′-(-(naphthalene-2,3-diylbis(azanylylidene))bis(methanylylidene))bis(naphthalen-2-ol); GCE = glassy carbon electrode. |
UV-Vis spectroscopy/PLS |
Pyrogallol as complexing agent |
— |
1.0 × 10−6 to 1.0 × 10−4 M |
39 800 nM |
— |
González et al.46 |
UV-Vis spectroscopy/CPE |
DCHNAQ-CTAB |
— |
1.6 × 10−9 to 1.6 × 10−7 M |
0.45 nM |
1.51 nM |
El-Sharjawy et al.47 |
Spectrofluorimetry |
2ClRAAP |
— |
1.6 × 10−9 to 8.2 × 10−8 M |
1.35 nM |
— |
Yu et al.49 |
SPE-ETAAS |
APDC/carbon nanotubes |
— |
4.1 × 10−10 to 3.28 × 10−8 M |
0.41 nM |
— |
López-García et al.51 |
ICP-OES |
Immobilized L-proline |
— |
0.0–4.1 × 10−7 M |
0.74 nM |
— |
Menegário et al.56 |
HPLC-HG-AFS |
Anion exchange column PRP-X100 |
— |
4.1 × 10−9 to 1.6 × 10−6 M |
0.574 nM |
— |
De Gregori et al.58 |
Adsorptive cathodic stripping voltammetry |
Morin/SMDE |
— |
1.0 × 10−9 to 3.0 × 10−7 M |
0.7 nM |
— |
Zhou et al.59 |
Anodic stripping voltammetry |
Pot. ferrocyanide/SPGE |
— |
8.2 × 10−9 to 7.47 × 10−6 M |
4.76 nM |
— |
V. Kolliopoulos et al.60 |
Square wave voltammetry |
HMDE |
— |
4.1 × 10−9 to 6.5 × 10−8 M |
1.0 nM |
— |
Locatelli et al.61 |
Potentiometric |
p-DMPF/HMDE |
— |
8.2 × 10−8 to 4.1 × 10−7 M |
0.40 nM |
— |
Zhang et al.62 |
I–V method NDNA/nafion/GCE |
NDNA/nafion/GCE |
12.658 × 10−4 μA μM−1 cm−2 |
1.0 × 10−10 to 0.01 M |
0.075 nM |
0.25 nM |
This work |
3.4. Real sample analysis
Real sample analysis was carried out in order to validate this proposed I–V method by using NDNA/nafion/GCE through the standard addition method.70 Industrial effluents, a baby feeding bottle, a PVC food packing bag, and a mineral water bottle were used for this purpose. A fixed amount of each sample (25.0 μL) was analyzed in PBS (0.1 M, pH = 7.0). The results (Table 5) obtained by this method were in good accord with the proposed I–V technique, which indicates that this technique is also reliable, satisfactory and stable for analyzing real samples with the newly designed NDNA/nafion/GCE as an efficient and selective Sb3+ cationic electrochemical sensor.
Table 5 Real sample analysis of Sb3+ in various environmental samplesa
Real samples |
Amount of 3-CP added |
No. of readings |
Measured response in (μA) |
% recovery |
Mean (% recovery) |
SD |
RSD |
SEM |
SD = standard deviation; RSD = relative standard deviation; SEM = standard error of mean. |
Sb3+ |
0.1 μM, 25 μL |
— |
21.073 |
100 |
— |
— |
— |
— |
Industrial effluent |
0.1 μM, 25 μL |
R1 |
16.262 |
77.1 |
73.0 |
3.857 |
5.27 |
2.22 |
R2 |
14.646 |
69.5 |
R3 |
15.297 |
72.5 |
Plastic baby feeding bottle |
0.1 μM, 25 μL |
R1 |
23.351 |
110.8 |
96.3 |
13.338 |
13.84 |
7.70 |
R2 |
19.747 |
93.7 |
R3 |
17.812 |
84.5 |
Polythene food packaging |
0.1 μM, 25 μL |
R1 |
10.875 |
51.6 |
48.7 |
2.559 |
5.25 |
1.47 |
R2 |
10.074 |
47.8 |
R3 |
9.849 |
46.7 |
Mineral water bottle |
0.1 μM, 25 μL |
R1 |
12.718 |
60.3 |
54.4 |
5.301 |
9.72 |
3.06 |
R2 |
11.185 |
53.0 |
R3 |
10.544 |
50.0 |
4. Conclusions
A new NDNA Schiff base was easily synthesized, and its molecular structure was confirmed by singly-crystal X-ray diffraction studies to have the dimensions of a = 20.7011(17) Å, b = 6.2155(4) Å, c = 18.0683(13) Å, space group = Pca2 and Z = 4. The final R2 was 0.1369 (all data), and R1 was 0.0543 (I > 2\s(I)). We observed inter- and intra-molecular hydrogen bonding interactions which provide stability to the crystal structure. The N–H⋯O and O–H⋯N type intramolecular interactions form the six-membered ring motifs. The NDNA was used to fabricate a new electrochemical sensor (NDNA/nafion/GCE) for the detection of Sb3+ from simulated as well as real water samples in the presence of other interfering heavy metal cations by using the I–V method. This novel study gives a good proposal for the selective and very sensitive detection of Sb3+ analytes by using NDNA/nafion/GCE as an antimony probe with short response time and good reproducibility as well as repeatability. Hence, this technique launches a new approach that can be applied for the development of new electrochemical sensors for probing other heavy metal cations in environmental and healthcare fields.
Conflicts of interest
The authors declare no conflict of interest.
Acknowledgements
This article was funded by the Deanship of Scientific Research (DSR) at King Abdulaziz University, Jeddah. The authors, therefore, acknowledge with thanks DSR for technical and financial support.
References
- I. Samb, J. Bell, P. Y. Toullec, V. Michelet and I. Leray, Org. Lett., 2011, 13, 1182–1185 CrossRef PubMed
. - T. A. Sheikh, M. N. Arshad, M. M. Rahman, A. M. Asiri, H. M. Marwani, R. Awual and W. A. Bawazir, Inorg. Chim. Acta, 2017, 464, 157–166 CrossRef
. - M. M. Hussain, M. M. Rahman, M. N. Arshad and A. M. Asiri, ACS Omega, 2017, 2, 420–431 CrossRef
. - M. N. Arshad, T. A. Sheikh, M. M. Rahman, A. M. Asiri, H. M. Marwani and M. R. Awual, J. Organomet. Chem., 2017, 827, 49–55 CrossRef
. - X. Li, Z. Liu, Y. Xu and D. Wang, J. Inorg. Biochem., 2017, 171, 37–44 CrossRef PubMed
. - J. L. Segura, M. J. Mancheño and F. Zamora, Chem. Soc. Rev., 2016, 45, 5635–5671 RSC
. - M. N. Arshad, M. M. Rahman, A. M. Asiri, T. R. Sobahi and S.-H. Yu, RSC Adv., 2015, 5, 81275–81281 RSC
. - V. K. Gupta, M. R. Ganjali, P. Norouzi, H. Khani, A. Nayak and S. Agarwal, Crit. Rev. Anal. Chem., 2011, 41, 282–313 CrossRef PubMed
. - A. Kathiravan, K. Sundaravel, M. Jaccob, G. Dhinagaran, A. Rameshkumar, D. Arul Ananth and T. Sivasudha, J. Phys. Chem. B, 2014, 118, 13573–13581 CrossRef PubMed
. - M. J. O'donnell, Acc. Chem. Res., 2004, 37, 506–517 CrossRef PubMed
. - P. Przybylski, A. Huczynski, K. Pyta, B. Brzezinski and F. Bartl, Curr. Org. Chem., 2009, 13, 124–148 CrossRef
. - Z. Guo, R. Xing, S. Liu, Z. Zhong, X. Ji, L. Wang and P. Li, Carbohydr. Res., 2007, 342, 1329–1332 CrossRef PubMed
. - A. Lehwess-Litzmann, P. Neumann, C. Parthier, S. Lüdtke, R. Golbik, R. Ficner and K. Tittmann, Nat. Chem. Biol., 2011, 7, 678 CrossRef PubMed
. - P. Adão, M. L. Kuznetsov, S. Barroso, A. M. Martins, F. Avecilla and J. C. Pessoa, Inorg. Chem., 2012, 51, 11430–11449 CrossRef PubMed
. - W. Yang, H. Liu and D.-M. Du, Org. Biomol. Chem., 2010, 8, 2956–2960 Search PubMed
. - S.-H. Hsieh, Y.-P. Kuo and H.-M. Gau, Dalton Trans., 2007, 97–106 RSC
. - S.-H. Li, F.-R. Chen, Y.-F. Zhou, J.-N. Wang, H. Zhang and J.-G. Xu, Chem. Commun., 2009, 4179–4181 RSC
. - S. A. Lee, G. R. You, Y. W. Choi, H. Y. Jo, A. R. Kim, I. Noh, S.-J. Kim, Y. Kim and C. Kim, Dalton Trans., 2014, 43, 6650–6659 RSC
. - A. Ganguly, B. K. Paul, S. Ghosh, S. Kar and N. Guchhait, Analyst, 2013, 138, 6532–6541 RSC
. - C.-M. Che, S.-C. Chan, H.-F. Xiang, M. C. W. Chan, Y. Liu and Y. Wang, Chem. Commun., 2004, 1484–1485 RSC
. - L. Zhou, C.-C. Kwok, G. Cheng, H. Zhang and C.-M. Che, Opt. Lett., 2013, 38, 2373–2375 CrossRef PubMed
. - T. Sano, Y. Nishio, Y. Hamada, H. Takahashi, T. Usuki and K. Shibata, J. Mater. Chem., 2000, 10, 157–161 RSC
. - E. M. Mohie and R. M. El-Shishtawy, Asian J. Chem., 2013, 25, 2719 Search PubMed
. - D. M. Epstein, S. Choudhary, M. R. Churchill, K. M. Keil, A. V Eliseev and J. R. Morrow, Inorg. Chem., 2001, 40, 1591–1596 CrossRef PubMed
. - L. Salmon, P. Thuéry, E. Rivière and M. Ephritikhine, Inorg. Chem., 2006, 45, 83–93 CrossRef PubMed
. - N. Roy, A. Dutta, P. Mondal, P. C. Paul and T. S. Singh, Sens. Actuators, B, 2016, 236, 719–731 CrossRef
. - D. Maity and T. Govindaraju, Inorg. Chem., 2011, 50, 11282–11284 CrossRef PubMed
. - Q. Zhao, F. Li and C. Huang, Chem. Soc. Rev., 2010, 39, 3007–3030 RSC
. - Y. J. Na, Y. W. Choi, G. R. You and C. Kim, Sens. Actuators, B, 2016, 223, 234–240 CrossRef
. - T. Gunnlaugsson, H. D. P. Ali, M. Glynn, P. E. Kruger, G. M. Hussey, F. M. Pfeffer, C. M. G. dos Santos and J. Tierney, J. Fluoresc., 2005, 15, 287–299 CrossRef PubMed
. - M. Y. Berezin and S. Achilefu, Chem. Rev., 2010, 110, 2641–2684 CrossRef PubMed
. - Z.-X. Han, X.-B. Zhang, Z. Li, Y.-J. Gong, X.-Y. Wu, Z. Jin, C.-M. He, L.-X. Jian, J. Zhang and G.-L. Shen, Anal. Chem., 2010, 82, 3108–3113 CrossRef PubMed
. - C.-Y. Li, Y. Zhou, Y.-F. Li, C.-X. Zou and X.-F. Kong, Sens. Actuators, B, 2013, 186, 360–366 CrossRef
. - G.-S. Jiao, L. H. Thoresen and K. Burgess, J. Am. Chem. Soc., 2003, 125, 14668–14669 CrossRef PubMed
. - Y. Zhang, Y. Fang, N.-Z. Xu, M.-Q. Zhang, G.-Z. Wu and C. Yao, Chin. Chem. Lett., 2016, 27, 1673–1678 CrossRef
. - J. Su, S. Zhong, X. Li and H. Zou, J. Electrochem. Soc., 2014, 161, H512–H516 CrossRef
. - J. O. Vinhal, A. D. Gonçalves, G. F. B. Cruz and R. J. Cassella, Talanta, 2016, 150, 539–545 CrossRef PubMed
. - L. A. Portugal, L. Ferrer, A. M. Serra, D. G. da Silva, S. L. C. Ferreira and V. Cerdà, J. Anal. At. Spectrom., 2015, 30, 1133–1141 RSC
. - I. Shtangeeva, R. Bali and A. Harris, J. Geochem. Explor., 2011, 110, 40–45 CrossRef
. - Y. Li, B. Hu and Z. Jiang, Anal. Chim. Acta, 2006, 576, 207–214 CrossRef PubMed
. - M. Eftekhari, M. Chamsaz, M. H. Arbab-Zavar and A. Eftekhari, Environ. Monit. Assess., 2015, 187, 4129 CrossRef PubMed
. - H. R. Hansen and S. A. Pergantis, J. Anal. At. Spectrom., 2006, 21, 731–733 RSC
. - F. Edition, WHO Chron., 2011, 38, 104–108 Search PubMed
. - M. Filella, N. Belzile and Y.-W. Chen, Earth-Sci. Rev., 2002, 57, 125–176 CrossRef
. - W. Shotyk and M. Krachler, J. Environ. Monit., 2009, 11, 1747–1753 RSC
. - M. J. G. González, O. D. Renedo and M. J. A. Martínez, Talanta, 2005, 68, 67–71 CrossRef PubMed
. - A.-A. M. El-Sharjawy and A. S. Amin, Anal. Biochem., 2016, 492, 1–7 CrossRef PubMed
. - C. Casiot, M. C. B. Alonso, O. F. X. Donard, M. Potin-Gautier and J. Boisson, Analyst, 1998, 123, 2887–2893 RSC
. - J. Yu, P. Dai, S. Ge, Y. Zhu, L. Zhang and X. Cheng, Spectrochim. Acta, Part A, 2009, 72, 17–21 CrossRef PubMed
. - I. López-García, S. Rengevicova, M. J. Muñoz-Sandoval and M. Hernández-Córdoba, Talanta, 2017, 162, 309–315 CrossRef PubMed
. - I. López-García, R. E. Rivas and M. Hernández-Córdoba, Talanta, 2011, 86, 52–57 CrossRef PubMed
. - A. de Jesus, M. B. Dessuy, C. S. Huber, A. V. Zmozinski, Á. T. Duarte, M. G. R. Vale and J. B. Andrade, Microchem. J., 2016, 124, 222–227 CrossRef
. - D. Sanchez-Rodas, W. T. Corns, B. Chen and P. B. Stockwell, J. Anal. At. Spectrom., 2010, 25, 933–946 RSC
. - T. Kubota, A. Kawakami, T. Sagara, N. Ookubo and T. Okutani, Talanta, 2001, 53, 1117–1126 CrossRef PubMed
. - J. Enger, A. Marunkov, N. Chekalin and O. Axner, J. Anal. At. Spectrom., 1995, 10, 539–549 RSC
. - A. A. Menegário, P. Smichowski, P. S. Tonello, G. Polla, E. P. Oliveira and R. E. Santelli, Anal. Chim. Acta, 2008, 625, 131–136 CrossRef PubMed
. - I. De Gregori, W. Quiroz, H. Pinochet, F. Pannier and M. Potin-Gautier, Talanta, 2007, 73, 458–465 CrossRef PubMed
. - I. De Gregori, W. Quiroz, H. Pinochet, F. Pannier and M. Potin-Gautier, J. Chromatogr. A, 2005, 1091, 94–101 CrossRef PubMed
. - C. Zhou, Y. Lu, X. Li, C.-N. Luo, Z. Zhang and J. You, Talanta, 1998, 46, 1531–1536 CrossRef PubMed
. - A. V Kolliopoulos, J. P. Metters and C. E. Banks, Anal. Methods, 2013, 5, 3490–3496 RSC
. - C. Locatelli, J. Sci. Food Agric., 2007, 87, 305–312 CrossRef
. - X. Zhang, C. Ma, L. Wang and J. Zhang, Talanta, 1995, 42, 897–900 CrossRef PubMed
. - O. D. CrysAlisPRO, 2012 Search PubMed
. - G. M. Sheldrick, Acta Crystallogr., Sect. A: Found. Crystallogr., 2008, 64, 112–122 CrossRef PubMed
. - L. J. Farrugia, J. Appl. Crystallogr., 2012, 45, 849–854 CrossRef
. - A. L. Spek, A Multipurpose Crystallographic Tool, PhD thesis, Utr. Univ. Utrecht, Netherlands, 2007
. - L. J. Farrugia, J. Appl. Crystallogr., 1999, 32, 837–838 CrossRef
. - J. Bernstein, R. E. Davis, L. Shimoni and N. Chang, Angew. Chem., Int. Ed. Engl., 1995, 34, 1555–1573 CrossRef
. - T. A. Sheikh, M. N. Arshad, M. M. Rahman, A. M. Asiri and K. A. Alamry, J. Organomet. Chem., 2016, 822, 53–61 CrossRef
. - I. Ahmad, M. N. Arshad, M. M. Rahman, A. M. Asiri, T. A. Sheikh and F. M. Aqlan, Inorg. Chim. Acta, 2017, 467, 297–306 CrossRef
.
Footnote |
† Electronic supplementary information (ESI) available: CCDC 1812136. For ESI and crystallographic data in CIF or other electronic format see DOI: 10.1039/c8ra01827h |
|
This journal is © The Royal Society of Chemistry 2018 |