DOI:
10.1039/C8RA01235K
(Paper)
RSC Adv., 2018,
8, 13408-13416
Natural assembly of a ternary Ag–SnS–TiO2 photocatalyst and its photocatalytic performance under simulated sunlight
Received
8th February 2018
, Accepted 5th April 2018
First published on 10th April 2018
Abstract
Natural assembly method was utilized to prepare a novel ternary Ag–SnS–TiO2 nanocomposite, in which TiO2 nanobelts were used as templates. The co-loading of Ag and SnS nanoparticles endows TiO2 nanobelts with enhanced photocatalytic capability, resulting from the broadened light absorption spectra and decreased band gaps. Comparing with raw TiO2 nanobelts and commercial Degussa P25, an improvement in photodegradation of simulated organic pollutants was successfully demonstrated due to the decreasing recombination of photogenerated electron–hole pairs. Our work presents a new strategy for the preparation of ternary TiO2-based photocatalysts in the practical application of wastewater treatment.
1. Introduction
With the growth of population and urbanization, the energy crisis and environmental pollution have emerged as two urgent challenges. Consequently, development of clean and sustainable alternative energy sources has attracted more and more attention, due to the decreasing availability of fossil fuels.1,2 Another effective strategy is to discover an adaptable catalyst to degrade the pre-existing contaminants. In developing countries, the ubiquitous release of organic pollutants including dyes, pigments, drugs, saturated hydrocarbons, aromatic compounds insecticides, and pesticides in the water resources is posing an irreversible problem to the environments and ecosystem. Owing to the green, benign, and cost effective properties, heterogeneous photocatalysts such as TiO2, ZnO, Fe2O3, CdS, ZnS, SnO2, Bi2O3, V2O5 have been widely developed for efficient photo-degradation of various harmful organic pollutants in the wastewater. In particular, TiO2 has attracted growing attentions due to its abundant reserves, low cost, non-toxicity, good light stability and high UV photocatalytic (PC) activity in nature.3–7 Nevertheless, the extensive practical applications of TiO2 are highly impeded by the unexpected diminishment of PC degradation efficiency resulted from certain factors such as electron (e−)/hole (h+) recombination. In addition, the wide band gap (3.2 eV) prohibits TiO2 to efficiently absorb the solar light, leading to low light utilization efficiency (less than 5%).8,9 Two significant strategies has been presented to overcome these drawbacks,6 which are coupling TiO2 with other semiconductors and addition of hybrid dopants (e.g., hydrogenation, noble metal modification and transition metals).10 The utility of semiconductors with narrower band gaps to fabricate TiO2-based P–N heterostructures is a reasonable method to improve the performance of TiO2. The P–N heterojunction not only creates a potential gradient at the junction interface, but also transfers electrons from the excited narrow-band gap semiconductor to another adhesion layer, in order to promote the light utilization efficiency. Therefore, a series of semiconductor quantum dots (QDs) have been widely used as photosensitizers to form P–N heterojunction then improve the utilization efficiency of solar energy.11–13 In particular, stannous sulfide (SnS), a typical p-type semiconductor material, possesses narrow direct band gap (1.3 eV) and indirect band gap (1.09 eV), exhibiting excellent performance in solar absorption and photocatalysis.14,15 As expected, SnS–TiO2 binary composite provides an effective platform to significantly improve the electron transport properties.15,16 Besides, addition of hybrid dopants is another effective method to promote the PC efficiency, owing to the good advantages on lower Fermi energy level and light absorption in visible region.17–22 In particular, Ag–TiO2 binary composite has received great attention in recent years, due to the direct sunlight PC activity and excellent chemical stability.19 The PC activity of Ag–TiO2 binary composite can be tuned by regulating nanoparticle loading, dispersion, shape, size and metal–support interaction.23 Lu et al.24 have prepared Ag–TiO2 nanorods by UV irradiation, exhibiting higher electron transport efficiency than that of pure TiO2 nanorods. Yang et al. have reported that Ag–TiO2 nanotube heterojunction possessed higher photocatalytic activity than pure TiO2 nanotubes.9 Herein, we firstly propose a new design of ternary Ag–SnS–TiO2 nanobelts photocatalyst prepared from TiO2 NBs. The obtained ternary nanocomposite showed broader visible light absorption and higher PC activity than the pristine TiO2 NBs and commercial P25, owing to the synergetic effects from Ag and SnS nanoparticles.
2. Experimental section
2.1 Materials
TiO2 (Degussa, P-25), sodium hydroxide (NaOH), hydrochloric acid (HCl), sodium sulfide (NaS), stannous chloride dihydrate (SnCl2·2H2O), silver nitrate (AgNO3), nitric acid (HNO3), ethanol (C2H5OH), methylene blue (MB), carbon tetrachloride (CCl4) and rhodamine B (RHB) were purchased from Sinopharm Chemical Co., Ltd. without further purification.
2.2 Preparation of TiO2 NBs
In a typical reaction, 0.3 g of P25 was mixed with 80 mL of 10 M NaOH aqueous solution. The mixture was transferred to a 100 mL Teflon-lined stainless steel autoclave. Followed by heating at 180 °C for 48 hours, the mixture was air cooled to room temperature. The obtained dispersion was filtered and thoroughly washed with deionized water for five times. The wet powder was immersed in 200 mL of 0.1 M HCl aqueous solution, stirring for 24 hours. Followed by washing with distilled water, the obtained H2Ti3O7 precursors were annealed at 500 °C for 3 hours to prepare bare TiO2 NBs.
2.3 Preparation of Ag–SnS–TiO2 NBs
Ternary Ag–SnS–TiO2 NBs were fabricated by natural assembly from photo-reduction technology25,26 and SILAR method,27,28 as shown in Fig. 1. Raw TiO2 NBs were synthesized by a alkali-hydrothermal method by using commercially available TiO2 (Degussa, P-25) as the titanium source.29 To facilitate the adsorption of Ag nanoparticles and deposition of SnS nanoparticles, hydroxyl groups were introduced onto the surface of TiO2 NBs, through soaking the nanobelts in concentrated nitric acid.30 The hydroxyl groups not only benefit the deposition of SnS and Ag, but also prevent the aggregation of nanoparticles. Following, Ag nanoparticles are decorated on TiO2 NBs after a moderate photo-reductive deposition. Followed by doping SnS onto the Ag–TiO2 NBs by SILAR method, the ternary Ag–SnS–TiO2 NBs were successfully synthesized. The overall reactions in each procedure can be described as follows:
Na2Ti3O7 + 2HCl → H2Ti3O7 + 2NaCl |
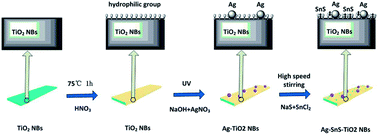 |
| Fig. 1 Assembly procedure for preparation of Ag–SnS–TiO2 NBs. | |
In a typical procedure, 0.2 g of TiO2 NBs was soaked in 100 mL of 10 M concentrated nitric acid for 1 hour, to introduce a large amount of hydroxyl groups onto the surface of TiO2 NBs. Ag nanoparticles are decorated on TiO2 NBs by constant ultraviolet light deposition. 0.2 g of TiO2 NBs and 100 mL of 0.001 M AgNO3 solution were mixed together, stirring and exposing to under a high-pressure mercury lamp for 6 hours. The power of the high-pressure mercury lamp is 300 W, and the distance between the solution and the lamp is 20 cm. Following, the samples are rinsed with ultrapure water and dried at 60 °C for 12 hours to obtain Ag–TiO2 NBs. SnS is deposited on Ag–TiO2 NBs by a modified successive ion layer absorption and reaction technique (SILAR) method.31 First, 0.2 g of Ag–TiO2 NBs was immersed in 100 mL of 1 M SnCl2 solution for 10 min and rinsed with ultrapure water and filtered, followed by rinsing with 200 mL of 1 M Na2S solution. After washing with ultrapure water and filtrating, the SILAR process was repeated 6 times to obtain Ag–SnS–TiO2 NBs precursors. Then, the precursor was sintered in an argon atmosphere (>99.9%) at 500 °C for 2 hours to prepare the final ternary Ag–SnS–TiO2 NBs.
2.4 PC activity test
Methylene blue (MB) (3.0 × 10−5 mol L−1, 100 mL, pH ≈ 6.0) and rhodamine B (RHB) (3.0 × 10−5 mol L−1, 100 mL, pH ≈ 6.0) were used as representative dye indicators to evaluate PC activity of Ag–SnS–TiO2 photocatalysts. Taking MB as an example, 20 mg of ternary Ag–SnS–TiO2 NBs photocatalyst was dispersed ultrasonically in MB aqueous solution and sonicated in the dark for 10 minutes to ensure the establishment of adsorption/desorption equilibrium. The mixture was loaded into a glass cylindrical jacketed vessel and exposed to a 300 W metal halide lamp, continuously agitating with an irradiation distance of 25 cm and an irradiation area of 31 cm2. The entire PC reaction is carried out at room temperature with vigorous stirring and water cooling. About 3.0 mL aliquot was removed at a given time interval and the catalyst was separated from the suspension by filtration through a 0.22 μm cellulose membrane. The PC degradation process was monitored by UV-vis spectrophotometer (Lambda 950; measurement of MB absorption at 664 nm; measurement of RHB absorption at 552 nm). Comparative experiments with electron (e−) or hole (h+) trapping agents were performed in the same manner except that 20 mL of ethanol or carbon tetrachloride were added.
2.5 Characterization
The phase and microstructure of the samples were investigated by Raman spectroscopy, X-ray diffraction (XRD), high resolution transmission electron microscopy (HRTEM) and X-ray photoelectron spectroscopy (XPS). Raman spectroscopy was performed using a Renishaw in via microscope with 488 nm Ar ion laser and an excitation wavelength of 325 nm. The morphology of Ag–SnS–TiO2 NBs and bare TiO2 NBs was evaluated using a JEOL JEM-2100 TEM at an accelerating voltage of 200 kV. Photoluminescence (PL) spectra were measured by a FP-6500 fluorescence spectrophotometer (JASCO, Japan) with an excitation wavelength of 325 nm. Powder XRD measurements were performed on a Bruker D8 Advance Powder X-ray diffractometer with CuKα (λ = 0.15406 nm). The resulting diffraction pattern was collected from 20° to 80° at a scan rate of 1.5° min−1. X-ray photoelectron spectroscopy (XPS) measurements were collected using a VG Scientific ESCALAB 250 instrument using an aluminum Kα X-ray radiation source (h = 1486.6 eV). For all scans, high resolution spectra were collected at a residence time of 0.1 s per point. The scans were measured by energy harvest at 50 eV and a separate sweep by energy at 20 eV.
3. Results and discussion
TEM and HRTEM were performed to elaborately observe the morphology change from pure TiO2 NBs to ternary Ag–SnS–TiO2 NBs (Fig. 2). Some ribbon-like TiO2 nanobelts with a length of several micrometers were clearly found in the low resolution TEM image (Fig. 2A). Measured from the high resolution TEM image (Fig. 2B), a lattice spacing of 0.351 was ascribed to the (101) planes of TiO2 crystal anatase phase.19 Recent investigation demonstrated that (101) facet of anatase crystals exhibited higher reactivity than (001) facet in photo-oxidation reactions for ˙OH radical generation.32 Fig. 2C and D showed representative TEM images of ternary Ag–SnS–TiO2 NBs, indicating the successful preparation of ternary nanocomposite. The nanocomposite consists of Ag and SnS nanoparticles with the diameters from several nanometers to over one hundred nanometers. The lattice fringes of Ag and SnS nanoparticles can be measured in the HRTEM image (Fig. 4D). The 0.224 nm and 0.293 nm lattice spacing were assigned to the (112) plane of Ag nanoparticle and (101) plane of SnS nanoparticle, respectively.19,33 Ag and SnS nanoparticles were firmly anchored on the surface of TiO2 NBs, where the hydrophilic groups serve as anchoring sites. In the nanocomposite, the coordination interaction of hydrophilic groups may hinder the nanoparticles from agglomeration and enable an even distribution on the TiO2 NBs, whilst the nanoparticles may serve as stabilizers to prevent the TiO2 NBs from aggregation.
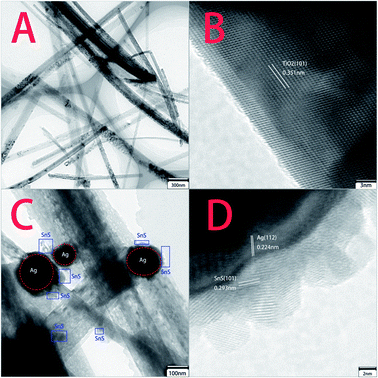 |
| Fig. 2 TEM and HRTEM images of pristine TiO2 NBs and ternary Ag–SnS–TiO2 NBs. (A and B) Pristine TiO2 NBs; (C and D) ternary Ag–SnS–TiO2 NBs. | |
The structural information and purity of Ag–SnS–TiO2 NBs were further characterized by XRD (Fig. 3). The diffraction peaks at 2θ = 25.3°, 37.7°, 48.0°, 53.8°, 54.9° and 62.6° were ascribed to the anatase phase of TiO2 (JCPDS, 71-1167). Meanwhile, the characteristic peaks at 2θ = 29.8° and 33.3° corresponded to the reduction phase (Ti9O17) of rutile phase (JCPDS 75-1751), indicating the interaction of SnS and the rutile phase.29 Besides, the diffraction peak of the SnS (101) crystal plane was found at 2θ = 31.5°, indicating the presence of SnS nanoparticles (JCPDS, 83-1758). The diffraction peak of the SnS (101) crystal plane at 2θ = 30.5° (JCPDS, 83-1758) may overlap with the diffraction peak of Ti9O17 at 2θ = 29.8°. The diffraction peak at 2θ = 37.8° assigned to the (112) plane of Ag nanoparticles have overlapped with the diffraction peak of (103) plane in the anatase phase of TiO2. However, the notable enhancement of shoulder peak indicated the in situ loading of Ag nanoparticles. Therefore, XRD spectra demonstrated the successful formation of ternary Ag–SnS–TiO2 NBs.
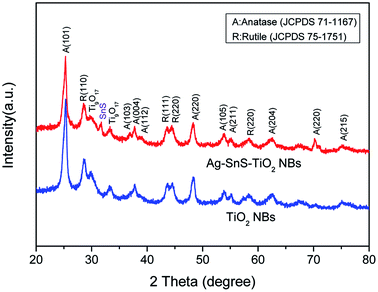 |
| Fig. 3 XRD spectra of ternary Ag–SnS–TiO2 NBs and pristine TiO2 NBs. | |
Raman spectroscopy provided further evidence for the ornaments of Ag and SnS nanoparticles on TiO2 NBs. As shown in Fig. 4, the strong scattering bands at 145 cm−1, 404 cm−1, 516 cm−1 and 635 cm−1 were ascribed to the anatase phase. Another two scattering bands at 229 cm−1 and 294 cm−1 were ascribed to the rutile phase, in good agreement with the XRD result. Strong scattering bands of the SnS (101) crystal plane were found at about 195 cm−1, indicating the presence of SnS nanoparticles. Notably, the strong scattering bands at 121 cm−1 and 248 cm−1 were attributed to the formation of chemical bonds among Ag, SnS and TiO2, lattice defects or disorders.34
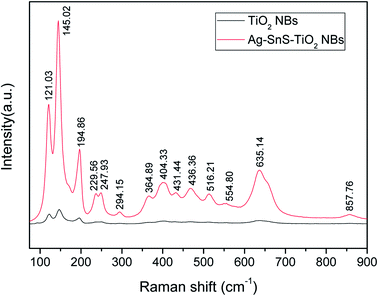 |
| Fig. 4 Raman spectra of ternary Ag–SnS–TiO2 NBs (red curve) and pristine TiO2 NBs (black curve). | |
XPS was also employed to analyze the information on the surface electronic state and the composition of ternary Ag–SnS–TiO2 NBs (Fig. 5). The fully scanned spectrum of ternary nanocomposite displayed in Fig. 5A, showing the presence of multiple elements in the TiO2 and Ag–SnS–TiO2 NBs. C signals in TiO2 and Ag–SnS–TiO2 NBs were attributed to carbon-based contaminants from the atmosphere or the vacuum system. The magnified XPS spectra exhibited elaborate surface binding energy (BE) of ternary Ag–SnS–TiO2 NBs (Fig. 5B–G). Two signals at 463.9 eV and 458.2 eV may stem from Ti 2p1/2 and Ti 2p3/2 (Fig. 5B), respectively, characteristic of TiO. The binding energy between Ti 2p1/2 and Ti 2p3/2 is 5.7 eV, which can be considered as the normal state of Ti4+ in Ag–SnS–TiO2 NBs. The signal of Ti 2p in Ag–SnS–TiO2 slightly shifted compared to that in TiO2 NBs, which can be ascribed to the formation of Ti9O17.29 Fig. 5C shows a high resolution XPS spectrum of Ag 3d in Ag–SnS–TiO2 NBs. Two signals at 373.7 eV and 486.6 eV corresponded to Ag 3d3/2 and Ag 3d1/2, respectively. The BE between Ag 3d3/2 and Ag 3d1/2 is 6.0 eV, indicating the formation of Ag nanoparticles in the nanocomposite.30 As shown in Fig. 5D, the signals at 495.0 eV and 486.6 eV were ascribed to Sn 3d3/2 and Sn 3d5/2, respectively. The BE between Sn 3d3/2 and Sn 3d5/2 is 8.4 eV, indicating the normal state of Sn2+ in the nanocomposite.35 As expected, the high-resolution XPS spectra of S 2p and O 1s in (Fig. 5E and F) revealed the presence of the SnS and TiO2. The interaction between Ti–O–Sn and O–Ag also indicated Ag and SnS particles here should covalently link to the surface of the TiO2 NBs.16,29 In contrast, the only two oxygen signals (529.4 eV and 531.0 eV) in Fig. 5G originated from the Ti–O–Ti and O–H, respectively.29 SnS and Ag content in the composites obtained from XPS analysis were 3.55% and 2.51%, respectively.
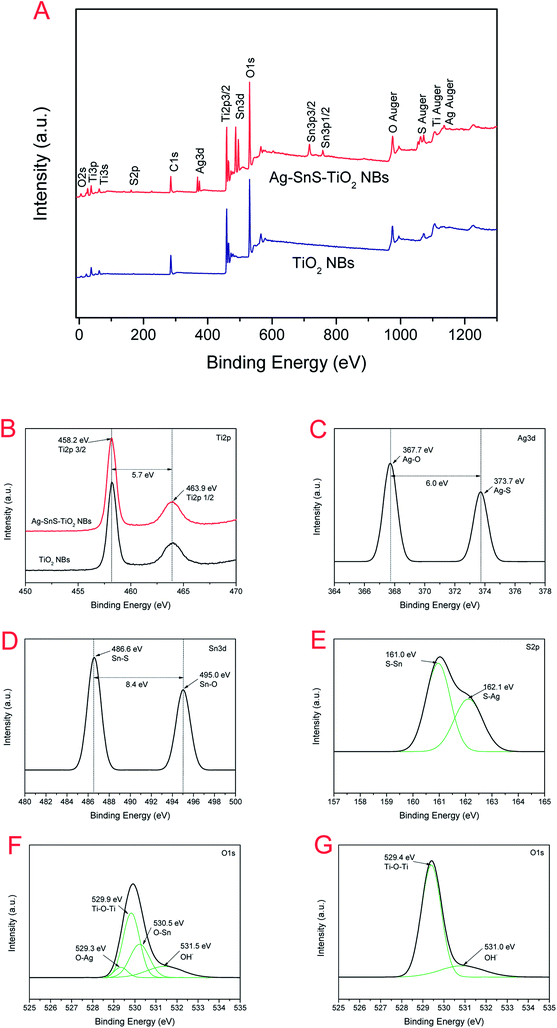 |
| Fig. 5 (A) Full scanning spectra of Ag–SnS–TiO2 NBs and TiO2 NBs; (B) Ti 2p fine XPS spectra of Ag–SnS–TiO2 NBs and TiO2 NBs; (C) Ag 3d fine XPS spectra of Ag–SnS–TiO2 NBs; (D) Sn 3d spectrum of Ag–SnS–TiO2 NBs; (E) S 2p fine XPS spectra of Ag–SnS–TiO2 NBs; (F) O 1s XPS spectra of Ag–SnS–TiO2 NBs; (G) O 1s Fine XPS spectra of TiO2 NBs. | |
The PC activities of commercial TiO2 (Degussa, P-25), pristine TiO2 NBs, Ag–TiO2 NBs, SnS–TiO2 NBs, and ternary Ag–SnS–TiO2 NBs were evaluated by simulating the photodegradation of simulated organic pollutants (MB and HRB) under sunlight. The degradation efficiency of simulated organic pollutants was defined as C/C0, where C and C0 represent the pollutants concentration at irradiation time t and pollutants adsorption–desorption equilibrium initial concentration. All the samples were sonicated in the dark for 10 min to get the pollutants adsorption–desorption equilibrium. The photodegradation curves of MB and HRB were shown in Fig. 6. Under the photo-induced degradation, the colored aqueous solution turned colorless with the time increase (the inset image of Fig. 6). Notably, the system with ternary Ag–SnS–TiO2 NBs exhibited the fastest photodegradation, compared to other control photocatalysts systems. In details, MB were fully degraded within 15 min under simulated sunlight irradiation, while Degussa P25 takes about 35 minutes to reach the same level (Fig. 6A). Similarly, Ag–SnS–TiO2 NBs also exhibited high PC activity against HRB (Fig. 6B) in 20 minutes. In summary, the PC activity of Ag–SnS–TiO2 NBs is several times faster than that of other control photocatalysts systems, owing to the synergetic effects of Ag and SnS nanoparticles. To investigate the stability of both material and performance of Ag–SnS–TiO2 NBs as a visible-light photocatalyst, we repeated the photocatalytic decolorization of MB and RHB five times and the corresponding results are shown in Fig. 6C and D. The as-prepared Ag–SnS–TiO2 NBs can maintain a stable and efficient photocatalytic performance after the first-cycle test, although the photocatalytic activity of repeated cycles was slight decreased compared with the first-cycle result.
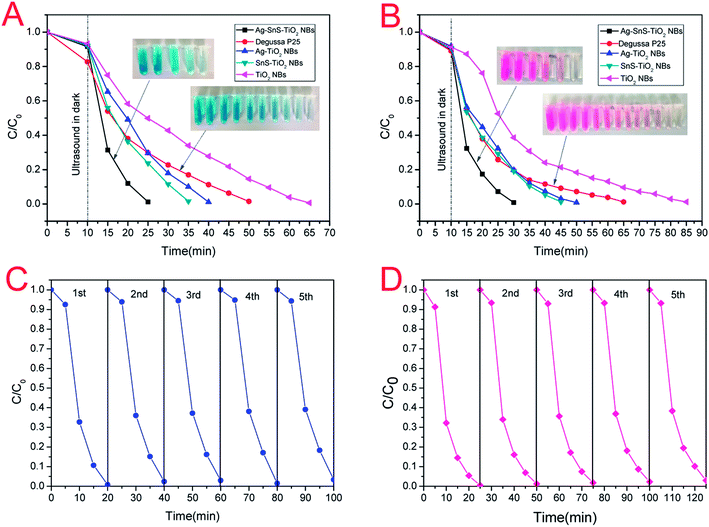 |
| Fig. 6 PC degradation curves of (A) MB and (B) HRB under simulated sunlight. The repeated photocatalytic decomposition of (C) MB and (D) HRB for the Ag–SnS–TiO2 NBs photocatalyst. | |
Fig. 7A displayed the UV-Vis absorption spectra of the prepared photocatalysts. The deposition of Ag and SnS nanoparticles significantly broadened the absorption rage of TiO2 NBs into the visible region, which may be attributed to the narrow band gap of SnS nanoparticles and the local surface plasmon resonance of Ag nanoparticles.15,19,21,36 Furthermore, Ag–SnS–TiO2 NBs exhibited the highest absorption intensity in the range of 350–800 nm due to the synergistic effect induced by SnS and Ag nanoparticles. Single nanoparticles loaded binary nanocomposites (SnS–TiO2 NBs and Ag–TiO2) NBs absorbed visible light much more strongly than unloaded TiO2 NBs and commercial Degussa P25 in the wavelength range of 400–750 nm, which was consistent with the previous literatures.6,37 It is obvious that the loading of SnS and Ag nanoparticles remarkably extended the absorption rage into the visible region, significantly improving the light efficiency utilization. The band gaps (Eg) of the synthesized photocatalysts were calculated from the UV-Vis absorption spectrum.38 The band gap energies of Ag–SnS–TiO2 NBs, SnS–TiO2 NBs, Ag–TiO2 NBs, Degussa P25 and TiO2 NBs determined by intercept were about 2.51, 2.82, 2.96, 3.08 and 3.11 eV (Fig. 7B), respectively. Unloaded TiO2 NBs and Degussa P25 possessed the highest band gaps, in the rage from 3.0 eV to 3.2 eV. The high band gaps were probably due to the presence of rutile phase (3.0 eV) and anatase phase (3.2 eV) in TiO2 NBs, indirectly consistent with XRD results. Remarkable decrease of the corresponding band gap energy value was attributed to the coupling of Ag and SnS nanoparticles with TiO2 NBs. The direct band gap of Ag–SnS–TiO2 NBs, SnS–TiO2 NBs, Ag–TiO2 NBs, P25 and TiO2 NBs was 3.04, 3.08, 3.11, 3.14 and 3.17 eV (Fig. 7C), respectively. There were no significant differences in the value of direct band gap between the groups. It indicates that the improvement in visible-light absorption can be ascribed to the significant decrease of indirect band gap. A Schottky junction formed between Ag nanoparticles and TiO2 NBs resulted in electrons transferring from the excited TiO2 NBs to the Ag nanoparticles.19 Consequently, the electron accumulation induced the Fermi level of Ag nanoparticles shift to the conduction band of TiO2 NBs, further diminishing the band gap energy of the nanocomposites.19 Besides, the introduction of narrow-banded SnS nanoparticles could also reduce the band gap of modified TiO2 NBs. The similar decrease on SnS–TiO2 heterostructures was also reported by Masahiro Miyauchi et al.39
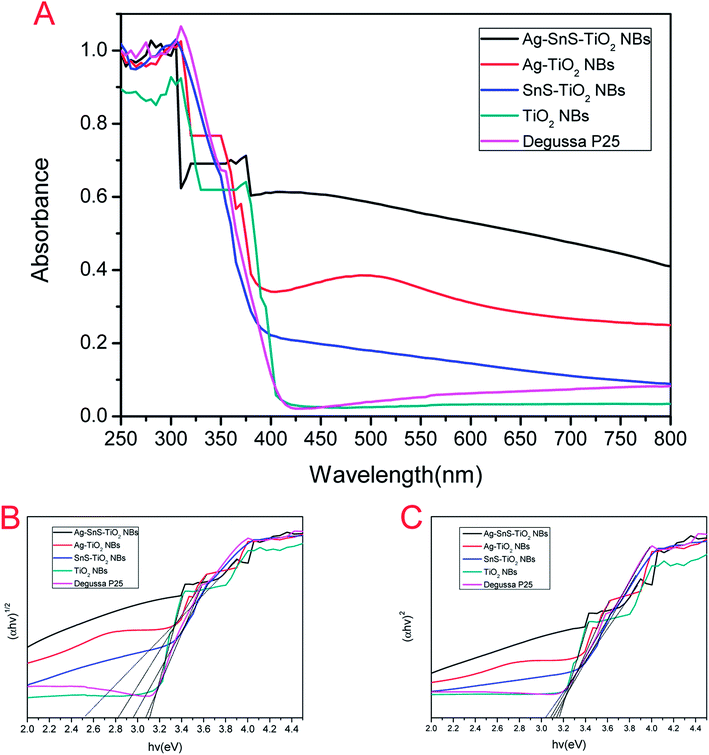 |
| Fig. 7 (A) UV-Vis absorption spectra of TiO2 NBs, Degussa P25, Ag–TiO2 NBs, SnS–TiO2 NBs and Ag–SnS–TiO2 NBs. (B) (αhν)1/2 vs. hν for TiO2 NBs, Degussa P25, Ag–TiO2 NBs, SnS–TiO2 NBs and Ag–SnS–TiO2 NBs. (C) (αhν)2 vs. hν for TiO2 NBs, Degussa P25, Ag–TiO2 NBs, SnS–TiO2 NBs and Ag–SnS–TiO2 NBs. | |
PL emission spectra were performed to characterize the efficiency of charge carrier trapping, transfer, and separation in photocatalysts. As shown in Fig. 8, all the photocatalysts emitted strong signals at 421, 451, 469, 484 and 492 nm. The high-energy peak at 421 nm may be associated with band edge excitation of TiO2, while the lower energy peaks at 451, 469, 484 and 492 nm were attributed to the oxygen vacancies forming at the surface of Ag–SnS–TiO2 NBs.40–42 It is generally believed that PL intensity is inverse related with efficiency of charge carrier separation.40 Based on our tests, the PL intensity order was as follow: Ag–SnS–TiO2 NBs < Ag–TiO2 NBs < SnS–TiO2 NBs < Degussa P25 < TiO2 NBs. Therefore, ternary Ag–SnS–TiO2 NBs exhibited the lowest recombination rate of photoinduced electrons (e−) and holes (h+).
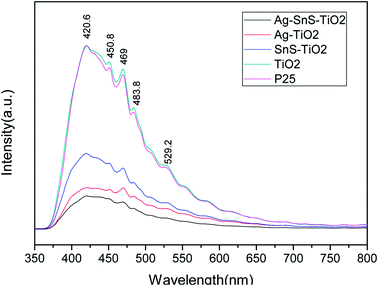 |
| Fig. 8 Photoluminescence spectra of TiO2 NBs, Degussa P25, Ag–TiO2 NBs, SnS–TiO2 NBs and Ag–SnS–TiO2 NBs. | |
To explore the impact of electron (e−) and hole (h+) on photodegradation, a comparative experiment were performed as shown in Fig. 9. In our experiment, ethanol and CCl4 were utilized as hole-trapping and electron-trapping agents, respectively. With the addition of ethanol, the photodegradation efficiency of ternary Ag–SnS–TiO2 NBs decreased gradually; therein, only half of the MB is degraded in 50 min (Fig. 9A). However, with the addition of CCl4, photodegradation efficiency happened more quickly; MB was completely degraded in 10 min. Similar results for RHB photodegradation in Fig. 9B were consistent with that for MB. The comparative experiment demonstrated that hole (h+) played a dominant role in the photodegradation of MB and RHB by Ag–SnS–TiO2 NBs.36 Besides, the interaction between Ag–SnS–TiO2 NB heterostructures functions as an “electron capture agent” to accelerate the photodegradation, similar to CCl4 in comparative experiments.
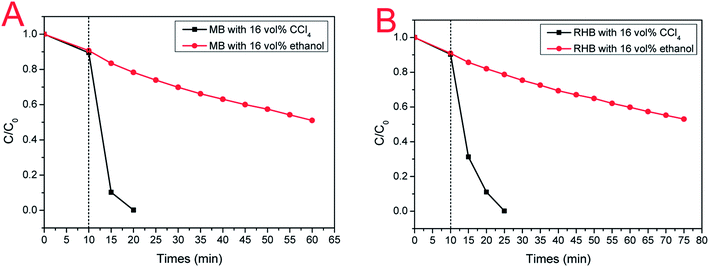 |
| Fig. 9 (A) Photodegradation curves of MB with electron (e−) or hole (h+) capture agents; (B) Photodegradation curves of RHB with electron (e−) or hole (h+) capture agents. Black curve: 20 mL ethanol was added to 100 mL of aqueous pollutant solution; red curve: 20 mL CCl4 was added to 100 mL of aqueous pollutant solution. | |
We proposed a possible reaction mechanism of MB/RHB photodegradation on ternary Ag–SnS–TiO2 NBs (Fig. 10). Upon exposure to the simulated sunlight, electron–hole pairs are generated in the two excitation semiconductors, TiO2 and SnS. Three possible ways for electron transfer are proposed in this system. First, due to the potential difference between the conduction band of the TiO2 NBs and the Fermi level of the Ag nanoparticle, the excited electrons can transfer from the conduction band of the TiO2 band to the Ag nanoparticles.19 Second, since the edge of the conduction band of SnS nanoparticle is more negative than that of TiO2, the mobilizing electrons tend to move from the conduction band of SnS nanoparticle to the conduction band of TiO2 NBs.39 Third, due to the large difference in work function between Ag and SnS, photogenerated electrons are directly implanted into Ag nanoparticles from the conduction band of SnS nanoparticle.43 Therefore, the ternary Ag–SnS–TiO2 NBs can effectively separate photogenerated electron–hole pairs and delay the carrier recombination. The captured electrons on the Ag nanoparticles can be effectively transferred to the adsorbed oxygen molecules to form superoxide radicals (O2−), which can be further converted into hydrogen peroxide (H2O2) by reacting with two protons. Meanwhile, the left holes can be reduced by contaminants or rapidly consumed by adsorbed hydroxyl ions or water molecules, generating hydroxyl radicals (·OH). As very strong oxidants, these actives oxidize organic molecules to inorganic compounds that are harmless to the environment.4,6,44 Therefore, we can conclude that the complex Ag–SnS–TiO2 structure promotes the formation of active species during the PC reaction, resulting in high PC activity. The PC reaction mechanism of Ag–SnS–TiO2 NBs for decomposition of MB/RHB can be summarized as follows:
MB/RHB + O2−/H2O2/·OH/h+ → H2O + CO2 |
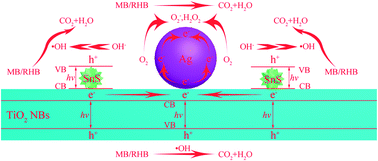 |
| Fig. 10 Schematic representation of photocatalysis mechanism. | |
4. Conclusion
In conclusion, new ternary Ag–SnS–TiO2 NBs composites have been successfully fabricated from natural assembly by using TiO2 nanobelts as templates. Optical absorption, PL and PC activity measurements show that the incorporation of Ag and SnS nanoparticles not only broadens the light absorption rage of TiO2 NBs into the visible region, but also enhances the PC degradation by improving charge transfer through interfacial charge transfer. The PC activity of Ag–SnS–TiO2 NBs photocatalysts is about 2–3 times than that of commercial P25. We hope this work will pave the way for further developing TiO2-based photocatalysts with high the solar energy conversion efficiency.
Conflicts of interest
There are no conflicts to declare.
Acknowledgements
This work was financially supported by National Natural Science Foundation of China (No. 21376199 and 51002128), Natural Science Foundation of Hunan Province (No. 2018JJ2393), Scientific Research Foundation of Hunan Provincial Education Department (No. 17A205) and Postgraduate Innovation Foundation of Hunan Province (No. CX2016B269).
References
- O. Edenhofer, K. Seyboth, F. Creutzig and S. Schlömer, Annu. Rev. Environ. Resour., 2013, 38, 169–200 CrossRef.
- D. Mitoraj and H. Kisch, Angew. Chem., Int. Ed., 2008, 47, 9975–9978 CrossRef CAS PubMed.
- Y. Xia, P. Yang, Y. Sun, Y. Wu, B. Mayers, B. Gates, Y. Yin, F. Kim and H. Yan, Adv. Mater., 2003, 15, 353–389 CrossRef CAS.
- R. Asahi, T. Morikawa, H. Irie and T. Ohwaki, Chem. Rev., 2014, 114, 9824–9852 CrossRef CAS PubMed.
- L. Wang and T. Sasaki, Chem. Rev., 2014, 114, 9455–9486 CrossRef CAS PubMed.
- J. Schneider, M. Matsuoka, M. Takeuchi, J. Zhang, Y. Horiuchi, M. Anpo and D. W. Bahnemann, Chem. Rev., 2014, 114, 9919–9986 CrossRef CAS PubMed.
- Q. Wang, J. He, Y. Shi, S. Zhang, T. Niu, H. She, Y. Bi and Z. Lei, Appl. Catal., B, 2017, 214, 158–167 CrossRef CAS.
- S. Sun, P. Gao, Y. Yang, P. Yang, Y. Chen and Y. Wang, ACS Appl. Mater. Interfaces, 2016, 8, 18126–18131 CAS.
- D. Yang, Y. Sun, Z. Tong, Y. Tian, Y. Li and Z. Jiang, J. Phys. Chem. C, 2015, 119, 5827–5835 CAS.
- N. Zhang, Y. Zhang, X. Pan, M.-Q. Yang and Y.-J. Xu, J. Phys. Chem. C, 2012, 116, 18023–18031 CAS.
- Y. Lixia, L. Shenglian, L. Yue, X. Yan, K. Qing and C. Qingyun, Environ. Sci. Technol., 2010, 44, 7641–7646 CrossRef PubMed.
- Q. Wang, T. Niu, L. Wang, C. Yan, J. Huang, J. He, H. She, B. Su and Y. Bi, Chem. Eng. J., 2017, 337, 506–514 CrossRef.
- Y. Chen, G. Zhu, M. Hojamberdiev, J. Gao, R. Zhu, C. Wang, X. Wei and P. Liu, J. Hazard. Mater., 2018, 344, 42–54 CrossRef CAS PubMed.
- H. Li, J. Ji, X. Zheng, Y. Ma, Z. Jin and H. Ji, Mater. Sci. Semicond. Process., 2015, 36, 65–70 CrossRef CAS.
- A. Umar, M. S. Akhtar, R. I. Badran, M. Abaker, S. H. Kim, A. Al-Hajry and S. Baskoutas, Appl. Phys. Lett., 2013, 103, 101602 CrossRef.
- Y. Jia, F. Yang, F. Cai, C. Cheng and Y. Zhao, Electron. Mater. Lett., 2013, 9, 287–291 CrossRef CAS.
- J. Fang, L. Xu, Z. Zhang, Y. Yuan, S. Cao, Z. Wang, L. Yin, Y. Liao and C. Xue, ACS Appl. Mater. Interfaces, 2013, 5, 8088–8092 CAS.
- N. Roy, K. T. Leung and D. Pradhan, J. Phys. Chem. C, 2015, 119, 19117–19125 CAS.
- Y. Yang, J. Wen, J. Wei, R. Xiong, J. Shi and C. Pan, ACS Appl. Mater. Interfaces, 2013, 5, 6201–6207 CAS.
- M. Maicu, M. C. Hidalgo, G. Colón and J. A. Navío, J. Photochem. Photobiol., A, 2011, 217, 275–283 CrossRef CAS.
- S. T. Kochuveedu, D.-P. Kim and D. H. Kim, J. Phys. Chem. C, 2012, 116, 2500–2506 CAS.
- A. A. Ismail, D. W. Bahnemann and S. A. Al-Sayari, Appl. Catal., A, 2012, 431–432, 62–68 CrossRef CAS.
- W. Zhou, G. Du, P. Hu, Y. Yin, J. Li, J. Yu, G. Wang, J. Wang, H. Liu, J. Wang and H. Zhang, J. Hazard. Mater., 2011, 197, 19–25 CrossRef CAS PubMed.
- Q. Lu, Z. Lu, Y. Lu, L. Lv, Y. Ning, H. Yu, Y. Hou and Y. Yin, Nano Lett., 2013, 13, 5698–5702 CrossRef CAS PubMed.
- Z. Yang, P. Zhang, Y. Ding, Y. Jiang, Z. Long and W. Dai, Mater. Res. Bull., 2011, 41, 1625–1631 CrossRef.
- Q. Wang, J. He, Y. Shi, S. Zhang, T. Niu, H. She and Y. Bi, Chem. Eng. J., 2017, 326, 411–418 CrossRef CAS.
- K. G. Deepa and J. Nagaraju, Mater. Sci. Semicond. Process., 2014, 27, 649–653 CrossRef CAS.
- H. Tsukigase, Y. Suzuki, M.-H. Berger, T. Sagawa and S. Yoshikawa, J. Nanosci. Nanotechnol., 2011, 11, 1914–1922 CrossRef CAS PubMed.
- Z. Yang, Y. Jiang, Q. Yu, Y. Ding, Y. Jiang, J. Yin and P. Zhang, J. Mater. Sci., 2017, 52, 13586–13595 CrossRef CAS.
- Y. Chen, W. Huang, D. He, Y. Situ and H. Huang, ACS Appl. Mater. Interfaces, 2014, 6, 14405–14414 CAS.
- X. Zhang, J. Liu and E. M. J. Johansson, Nanoscale, 2015, 7, 1454–1462 RSC.
- J. Pan, G. Liu, G. Q. Lu and H.-M. Cheng, Angew. Chem., Int. Ed., 2011, 50, 2133–2137 CrossRef CAS PubMed.
- J. Ning, K. Men, G. Xiao, L. Wang, Q. Dai, B. Zou, B. Liu and G. Zou, Nanoscale, 2010, 2, 1699–1703 RSC.
- I. Kosackia, T. Suzuki, H. U. Anderson and P. Colomban, Solid State Ionics, 2002, 149, 99–105 Search PubMed.
- H. Hu, B. Yang, J. Zeng and Y. Qian, Mater. Chem. Phys., 2004, 86, 233–237 CrossRef CAS.
- Y. Liu, G. Zhu, J. Gao, M. Hojamberdiev, R. Zhu, X. Wei, Q. Guo and P. Liu, Appl. Catal., B, 2017, 200, 72–82 CrossRef CAS.
- Q. Chen, H. Liu, Y. Xin and X. Cheng, Chem. Eng. J., 2014, 241, 145–154 CrossRef CAS.
- Q. Zhang, L. Wang, J. Feng, H. Xu and W. Yan, Phys. Chem. Chem. Phys., 2014, 16, 23431–23439 RSC.
- M. Miyauchi, Chem. Phys. Lett., 2011, 514, 151–155 CrossRef CAS.
- Z. Wu, Y. Wang, L. Sun, Y. Mao, M. Wang and C. Lin, J. Mater. Chem. A, 2014, 2, 8223 CAS.
- K. Li, B. Chai, T. Peng, J. Mao and L. Zan, ACS Catal., 2013, 3, 170–177 CrossRef CAS.
- M. Nasir, Z. Xi, M. Xing, J. Zhang, F. Chen, B. Tian and S. Bagwasi, J. Phys. Chem. C, 2013, 117, 9520–9528 CAS.
- K. S. Kumar, A. G. Manohari, S. Dhanapandian and T. Mahalingam, Mater. Lett., 2014, 131, 167–170 CrossRef CAS.
- Y. Ma, X. Wang, Y. Jia, X. Chen, H. Han and C. Li, Chem. Rev., 2014, 114, 9987–10043 CrossRef CAS PubMed.
Footnote |
† The first two authors contributed equally to this work. |
|
This journal is © The Royal Society of Chemistry 2018 |