DOI:
10.1039/C8RA00600H
(Paper)
RSC Adv., 2018,
8, 13865-13870
On peroxymonosulfate-based treatment of saline wastewater: when phosphate and chloride co-exist
Received
20th January 2018
, Accepted 3rd April 2018
First published on 13th April 2018
Abstract
Both chloride and phosphate are common inorganic anions in industrial wastewater, however, their effects on peroxymonosulfate (PMS)-based oxidation systems are largely unknown. The present results show that addition of chloride (>1 mM) apparently enhanced the degradation of Acid Orange 7 (AO7) independent of the presence of phosphate (PBS) buffer. Both PBS and chloride favored the degradation of AO7, while PBS played a more important role when they co-existed. The degradation efficiency of AO7 was enhanced by increasing the concentration of PBS and chloride. A maximum of absorbable organic halides (AOX) accumulation was observed; indicating some chlorinated byproducts could be initially generated and further oxidized by increasing the reaction time. It is demonstrated that the PBS/PMS system, with a lower AOX formation at the same chloride concentration, is superior to the Co/PMS system, a typical sulfate radical-based system. The active chlorine species (HClO/Cl2) were found to be the dominant oxidants in the presence of higher chloride concentration (>50 mM) under neutral conditions. The findings of this work may promote the further application of PMS-based oxidation processes in saline effluents treatment.
1. Introduction
Approximately 10–20% of the total production of dyes released from textile and other industrial activities have caused increasing environmental hazards owing to their non-biodegradability, toxicity and potential carcinogenicity.1 As one of common dyes, the azo dye presenting in the wastewater could result in lower water absorbance and eutrophication, and thus has gained great attention in wastewater treatment.2 Because conventional treatment processes, such as adsorption, flocculation and sludge treatment have not achieved satisfactory efficiency in removing dyes,3,4 more efficient purification processes are required. Recently, increasing attention has been paid to the application of sulfate radicals (SO4˙−) based advanced oxidation processes (AOPs) in eliminating refractory organic pollutants or remediating contaminated groundwater and soil, due to their selective oxidation capacity and excellent treatment performance.5–8 In general, SO4˙− with a high redox potential (2.5–3.1 V versus NHE) can be generated from activation of peroxymonosulfate (PMS) and persulfate (PS) by various methods, such as UV,7,9 ultrasonic,10,11 transition metals,12,13 base14 and carbon materials.15,16 For example, the degradation efficiency of atrazine by cobalt/peroxymonosulfate process was better than that of Fenton's reagent.6
In the actual water matrix, the common component Cl− could scavenge SO4˙− and HO˙ to form less reactive species (e.g., Cl2˙− and Cl˙)17 and thus degradation efficiency of pollutants would be restrained by the presence of chloride.18,19 More recently, several investigations have reported the direct reaction between PMS and chloride could lead to the formation of active chlorine species (Cl2/HClO), which are capable of degrading pollutants.20,21 An enhancement of dye decolorization was observed in PMS/Cl− system as compared with that in the presence of PMS alone.22 Grebel et al.23 found that the degradation of phenol was markedly enhanced under a high concentration of chloride by PMS/Cl− process. However, our previous studies indicate that absorbable organic halides (AOX) was produced and the formation of AOX increased with chloride concentration increasing,24,25 owing to the generation of poly-chlorinated byproducts in PMS-based oxidation system.22,26 AOX formation and accumulation is a matter of great concern due to their potential toxicity.27 Fang et al.24 investigated the inhibitory effect of chlorinated byproducts on bioluminescence during the treatment of 0.2 mM 2,4,6-trichlophenol (TCP) using a Co/PMS/Cl− process. An increase in AOX was observed while the corresponding inhibition varied from 60% to 86% after 180 min reaction time, indicating the adverse impact of AOX. Yuan et al.22 demonstrated that AOX value was 2.2 mg L−1 at the application of 0.2 mM chloride while it rose to 3.5 mg L−1 at the application of 100 mM chloride at a reaction time of 120 min in Co/PMS system.
In addition to a high concentration of chloride, phosphate is also one of the most common phosphoric components in the industrial wastewater. Large amounts of phosphate are often released from industrial activities, such as leather tanning, chemical fertilizer production and refractory manufacture,28–30 leading to phosphate pollution in the wastewater. However, phosphate may affect the efficiency of AOPs during the treatment.31,32 Recently, it is reported that PMS could be activated by phosphate to degrade pollutants.33 Lou et al.34 reported 15.7% of 0.2 mM AO7 and 37.7% of 0.25 mM TCP were completely mineralized, respectively while 2.5 mM PMS and 0.1 mM PBS were applied in a PBS/PMS process. Besides, nearly 100% of 0.05 mM dye was efficiently degraded with the addition of 2.5 mM PMS and 0.1 M pyrophosphate in a PMS-based process.35 In practice, the efficiency of AOPs was always constrained by the presence of various ions. To the best of our knowledge, effect of the single ion on contaminant decomposition based on SO4˙− has been investigated extensively, while effect of co-existing ions still remains obscure.
In this work, we investigated the effect of both phosphate and chloride in a PMS-based process. The influences of chloride concentration, PBS dosage, and pH (3.0 to 8.0) on the degradation of Acid Orange 7 (AO7) have been examined. In order to assess the effect of chloride on the formation of chlorinated compounds, AOX determination was carried out. In addition, quenching experiments were conducted to identify active species during the treatment of wastewater. This work may provide a helpful reference for disposal of saline wastewater.
2. Experimental
2.1. Materials
Oxone® ([2KHSO5·KHSO4·K2SO4] salt, 95%) and Acid Orange 7 (AO7, pure) were supplied by Sigma-Aldrich. For the preparation of phosphate buffer solution, dipotassium hydrogen phosphate (K2HPO4·3H2O), potassium dihydrogen phosphate (KH2PO4) and phosphoric acid (H3PO4) were mixed proportionally and adjusted to different pH values. The concentration of PBS was counted by the content of phosphate anions. KH2PO4, K2HPO4·3H2O, (NH4)2SO4 and tert-butyl alcohol (TBA) were all purchased from Sinopharm Chemical Reagent Co., Ltd. China and used without further purification. Methanol (MeOH, HPLC grade) was purchased from CNW Technologies GmbH. Barnstead Ultra-Pure water (>18.2 MΩ cm) was used throughout the study.
2.2. Experimental procedures
All reactions were initiated in 10 mL beakers by mixing appropriate concentration of phosphate buffer, AO7, chloride, and PMS at ambient temperature. The initial pH did not change by more than 0.2 pH units during the experiments. The degradation of AO7 was monitored immediately at 484 nm in time scan mode on an Epscord200 PLUS spectrophotometer. The optimal dosage of PMS was selected according to the preliminary results. AOX determinations were carried out by quantitative analysis (AOX, multi X® 2500, Jena Germany) after enrichment on activated carbon at 950 °C. TOC analyzer (multi N/C® 3100) was used to measure the TOC. The identification of active species was performed by quenching experiments. These experiments were conducted in duplicate and the average data with their standard deviations were displayed.
3. Results and discussion
3.1. Effect of Cl− concentration
The degradation of AO7 followed the pseudo first-order kinetic model under the different concentration of Cl−:36 |
C = C0 exp(−kt)
| (1) |
where C0 is the concentration of AO7 at the time t = 0 and t, respectively; k is the observed degradation rate constant, which could be estimated from the slopes of the lines in the plots of −ln(C/C0) versus reaction time. As shown in Fig. 1, the reaction rate constants were 0.32 × 10−2, 0.59 × 10−2, 1.61 × 10−2 and 1.75 × 10−2 s−1 corresponding to 1, 50, 200, 300 mM chloride, respectively (R2 > 0.95). The kinetics data indicate that PBS/PMS oxidation was influenced by chloride. Notably, a dual effect was found on AO7 degradation which is similar to our previous investigations.22,37 At a low level of chloride (<1 mM), the degradation rate of AO7 declined slightly. However, further addition of chloride (>1 mM) apparently accelerated dye degradation. It is supposed that chloride directly reacted with PMS to form active chlorine species for dye degradation at a high chloride concentration.21 As the concentration of chloride continually increased from 10 to 300 mM, the degradation efficiency of AO7 was enhanced significantly. These results demonstrate the favorable effect of chloride on the PMS-based process. For example, the rate constant of AO7 degradation was about 0.85 × 10−2 s−1 with the presence of 100 mM chloride, higher than that without chloride (k = 0.54 × 10−2 s−1).
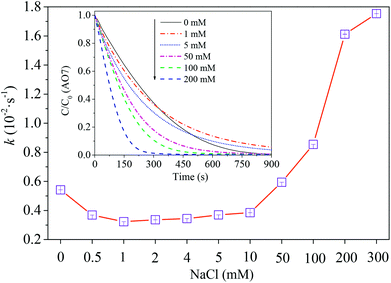 |
| Fig. 1 Effect of chloride concentration on AO7 degradation rate constants. Conditions: [AO7] = 0.06 mM, [PMS] = 2.5 mM, [PBS] = 0.12 M, pH = 7.0. | |
3.2. Effect of PBS dosage
The effect of ionic strength on the degradation rate of rhodamine B was demonstrated by Lou.34 As one kind of common inorganic compounds, PBS might have a similar effect on AO7 degradation. Additional tests were carried out at various PBS concentration. As shown in Fig. 2, an enhanced degradation of AO7 was observed with the increase of PBS. This indicates that PMS could be activated by PBS alone (in the absence of chloride) which was in common with the previous study.38 As the concentration of PBS varied from 0 to 0.12 M, the rate constant of AO7 degradation increased from 0 to 0.54 × 10−2 s−1. Similar to transition metals, the higher PBS concentration led to the higher degradation efficiency of AO7.39 The degradation of AO7 in the presence of chloride was also performed. At a low concentration of chloride (<50 mM), the addition of chloride did not affect the degradation profiles, implying the dominant role of PMS activation with PBS. When chloride concentration was up to 300 mM, however, the degradation rate constant of AO7 increased to 0.63 × 10−2 s−1 at [PBS]0 = 0 mM, which should be caused by the reaction of PMS with chloride. Increasing the concentration of PBS apparently resulted in much more AO7 degradation. The degradation rate constant of AO7 increased to 1.72 × 10−2 s−1 with the presence of 0.12 M PBS. This result unambiguously confirms that PBS rather than chloride played a key role in the degradation of AO7 in a PMS-based process and the effect of ionic strength on the reaction rate. The positive impact of PBS dosage on the degradation indicates the suitable application of PMS in treating phosphate abundant wastewater.
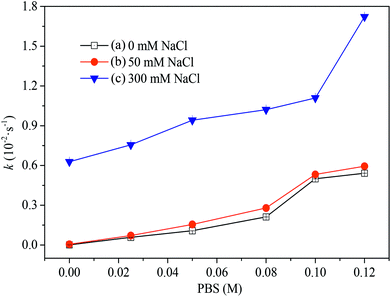 |
| Fig. 2 Effect of PBS concentration on AO7 degradation rate constants. Conditions: [AO7] = 0.06 mM, [PMS] = 2.5 mM, pH = 7.0. (a) [NaCl] = 0 mM. (b) [NaCl] = 50 mM. (c) [NaCl] = 300 mM. | |
3.3. Effect of pH
It is reported that the pH would strongly influence the degradation efficiency of pollutants in a PMS-based process.40 Therefore, effect of pH on AO7 degradation were further examined by virtue of the buffer effect of phosphate-based solution. Fig. 3 depicts the degradation rate of AO7 was evidently enhanced with the increase of pH in the absence of chloride. PMS is stable at pH < 4.0, while it becomes unstable at pH > 7.0.41 Thus, faster degradation rates of dye were expected under neutral or alkaline conditions. It should be noted that the addition of chloride could enhance AO7 degradation in the examined pH of 3.0–8.0. Under the acidic conditions (pH < 4.0), chloride is directly oxidized via a two-electron process (eqn (2) and (3)), while the contribution of the reaction between PBS and PMS should be negligible. A further increase of pH to 6.0 resulted in a significant decrease in degradation rate. The decomposition of HClO (1.49 V versus NHE) to ClO− with lower oxidation potential (0.94 V versus NHE) led to lower dye degradation efficiency.42 As the pH increased from 7.0 to 8.0, degradation efficiency of AO7 was enhanced significantly. For example, AO7 degradation rate increased from 0.761 × 10−2 to 1.621 × 10−2 s−1 when chloride concentration varied from 100 to 200 mM chloride at pH 7.0. The oxidation capacity of the direct reaction between PMS and chloride declined, whereas the reaction between PBS and PMS rose remarkably in the examined pH of 7.0–8.0. When pH was 7.0–8.0, the reaction between PMS and PBS governed the overall degradation reaction and a favorable effect on dye degradation were found. Hence, under the various PBS/PMS reaction condition, a higher AO7 degradation efficiency was observed in neutral solution. The result was in good with the previous report by Lou.35 |
2Cl− + HSO5− + H+ → SO42− + Cl2 + H2O
| (2) |
|
Cl− + HSO5− → SO42− + HClO
| (3) |
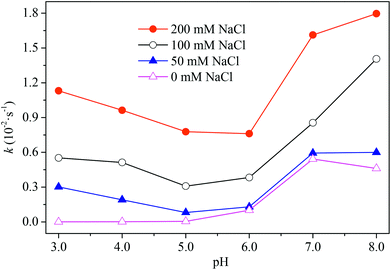 |
| Fig. 3 Effect of pH on AO7 degradation rate constants in the presence of chloride. Conditions: [AO7] = 0.06 mM, [PMS] = 2.5 mM, [PBS] = 0.12 M. | |
3.4. AOX formation
The coexistence of both chloride and phosphate apparently favored PMS-based process for efficient dye degradation. However, AOX accumulation occurs if chloride is oxidized to reactive chlorine species or radicals (such as Cl˙ and Cl2˙−) which further chlorinate organic compounds.22 Thus, it is necessary to quantify AOX level during the reaction in the presence of chloride. Fig. 4 compares AOX values under different chloride concentration (5 mM, 50 mM). Measurable AOX was not observed at t = 0 min. At a low concentration of chloride (5 mM), an enhanced AOX formation was observed and AOX declined after 15 h. At a higher level of chloride (50 mM), a significant AOX accumulation happened, while a decay of AOX was observed after 3 h. Increasing chloride concentration resulted in higher AOX values, in corresponding with the previous studies.22,24 The decrease of AOX implies that some chlorinated byproducts could be mineralized after a long reaction time. In addition, it should be much difficult to eliminate overall AOX at a higher chloride concentration due to the simultaneous occurrence of dechlorination and re-chlorination.25
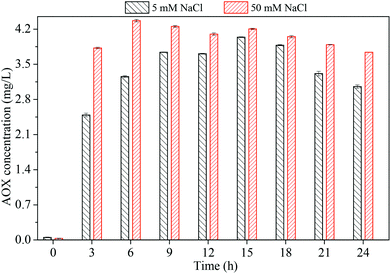 |
| Fig. 4 AOX formation in the PBS/PMS system as a function of time. Conditions: [AO7] = 0.2 mM, [PBS] = 0.12 M, [PMS] = 8 mM. | |
Co/PMS system, a common system to produce sulfate radicals, was compared with the PBS/PMS system on AOX formation. Fig. 5 shows the overall AOX formation and reduction in the Co/PMS/Cl− system. When chloride concentration varied from 5 mM to 50 mM, the enhanced AOX values was also observed. The maximum AOX values in a PBS/PMS system were 4.04 mg L−1 and 4.37 mg L−1, respectively, while the corresponding values increased dramatically from 9.17 mg L−1 and 10.19 mg L−1 in the Co/PMS system, respectively. A similar phenomenon was reported during the treatment of 2,4,6-trichlophenol in a Co/PMS/Cl− system.18 This comparison in AOX formation demonstrates that chloride in the PBS/PMS system cannot be effectively activated than in the Co/PMS process. It is suggested that PBS/PMS process is promising for industrial wastewater treatment due to the less formation of AOX.
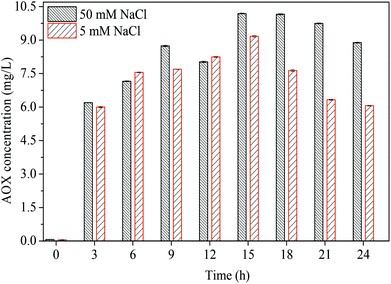 |
| Fig. 5 AOX values of AO7 degradation in the Co/PMS system as a function of reaction time. Conditions: [AO7] = 0.2 mM, [Co2+] = 0.1 mM, [PMS] = 10 mM. | |
3.5. TOC removal
The mineralization of AO7 in the Co/PMS system and PBS/PMS system was examined by varying the initial content of chloride ion, respectively. Fig. 6 and 7 show that removal rates of TOC increased over the reaction time in these two systems. Increasing the concentration of chloride was favorable to TOC removal in a PBS/PMS process, but apparently decreased the extent of AO7 mineralization in a Co/PMS process, in good agreement with our previous observation.22 The contrasting TOC results demonstrate the difference in reaction mechanism between Co/PMS and PBS/PMS processes.
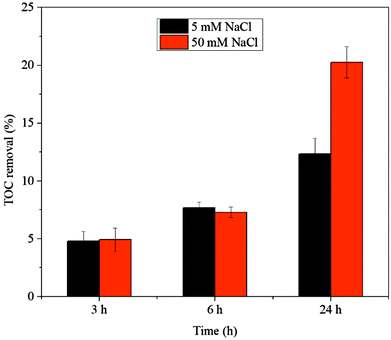 |
| Fig. 6 TOC removal of AO7 in the PBS/PMS system as a function of reaction time. Conditions: [AO7] = 0.2 mM, [PBS] = 0.12 M, [PMS] = 8 mM. | |
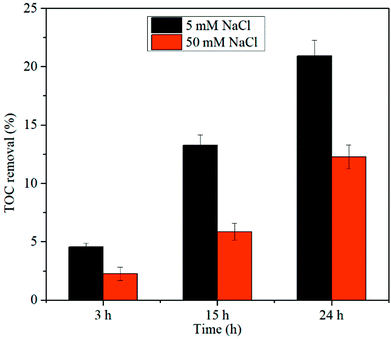 |
| Fig. 7 TOC removal of AO7 in the Co/PMS system as a function of reaction time. Conditions: [AO7] = 0.2 mM, [Co2+] = 0.1 mM, [PMS] = 10 mM. | |
3.6. Identification of the reactive species
Generally, HO˙ can be generated in the SO4˙−-based process due to the reaction of SO4˙− with hydroxide (7.3 × 107 M−1 s−1) and water (103 to 104 s−1) under neutral conditions.43,44 The organic pollutant can be decomposed by both SO4˙− and HO˙. Both methanol and tert-butyl alcohol were selected as scavengers due to the reactivity of methanol toward SO4˙− (7.8 × 106 M−1 s−1)45 and HO˙ (9.7 × 108 M−1 s−1)46 and the reactivity of tert-butyl alcohol toward HO˙ ((3.6 to 7.8) × 108 M−1 s−1).47 As shown in Fig. 8, in the absence of chloride, the degradation of AO7 was successfully inhibited after adding methanol and a similar inhibition was observed with the addition of TBA. The coexistence of both SO4˙− and HO˙ may be responsible for AO7 degradation. However, chloride may mitigate the inhibitory effect of two kinds of scavengers. At low concentration of chloride (<50 mM), degradation rates of AO7 increased slightly. As chloride concentration increased from 50 to 300 mM, however, the enhanced degradation of AO7 was observed. The failure of scavengers to inhibit AO7 degradation suggests that other active species, instead of both SO4˙− and HO˙, contributed to the degradation of AO7. The high level of chloride (>50 mM) resulted in a high concentration of HClO/Cl2 which are responsible for pollutant degradation. Thus, HClO/Cl2 might play a key role in AO7 oxidation at the higher chloride concentration (>50 mM).
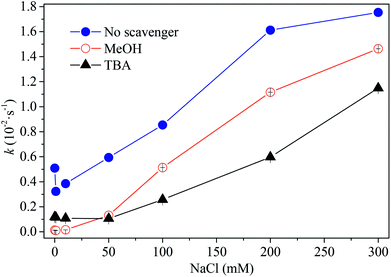 |
| Fig. 8 Effect of radical scavengers on dye degradation rate constants. Conditions: [PMS] = 2.5 mM, [PBS] = 0.12 M, [AO7] = 0.06 mM, [MeOH] = 1.5 M, [TBA] = 1.5 M. | |
3.7. Mechanism discussion
In the PBS/PMS system, phosphate can effectively activate peroxymonosulfate (PMS) to produce oxidizing radicals for dye degradation. Measurable degradation was not observed in the absence of PBS and the increase of PBS concentration led to the enhanced degradation rates of AO7, indicating the crucial role of PBS for PMS activation. The PBS/PMS system can be subject to pH changes, with the best performance at pH 7.0. In the PMS/Cl− system, the generation of HClO via two-electron reaction between PMS and Cl− could lead to AO7 degradation. However, the decomposition of HClO to ClO− led to a lower dye degradation efficiency in weakly acidic solution. Higher chloride concentration could favor higher dye degradation efficiency. Therefore, faster degradation of AO7 was expected in strongly acidic and higher chloride solution. In the PBS/PMS/Cl− system, a rapid AO7 degradation was observed. Both high chloride concentration and higher pH could accelerate dye degradation. Both SO4˙− and HO˙ were not detected with high levels of chloride at pH 7.0. Chlorine in the form of a liquid or gas has been applied to decolorization of dyes.48 PMS activation by both PBS and chloride may result in rapid dye degradation.
4. Conclusions
Degradation efficiency of the PMS/Cl−, PBS/PMS, and PBS/PMS/Cl− systems was investigated by varying the contents of chloride and PBS. An efficient AO7 degradation was observed while 0.12 M PBS and chloride (>50 mM) were applied in a PBS/PMS system at pH 7.0. The results shed light on the key role of PBS in dye degradation. Higher concentration of chloride ion could lead to more AOX accumulation. The low level of AOX accumulation with the same chloride concentration indicates less chlorination of organic compounds occurs in the PBS/PMS system as compared to the typical Co/PMS system. In contrast to the role of chloride in the Co/PMS system, increasing chloride ion could enhance the mineralization of AO7 in the PBS/PMS system, though with a low removal efficiency. Besides, the failure of both methanol and TBA to inhibit degradation of organics indicates low reactivity between reactive species and scavengers. HClO/Cl2 might be the dominant active oxidants with a high concentration of chloride under neutral conditions. The degradation of AO7 could undergo a non-radical reaction pathway under a chloride-rich condition. The high reactivity and wide effective pH range made PBS/PMS oxidation a promising AOP for metal-free removal of pollutants.
Conflicts of interest
There are no conflicts to declare.
Acknowledgements
This work was supported by National Key Research and Development Program of China (2016YFC0400501/2016YFC0400509) and the National Natural Science Foundation of China (NSFC) (No. 21677031). Z. H. W. would like to thank the partial support of Australian Research Council (DE150101477).
Notes and references
- S. Y. Yang, X. Yang, X. T. Shao, R. Niu and L. L. Wang, J. Hazard. Mater., 2011, 186, 659 CrossRef CAS PubMed.
- P. K. Malik and S. K. Saha, Sep. Purif. Technol., 2003, 31, 241 CrossRef CAS.
- A. Azam and A. Hamid, J. Hazard. Mater., 2006, 133, 167 CrossRef CAS PubMed.
- A. Hussein and M. Scholz, Environ. Sci. Pollut. Res., 2018, 25, 6870 CrossRef CAS PubMed.
- X. Y. Chen, X. L. Qiao, D. G. Wang, J. Lin and J. W. Chen, Chemosphere, 2007, 67, 802 CrossRef CAS PubMed.
- G. P. Anipsitakis and D. D. Dionysiou, Environ. Sci. Technol., 2003, 37, 4790 CrossRef CAS PubMed.
- G. P. Anipsitakis and D. D. Dionysiou, Appl. Catal., B, 2004, 54, 155 CrossRef CAS.
- S. H. Do, J. H. Jo, Y. H. Jo, H. K. Lee and S. H. Kong, Chemosphere, 2009, 77, 1127 CrossRef CAS PubMed.
- X. W. Liu, T. Q. Zhang, Y. C. Zhou, L. Fang and Y. Shao, Chemosphere, 2013, 93, 2717 CrossRef CAS PubMed.
- S. L. Wang, N. Zhou, S. Wu, Q. Zhang and Z. Yang, Ultrason. Sonochem., 2015, 23, 128 CrossRef CAS PubMed.
- Z. S. Wei, F. A. Villamena and L. K. Weavers, Environ. Sci. Technol., 2017, 51, 3410 CrossRef CAS PubMed.
- P. H. Shi, X. L. Wang, X. J. Zhou, Y. L. Min, J. C. Fan and W. F. Yao, RSC Adv., 2015, 5, 34125 RSC.
- S. Muhammad, E. Saputra, H. Q. Sun, J. C. Izidoro, D. A. Fungaro, H. M. Ang, M. O. Tade and S. B. Wang, RSC Adv., 2012, 2, 5645 RSC.
- C. D. Qi, X. T. Liu, J. Ma, C. Y. Lin, X. W. Li and H. J. Zhang, Chemosphere, 2016, 151, 280 CrossRef CAS PubMed.
- X. G. Duan, H. Q. Sun, J. Kang, Y. X. Wang, S. Indrawirawan and S. B. Wang, ACS Catal., 2015, 5, 4629 CrossRef CAS.
- L. Tang, Y. N. Liu, J. J. Wang, G. M. Zeng, Y. C. Deng, H. R. Dong, H. P. Feng, J. J. Wang and B. Peng, Appl. Catal., B, 2018, 231, 1 CrossRef CAS.
- A. E. Grigorev, I. E. Makarov and A. K. Pikaev, High Energy Chem., 1987, 21, 99 Search PubMed.
- L. Xu, R. X. Yuan, Y. G. Guo, D. X. Xiao, Y. Cao, Z. H. Wang and J. S. Liu, Chem. Eng. J., 2013, 217, 169 CrossRef CAS.
- J. Zhou, J. H. Xiao, D. X. Xiao, Y. G. Guo, C. L. Fang, X. Y. Lou, Z. H. Wang and J. S. Liu, Chemosphere, 2015, 134, 446 CrossRef CAS PubMed.
- N. Narender, P. Srinivasu, S. J. Kulkarni and K. V. Raghavan, Synth. Commun., 2002, 32, 279 CrossRef CAS.
- P. Wang, S. Y. Yang, L. Shan, R. Niu and X. T. Sha, J. Environ. Sci., 2011, 23, 1799 CrossRef CAS.
- R. X. Yuan, S. N. Ramjaun, Z. H. Wang and J. S. Liu, J. Hazard. Mater., 2011, 196, 173 CrossRef CAS PubMed.
- J. E. Grebel, J. J. Pignatello and W. A. Mitch, Environ. Sci. Technol., 2010, 44, 6822 CrossRef CAS PubMed.
- C. L. Fang, D. X. Xiao, W. Q. Liu, X. Y. Lou, J. Zhou, Z. H. Wang and J. S. Liu, Chemosphere, 2016, 144, 2415 CrossRef CAS PubMed.
- Z. H. Wang, M. Feng, C. L. Fang, Y. Huang, L. Y. Ai, F. Yang, Y. Xue, W. Q. Liu and J. S. Liu, RSC Adv., 2017, 7, 12318 RSC.
- J. Kiwi, A. Lopez and V. Nadtochenko, Environ. Sci. Technol., 2000, 34, 2162 CrossRef CAS.
- J. C. Alexander and C. R. Ramirez-Cortina, Ozone: Sci. Eng., 2016, 38, 181 CrossRef CAS.
- R. A. Palop and A. Marsal, J. Am. Leather Chem. Assoc., 2004, 99, 405 Search PubMed.
- G. A. Kazemi, Hydrogeol. J., 2004, 12, 723 CrossRef CAS.
- J. Nunthanid, A. Laungtana-Anan, P. Sriamornsak, S. Limmatvapirat, S. Puttipipatkhachorn, L. Y. Lim and E. Khor, J. Controlled Release, 2004, 99, 15 CrossRef CAS PubMed.
- M. Muthukumar and N. Selvakumar, Dyes Pigm., 2004, 62, 221 CrossRef CAS.
- C. Hu, J. C. Yu, Z. Hao and P. K. Wong, Appl. Catal., B, 2003, 46, 35 CrossRef CAS.
- S. Y. Yang, P. Wang, X. Yang, L. Shan, W. Y. Zhang, X. T. Shao and R. Niu, J. Hazard. Mater., 2010, 179, 552 CrossRef CAS PubMed.
- X. Y. Lou, Y. G. Guo, D. X. Xiao, Z. H. Wang, S. Y. Lu and J. S. Liu, Environ. Sci. Pollut. Res., 2013, 20, 6317 CrossRef CAS PubMed.
- X. Y. Lou, C. L. Fang, Z. N. Geng, Y. M. Jin, D. X. Xiao, Z. H. Wang, J. S. Liu and Y. G. Guo, Chemosphere, 2017, 173, 529 CrossRef CAS PubMed.
- Y. E. Yan and F. W. Schwartz, Environ. Sci. Technol., 2000, 34, 2535 CrossRef CAS.
- Y. Huang, F. Yang, L. Y. Ai, M. Feng, C. Wang, Z. H. Wang and J. S. Liu, Chemosphere, 2017, 179, 331 CrossRef CAS PubMed.
- M. Sanchez, A. Hadasch, R. T. Fell and B. Meunier, J. Catal., 2001, 202, 177 CrossRef CAS.
- P. Nfodzo and H. Choi, Chem. Eng. J., 2011, 174, 629 CrossRef CAS.
- O. S. Furman, A. L. Teel and R. J. Watts, Environ. Sci. Technol., 2010, 44, 6423 CrossRef CAS PubMed.
- Y. H. Guan, J. Ma, X. C. Li, J. Y. Fang and L. W. Chen, Environ. Sci. Technol., 2011, 45, 9308 CrossRef CAS PubMed.
- C. M. Gerritsen and D. W. Margerum, Inorg. Chem., 1990, 29, 2757 CrossRef CAS.
- Y. Feng, P. H. Lee, D. L. Wu and K. M. Shih, Environ. Sci. Technol., 2017, 51, 2312 CrossRef CAS PubMed.
- L. R. Bennedsen, J. Muff and E. G. Sogaard, Chemosphere, 2012, 86, 1092 CrossRef CAS PubMed.
- H. Eibenberger, S. Steenken, P. O'Neill and D. Schulte-Frohlinde, J. Phys. Chem., 1978, 82, 749 CrossRef CAS.
- G. V. Buxton, C. L. Greenstock, W. P. Helman and A. B. Ross, J. Phys. Chem. Ref. Data, 1988, 17, 513 CrossRef CAS.
- C. L. Clifton and R. E. Huie, Int. J. Chem. Kinet., 1989, 21, 677 CrossRef CAS.
- K. Hamada, M. Nishizawa, D. Yoshida and M. Mitsuishi, Dyes Pigm., 1998, 36, 313 CrossRef CAS.
|
This journal is © The Royal Society of Chemistry 2018 |