DOI:
10.1039/C7RA13403G
(Paper)
RSC Adv., 2018,
8, 4525-4530
Fluorine-functionalized ionic liquids with high oxygen solubility†
Received
17th December 2017
, Accepted 17th January 2018
First published on 25th January 2018
Abstract
Eight fluorine-functionalized ionic liquids were synthesized and the oxygen solubility was compared to commercial ionic liquids without the extra fluorinated chain. The concentration of dissolved oxygen increased with the fluorine content of the alkyl chain, which can be attached either to the cation or the anion. This approach maintains the freedom to design an ionic liquid for a specific application, while at the same time the oxygen solubility is increased.
1 Introduction
Ionic liquids (ILs) are solvents that consist entirely of ions.1–4 They are receiving a lot of attention due to their unique properties, such as low volatility, high (electro)chemical stability, intrinsic electrical conductivity, thermal stability and their highly tuneable properties. Research on gas solubility in ionic liquids is mainly focussed on CO2 due to the high solubility of this gas.5–7 Less data are available on the solubility of other gases such as CO, N2, H2 and O2 in ionic liquids, because the dissolved gas concentrations are considerably lower. However, this does not mean that such data would be less important. Recently, the dissolution of oxygen in ionic liquids is gaining a lot of interest, thanks to its application in (electro)catalysis and energy storage technologies.8,9 In oxidative catalysis, dissolved oxygen is used as oxidant for the oxidation of various reagents, such as alcohols, olefins, nitrotoluene and cysteine.10–20 For the latter reaction, Shan et al. observed that the performance of the catalyst was directly proportional to the solubility of oxygen and that a higher oxygen concentration resulted in higher yields. Ionic liquids with dissolved oxygen have also been used in metal-free catalytic systems for the depolymerization of lignin model compounds and lignin,21,22 or as solvent for transition-metal catalyzed oxidation of lignin model compounds.23
In the field of energy storage, more specifically for fuel cells and metal–air batteries, there is a lot of interest in the use of ionic liquids as electrolytes. For fuel cells, a high oxygen solubility in ionic liquids is crucial when they are use in (nano)composite electrodes, where it improves the catalytic four-electron oxygen reduction reaction which is necessary for fuel-cell commercialization.24–30 On the other hand, rechargeable metal–air batteries use oxygen from the atmosphere as active cathode material and thus present a higher energy density than the conventional lithium-ion battery, which is based on intercalation of ions (Li+) into a metal oxide host. The mechanism of metal–air batteries with non-aqueous electrolytes is based on a different principle and starts by the reduction of oxygen to the superoxide radical O2˙−. Subsequently this radical reacts, ideally with the metal ions in solution. Due to the high reactivity of these radicals, special attention has to be paid to the development of appropriate electrolytes that can withstand their attack. Several molecular solvents have been investigated as electrolyte for metal–air batteries, e.g. ethylene carbonate, propylene carbonate, 1,2-dimethoxyethane, diethylene glycol diethyl ether, tetraethylene glycol dimethyl ether, dimethylsulfoxide and acetonitrile.31–41 Unfortunately all these solvents have issues such high volatility, flammability, a limited stability in the presence of superoxide radicals or singlet O2,42 and a low oxygen solubility. Therefore, ionic liquids are being investigated as alternative electrolytes for metal–air batteries.43–45
For biological applications, such as artificial blood, perfluorinated carbons (PFCs) are typically used to increase the oxygen solubility in the solution.46–50 The beneficial effect of PFC additives on the performance of metal–air batteries has already been reported in the literature.51–55 Nevertheless, the limited miscibility of PFCs in organic solvents remains an issue. One proposed strategy is to make a dispersion of the liquid medium.54 However, this approach does not meet the long-term stability requirement of the two-phase liquid/liquid dispersion. Another type of organic solvents with a high fluorine content besides the perfluorinated hydrocarbons are some type of ionic liquids (ILs). Typically, fluorine is introduced in the ionic liquid via the anion. Examples include tetrafluoroborate, hexafluorophosphate and bis(trifluoromethylsulfonyl)imide anions. Much less examples are known of ionic liquids with fluorinated alkyl chains in the cation.56–61 An alternative approach is via perfluorinated alkyl chains in the anion.62–65 However, this approach leads in general to ionic liquids with higher melting points.
In this paper, ionic liquids were functionalized with fluorinated alkyl chains in the cation or anion, making them an inherent part of the electrolyte and not an additive. After the characterization of the physical properties of these fluorinated ionic liquids such as their density, viscosity and thermal stability, electrochemical experiments were conducted to determine the oxygen solubility in those fluorinated ionic liquids, which was shown to be increased with fluorinated chain length.
2 Experimental
The detailed synthesis and characterization of precursor molecules and ionic liquids is described in the ESI.† The ionic liquids were characterized by NMR (1H, 13C, 19F), FTIR-ATR, CHN elemental analysis and TGA. In addition, the water content, the viscosity and density of the ionic liquids were measured. All ionic liquids were dried on a vacuum line at 70 °C for 72 hours and subsequently stored in an argon-filled glove box with oxygen and water concentrations below 1 ppm. The water content in the ionic liquids was determined by Karl Fischer coulometry (Mettler-Toledo model DL39) and was always below 20 ppm. Viscosity (dynamic and kinematic) and density of the ionic liquids were measured by an Anton Paar Lovis 2000 M/ME, DMA 4500 M rolling-ball viscometer at 25 °C. Thermogravimetric analysis was performed by using a SDT Q600 (TA Instruments) under a constant argon flow. First the mass was monitored for 60 min at 25 °C to evaluate the volatility at room temperature. Subsequently the temperature was increased to 450 °C at a heating rate of 5 °C min−1, to evaluate the volatility and thermal stability at higher temperatures. All electrochemical experiments were performed using a three-electrode set up. The electrochemical cell was filled and closed inside the glove box, followed by gas bubbling outside the glove box. Oxygen gas (≥99.9995%, Air Liquide, ALPHAGAZ 2) was used for the oxygen dissolution measurements in the ionic liquids. The gas was bubbled through the ionic liquid via a glass tube with a P2 glass filter for 30 minutes. The bubbler was connected to the gas bottle using silicone tubing. To avoid water contamination during bubbling, an extra drying tower (Sigma-Aldrich) was used, filled with activated molecular sieves 3 Å (beads, 4–8 mesh, Sigma-Aldrich) and placed in between the gas bottle and the electrochemical cell. The molecular sieves were activated overnight at a temperature of 250 °C under a flow of argon. All glassware used for the experiments was dried overnight in an oven at 120 °C and transferred into the glove box while still hot. All electrochemical measurements were performed using an Autolab 302N bipotentiostat controlled by NOVA 1.11.1 software. Two different working electrodes were used: (1) a gold disk macroelectrode (ø = 0.6 mm) or (2) a gold ultramicroelectrode (ø = 25 μm, CH Instruments Inc.). The real reference electrode was a silver wire (≥99.98%, Chempur) in a glass tube filled with a dry acetonitrile solution of 0.01 M silver nitrate and 0.1 M tetrabutylammonium perchlorate, which was separated from the electrolyte by a glass frit. The reference tube was assembled at least 30 minutes prior to the electrochemical measurement. A platinum coil was used as counter electrode. The electrochemical cell was placed in an oil bath at 25.0 ± 0.2 °C.
3 Results and discussion
3.1 Synthesis and characterization
The perfluorinated cations were based on a piperidinium or pyrrolidinium heterocycle or a quaternary ammonium. The perfluorinated anions were based on a carboxylate ion (Fig. 1). For the synthesis of the perfluorinated cations, a general synthetic procedure was followed: (1) synthesis of the fluorinated amino ether, (2) synthesis of the iodide salt and (3) preparation of the final bis(trifluoromethylsulfonyl)imide (Tf2N−) ionic liquid by a metathesis reaction with LiTf2N. The first step was based on a modified procedure described by Kim et al.66
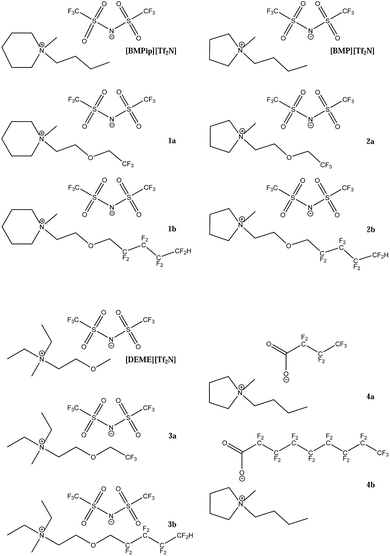 |
| Fig. 1 Structures of the fluorinated ionic liquids studied in this work, 1a, b: piperidinium-based ionic liquids with the fluorinated chain attached to the cation. 2a, b: pyrrolidinium-based ionic liquids with the fluorinated chain attached to the cation. 3a, b: quaternary ammonium-based ionic liquids with the fluorinated chain attached to the cation. 4a, b: carboxylate-based ionic liquids with the fluorinated chain attached to the anion. [BMPip][Tf2N]: N-butyl-N-methylpiperidinium bis(trifluoromethylsulfonyl)imide, [BMP][Tf2N]: N-butyl-N-methylpyrrolidinium bis(trifluoromethylsulfonyl)imide, [DEME][Tf2N]: N,N-diethyl-N-(2-methoxyethyl)-N-methylammonium bis(trifluoromethylsulfonyl)imide. | |
The physical properties of the fluorinated ionic liquids are reported in Table 1. It is evident that the density and viscosity of the ionic liquids increased with the fluorine content of the alkyl chain. All six ionic liquids with a fluorinated chain attached to the cation (1a, 1b, 2a, 2b, 3a, 3b) were liquid at room temperature and the dissolved oxygen concentration could be measured as such in the pure ionic liquid. The two ionic liquids 4a and 4b with the fluorinated chain attached to the anion, were solid at room temperature and had to be mixed with N-butyl-N-methylpyrrolidinium bis(trifluoromethylsulfonyl)imide ([BMP][Tf2N]) in a 1
:
1 mass ratio. The resulting ionic liquid mixture was liquid around room temperature and could be used for the electrochemical measurements.
Table 1 Physical properties of the fluorinated ionic liquids used in this work measured at 25 °C
Ionic liquid |
Density (kg m−3) |
Dynamic viscosity (mPa s) |
Decomposition temperaturec (°C) |
Ionic liquids 4a, b were solid at room temperature and were mixed with [BMP][Tf2N] in a 1 : 1 mass ratio. Measured at 35 °C. Dynamic TGA, heating rate 5 °C min−1. The decomposition temperature is determined as the temperature where 1% of the initial mass of the ionic liquid is decomposed. |
[BMPip][Tf2N] |
1379 |
166 |
385 |
1a |
1501 |
228 |
327 |
1b |
1583 |
587 |
341 |
[BMP][Tf2N] |
1395 |
82 |
357 |
2a |
1530 |
116 |
329 |
2b |
1609 |
320 |
337 |
[DEME][Tf2N] |
1407 |
77 |
325 |
3a |
1481 |
144 |
329 |
3b |
1566 |
446 |
302 |
4aa,b |
1295 |
178 |
137 |
4ba |
1442 |
185 |
141 |
3.2 Dissolved oxygen concentration
The dissolved oxygen concentration was obtained via electrochemical measurements and was calculated by combining the Cottrell equation (eqn (1), Fig. 2) on a macroelectrode and the steady-state current on an ultramicroelectrode described by eqn (2) (Fig. 3), for the reduction of oxygen: O2 + e− ⇌ O2˙−. Detailed experimental conditions can be found in the ESI.† |
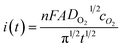 | (1) |
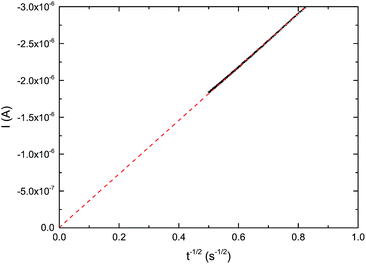 |
| Fig. 2 Linear fit for the determination of the slope the Cottrell equation (eqn (1)). A potentiostatic signal of −1.65 V vs. Ag+/Ag was applied, using IL 2a as electrolyte, a gold macroelectrode (ϕ = 0.6 mm) and a platinum counter electrode at room temperature. | |
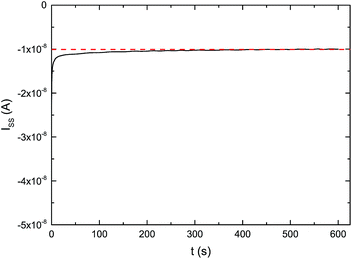 |
| Fig. 3 Steady state current on the ultramicroelectrode iss equation (eqn (2)). A potentiostatic signal of −1.65 V vs. Ag+/Ag was applied, using IL 2a as electrolyte, a gold ultramicroelectrode (ϕ = 25 μm) as working electrode and a platinum counter electrode at room temperature. | |
Combining these equations gave values for the dissolved oxygen concentration cO2 and oxygen diffusion coefficient DO2 (Table 2). In Fig. 4, an increase of the oxygen concentration with the fluorine content of the alkyl chain is observed. Compared to the non-fluorinated ionic liquids oxygen solubility increases for the piperidinium cations 2.5 and 4.1 times, for the pyrrolidinium cations 3.9 and 5.1 times and for the quaternary ammonium cations 3.1 and 5.8 times when respectively a trifluoroethoxy and an octafluoropentoxy chain is attached to the cation. The highest oxygen concentration of 34.0 mol m−3 was measured for IL 2b. The oxygen solubility increases from 9.6 mol m−3 to 19.5 mol m−3 upon increasing the fluorinated chain length on the carboxylate-based anion, from hexafluorobutanoate to heptadecafluorononanoate, respectively. The results are summarized in Table 2 and in the ESI.†
Table 2 Dissolved oxygen concentration (cO2), oxygen diffusion coefficient (DO2) measured at 25 °C
Ionic liquid |
cO2 (mol m−3) |
DO2 (×10−10 m2 s−1) |
Ionic liquids 4a, b were solid at room temperature and were mixed with [BMP][Tf2N] in a 1 : 1 mass ratio. Measured at 35 °C. |
[BMPip][Tf2N] |
6.3 |
2.2 |
1a |
16.0 |
0.8 |
1b |
26.1 |
0.2 |
[BMP][Tf2N] |
6.7 |
5.0 |
2a |
26.0 |
0.8 |
2b |
34.0 |
0.3 |
[DEME][Tf2N] |
5.1 |
4.8 |
3a |
16.0 |
0.7 |
3b |
29.5 |
0.2 |
4aa,b |
9.6 |
1.2 |
4ba |
19.5 |
1.0 |
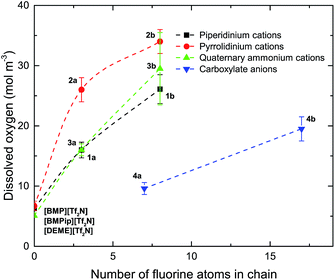 |
| Fig. 4 Influence of fluorine chain length on the dissolved oxygen concentration. | |
3.3 Oxygen supply capacity for metal–air batteries
Since ionic liquids are in general more viscous than molecular solvents and even more so upon increasing the fluorinated chain length, there is a tradeoff between the length of the fluorinated chain to increase the oxygen solubility on one hand and keeping viscosity as low as possible on the other hand. This is also reflected in the decrease of the diffusion coefficient of oxygen with an increasing viscosity and fluorinated chain length (Table 2). Especially for heterogeneous reactions, this is an important parameter to consider. For example, in metal–air batteries the current at the electrode interface is proportional to
. This value can be seen as the oxygen supply capacity in a stagnant solution and therefore it is a more appropriate parameter to compare the various ionic liquids as electrolytes for metal–air batteries.67 In Fig. 5 it can be seen that for the perfluorinated cations, IL 2a and 3b perform the best with an oxygen supply capacity that is 1.6 and 1.4 times higher than that of a commercial ionic liquid [BMP][Tf2N] and [DEME][Tf2N], respectively. Note that the oxygen concentration is a more important factor than the oxygen diffusion coefficient for the oxygen supply capacity. For IL 4a and 4b it can be seen that mixing the fluorinated ionic liquids with [BMP][Tf2N] results in a comparable viscosity and thus a comparable oxygen diffusion coefficient. Doubling the oxygen solubility by increasing the fluorinated chain length, results thus in an oxygen supply capacity that is twice as high. For flow-type systems or homogeneous catalysis, the contribution of the diffusion coefficient becomes even less pronounced for the supply of oxygen. Due to the limited diffusion path length, a high dissolved oxygen concentration will be more important and result in higher reaction rates.
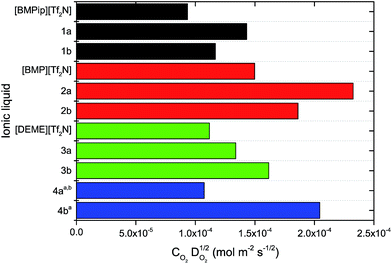 |
| Fig. 5 Oxygen supply capacity of different ionic liquids for the heterogenous oxygen reduction reaction, where the current . aIonic liquids 4a, b were solid at room temperature and were mixed with [BMP][Tf2N] in a 1 : 1 mass ratio. bMeasured at 35 °C. | |
3.4 Stability of the ionic liquids
An extra advantage of using fluorinated ionic liquids is their hydrophobicity and low volatility. Therefore, these ionic liquids can be used as solvents in open or half open systems, and even in flow gas systems. The volatility was assessed using thermogravimetric analysis. The mass of the ionic liquids was monitored for 1 hour at 25 °C and subsequently, the temperature was increased until the ionic liquid started to decompose. For none of the ionic liquids a mass decrease was observed before the decomposition temperature (Fig. S4–S7†). This is a clear indication that the ionic liquids are non-volatile, not only at room temperature, but also at elevated temperatures. For all ionic liquids with the fluorinated chain attached to the cation, the decomposition temperature was higher than 300 °C. Ionic liquids with a fluorinated carboxylate-anion decomposed at lower temperatures starting at 137 °C for IL 4a and 141 °C for IL 4b (Table 1). The stability of the ionic liquids against attack of the superoxide radical was assessed by cyclic voltammetry. This is important for applications where dissolved oxygen is reduced to its radical, e.g. metal–air batteries. Although the time required to measure a cyclic voltammogram is relatively short, it provides an insight in the stability and complexation of the superoxide radical in the ionic liquid. All ionic liquids showed a reversible oxygen reduction reaction and oxygen evolution reaction at a slow scan rate of 10 mV s−1 during a cyclic voltammogram (Fig. S8–S11†).
4 Conclusions
In conclusion, various fluorinated ionic liquids were synthesized to improve the oxygen solubility. Both cations and anions were fluorinated and this resulted in an increase in oxygen solubility. Concentrations up to 34 mM were measured. This is more than five times the concentration of dissolved oxygen in the commercial ionic liquid without a fluorinated alkyl chain. The dissolved oxygen concentration was determined via electrochemical measurements on a macro- and ultramicroelectrode. The stability, low vapor pressure and hydrophobic character of the ionic liquids make them suitable for use in open, half-open or flow systems. Since fluorination of both the cation and anion results in a higher oxygen solubility, there is a freedom to operate and thus, depending on the application, the cation or anion can be used to increase the dissolved oxygen concentrations in the ionic liquid.
Conflicts of interest
There are no conflicts to declare.
Acknowledgements
The authors acknowledge financial support of the Battery Research division (M6) at Higashi Fuji Technical Centre in Japan and the IWT Flanders (SBO project 18142 “SoS-Lion”) and the KU Leuven (IOF-KP 14/005 “Better Batteries”). We thank Karel Duerinckx for his assistance with the NMR measurements.
References
- K. R. Seddon, J. Chem. Technol. Biotechnol., 1997, 68, 351–356 CrossRef CAS
. - T. Welton, Chem. Rev., 1999, 99, 2071–2084 CrossRef CAS PubMed
. - F. Endres, ChemPhysChem, 2002, 3, 144–154 CrossRef CAS
. - K. Seddon, Nat. Mater., 2003, 2, 363–365 CrossRef CAS PubMed
. - E. D. Bates, R. D. Mayton, I. Ntai and J. H. Davis, J. Am. Chem. Soc., 2002, 124, 926–927 CrossRef CAS PubMed
. - C. Cadena, J. L. Anthony, J. K. Shah, T. I. Morrow, J. F. Brennecke and E. J. Maginn, J. Am. Chem. Soc., 2004, 126, 5300–5308 CrossRef CAS PubMed
. - A. M. Scurto, S. N. V. K. Aki and J. F. Brennecke, J. Am. Chem. Soc., 2002, 124, 10276–10277 CrossRef CAS PubMed
. - D. Betz, P. Altmann, M. Cokoja, W. A. Herrmann and F. E. Kühn, Coord. Chem. Rev., 2011, 255, 1518–1540 CrossRef CAS
. - M. Armand, F. Endres, D. R. MacFarlane, H. Ohno and B. Scrosati, Nat. Mater., 2009, 8, 621–629 CrossRef CAS PubMed
. - N. Jiang and A. J. Ragauskas, J. Org. Chem., 2007, 72, 7030–7033 CrossRef CAS PubMed
. - A. Shaabani, E. Farhangi and A. Rahmati, Appl. Catal., A, 2008, 338, 14–19 CrossRef CAS
. - S. Riaño, D. Fernández and L. Fadini, Catal. Commun., 2008, 9, 1282–1285 CrossRef
. - S. Kodama, Y. Ueta, J. Yoshida, A. Nomoto, S. Yano, M. Ueshima and A. Ogawa, Dalton Trans., 2009, 9708–9711 RSC
. - H.-Y. Shen, L.-Y. Ying, H.-L. Jiang and Z. M. A. Judeh, Int. J. Mol. Sci., 2007, 8, 505–512 CrossRef CAS
. - Z. Zhang, L. Hui, L. Yürong and Y. Yühua, Synth. React. Inorg. Met.-Org. Chem., 2009, 39, 144–148 CAS
. - X. Yun, X. Hu, Z. Jin, J. Hu, C. Yan, J. Yao and H. Li, J. Mol. Catal. A: Chem., 2010, 327, 25–31 CrossRef CAS
. - A. Chrobok, Tetrahedron, 2010, 66, 2940–2943 CrossRef CAS
. - X. Zhao, A. Kong, C. Shan, P. Wang, X. Zhang and Y. Shan, Catal. Lett., 2009, 131, 526–529 CrossRef CAS
. - X. Zhao, A. Kong, X. Zhang, C. Shan, H. Ding and Y. Shan, Catal. Lett., 2010, 135, 291–294 CrossRef CAS
. - M. J. Schultz and M. S. Sigman, Tetrahedron, 2006, 62, 8227–8241 CrossRef CAS
. - Y. Yang, H. Fan, J. Song, Q. Meng, H. Zhou, L. Wu, G. Yang and B. Han, Chem. Commun., 2015, 51, 4028–4031 RSC
. - G. Chatel and R. D. Rogers, ACS Sustainable Chem. Eng., 2014, 2, 322–339 CrossRef CAS
. - J. Zakzeski, A. L. Jongerius and B. M. Weckhuysen, Green Chem., 2010, 12, 1225–1236 RSC
. - J. Snyder, T. Fujita, M. W. Chen and J. Erlebacher, Nat. Mater., 2010, 9, 904–907 CrossRef CAS PubMed
. - Y. Tan, C. Xu, G. Chen, N. Zheng and Q. Xie, Energy Environ. Sci., 2012, 5, 6923–6927 CAS
. - J. Snyder, K. Livi and J. Erlebacher, Adv. Funct. Mater., 2013, 23, 5494–5501 CrossRef CAS
. - C. Chen, Y. Kang, Z. Huo, Z. Zhu, W. Huang, H. L. Xin, J. D. Snyder, D. Li, J. A. Herron, M. Mavrikakis, M. Chi, K. L. More, Y. Li, N. M. Markovic, G. A. Somorjai, P. Yang and V. R. Stamenkovic, Science, 2014, 343, 1339–1343 CrossRef CAS PubMed
. - E. Benn, H. Uvegi and J. Erlebacher, J. Electrochem. Soc., 2015, 162, H759–H766 CrossRef CAS
. - G.-R. Zhang and B. J. Etzold, J. Energy Chem., 2016, 25, 199–207 CrossRef
. - H. A. Gasteiger, S. S. Kocha, B. Sompalli and F. T. Wagner, Appl. Catal., B, 2005, 56, 9–35 CrossRef CAS
. - W. Xu, J. Xiao, D. Wang, J. Zhang and J.-G. Zhang, J. Electrochem. Soc., 2010, 157, A219–A224 CrossRef CAS
. - W. Xu, J. Xiao, J. Zhang, D. Wang and J.-G. Zhang, J. Electrochem. Soc., 2009, 156, A773–A779 CrossRef CAS
. - S. A. Freunberger, Y. Chen, N. E. Drewett, L. J. Hardwick, F. Bardé and P. G. Bruce, Angew. Chem., Int. Ed., 2011, 50, 8609–8613 CrossRef CAS PubMed
. - F. Mizuno, S. Nakanishi, A. Shirasawa, K. Takechi, T. Shiga, H. Nishikoori and H. Iba, Electrochemistry, 2011, 79, 876–881 CrossRef CAS
. - C. O. Laoire, S. Mukerjee, E. J. Plichta, M. A. Hendrickson and K. M. Abraham, J. Electrochem. Soc., 2011, 158, A302–A308 CrossRef CAS
. - J. Herranz, A. Garsuch and H. A. Gasteiger, J. Phys. Chem. C, 2012, 116, 19084–19094 CAS
. - B. M. Gallant, D. G. Kwabi, R. R. Mitchell, J. Zhou, C. V. Thompson and Y. Shao-Horn, Energy Environ. Sci., 2013, 6, 2518–2528 CAS
. - C. O. Laoire, S. Mukerjee, K. M. Abraham, E. J. Plichta and M. A. Hendrickson, J. Phys. Chem. C, 2009, 113, 20127–20134 CAS
. - Z. Peng, S. A. Freunberger, L. J. Hardwick, Y. Chen, V. Giordani, F. Bardé, P. Novák, D. Graham, J.-M. Tarascon and P. G. Bruce, Angew. Chem., 2011, 123, 6475–6479 CrossRef
. - F. Bardé, Y. Chen, L. Johnson, S. Schaltin, J. Fransaer and P. G. Bruce, J. Phys. Chem. C, 2014, 118, 18892–18898 Search PubMed
. - K. U. Schwenke, S. Meini, X. Wu, H. A. Gasteiger and M. Piana, Phys. Chem. Chem. Phys., 2013, 15, 11830–11839 RSC
. - A. C. Luntz and B. D. McCloskey, Nat. Energy, 2017, 2, 17056 CrossRef
. - C. J. Allen, J. Hwang, R. Kautz, S. Mukerjee, E. J. Plichta, M. A. Hendrickson and K. M. Abraham, J. Phys. Chem. C, 2012, 116, 20755–20764 CAS
. - G. Girishkumar, B. McCloskey, A. C. Luntz, S. Swanson and W. Wilcke, J. Phys. Chem. Lett., 2010, 1, 2193–2203 CrossRef CAS
. - T. Ogasawara, A. Débart, M. Holzapfel, P. Novák and P. G. Bruce, J. Am. Chem. Soc., 2006, 128, 1390–1393 CrossRef CAS PubMed
. - J. G. Riess, Colloids Surf., A, 1994, 84, 33–48 CrossRef CAS
. - J. G. Riess, Chem. Rev., 2001, 101, 2797–2920 CrossRef CAS PubMed
. - A. M. A. Dias, R. P. Bonifacio, I. M. Marrucho, A. A. H. Padua and M. F. C. Gomes, Phys. Chem. Chem. Phys., 2003, 5, 543–549 RSC
. - M. F. C. Gomes, J. Deschamps and D. H. Mertz, J. Fluorine Chem., 2004, 125, 1325–1329 CrossRef
. - Fluorine and Health: Molecular Imaging, Biomedical Materials and Pharmaceuticals, ed. M. P. Krafft and J. G. Riess, Elsevier, Amsterdam, 2008 Search PubMed
. - R. Yazami, US Pat. 0266907 A1, October 21, 2010
. - M. Balaish, A. Kraytsberg and Y. Ein-Eli, Phys. Chem. Chem. Phys., 2014, 16, 2801–2822 RSC
. - M. Balaish, A. Kraytsberg and Y. Ein-Eli, ChemElectroChem, 2014, 1, 90–94 CrossRef
. - Y. Wang, D. Zheng, X.-Q. Yang and D. Qu, Energy Environ. Sci., 2011, 4, 3697–3702 CAS
. - Y. Nishikami, T. Konishi, R. Omoda, Y. Aihara, K. Oyaizu and H. Nishide, J. Mater. Chem. A, 2015, 3, 10845–10850 CAS
. - T. L. Merrigan, E. D. Bates, S. C. Dorman and J. H. Davis, Chem. Commun., 2000, 2051–2052 RSC
. - J. E. Bara, T. K. Carlisle, C. J. Gabriel, D. Camper, A. Finotello, D. L. Gin and R. D. Noble, Ind. Eng. Chem. Res., 2009, 48, 2739–2751 CrossRef CAS
. - D. Almantariotis, T. Gefflaut, A. A. H. Padua, J. Y. Coxam and M. F. C. Gomes, J. Phys. Chem. B, 2010, 114, 3608–3617 CrossRef CAS PubMed
. - T. Alpers, M. Schmidtmann, T. W. T. Muesmann, O. Temme and J. Christoffers, Eur. J. Org. Chem., 2017, 4283–4290 CrossRef CAS
. - D. Rauber, P. Zhang, V. Huch, T. Kraus and H. Rolf, Phys. Chem. Chem. Phys., 2017, 19, 27251–27258 RSC
. - D. Rauber, F. Heib, M. Schmitt and R. Hempelmann, Colloids Surf., A, 2018, 537, 116–125 CrossRef CAS
. - A. B. Pereiro, J. a. M. M. Araujo, S. Martinho, F. Alves, S. Nunes, A. Matias, C. M. M. Duarte, L. P. N. Rebelo and I. M. Marrucho, ACS Sustainable Chem. Eng., 2013, 1, 427–439 CrossRef CAS
. - D. Almantariotis, A. S. Pensado, H. Q. N. Gunaratne, C. Hardacre, A. A. H. Padua, J. Y. Coxam and M. F. C. Gomes, J. Phys. Chem. B, 2017, 121, 426–436 CrossRef CAS PubMed
. - A. B. Pereiro, F. Llovell, J. a. M. M. Araujo, A. S. S. Santos, L. P. N. Rebelo, M. M. Pineiro and L. F. Vega, ChemPhysChem, 2017, 18, 2012–2023 CrossRef CAS PubMed
. - D. Rauber, F. Heib, T. Dier, D. A. Volmer, R. Hempelmann and M. Schmitt, Colloids Surf., A, 2017, 529, 169–177 CrossRef CAS
. - J. Kim, R. P. Singh and J. M. Shreeve, Inorg. Chem., 2004, 43, 2960–2966 CrossRef CAS PubMed
. - H. Nakamoto, Y. Suzuki, T. Shiotsuki, F. Mizuno, S. Higashi, K. Takechi, T. Asaoka, H. Nishikoori and H. Iba, J. Power Sources, 2013, 243, 19–23 CrossRef CAS
.
Footnote |
† Electronic supplementary information (ESI) available: The detailed synthesis and characterization of precursor molecules and ionic liquids is described: 1H, 13C, 19F NMR spectra, CHN analysis data, thermogravimetric analysis (TGA), electrochemical procedures and measurements. See DOI: 10.1039/c7ra13403g |
|
This journal is © The Royal Society of Chemistry 2018 |
Click here to see how this site uses Cookies. View our privacy policy here.