DOI:
10.1039/C7RA12092C
(Paper)
RSC Adv., 2018,
8, 230-242
Combination of icariin and oleanolic acid attenuates in vivo and in vitro glucocorticoid resistance through protecting dexamethasone-induced glucocorticoid receptor impairment
Received
3rd November 2017
, Accepted 6th December 2017
First published on 2nd January 2018
Abstract
Glucocorticoid resistance (GCR) remains a significant problem and is the most important reason for treatment failure of glucocorticoids (GCs). How to increase the sensitivity to GCs and exploring new agents to overcome GCR is of great significance. Herein, we report for the first time that the effects of combination of icariin and oleanolic acid (OA) on dexamethasone (Dex)-induced glucocorticoid receptor (GR) impairment in vivo and in vitro. Compared with the use of Dex or combination of icariin and OA alone, co-administration resulted in a significant increase in apoptosis, GR protein, GR binding and GRα protein both in GC-sensitive CEM-C7 and GC-resistant CEM-C1 cells and a decrease in GRβ protein and GRβ/GRα only in CEM-C1 cells. Furthermore, an ovalbumin-induced rat asthma model was chosen to evaluate the effects of co-administration of Dex and combination of icariin and OA in vivo. Compared with the use of Dex alone, co-administration could protect blood lymphocyte from excessive apoptosis, significantly increasing GR binding, GR protein and mRNA of GRα while decreasing GRβ protein and mRNA of GRβ. The results suggested that combination of icariin and OA could increase sensitivity to GCs through attenuating in vivo and in vitro Dex-induced GR impairment.
1. Introduction
Glucocorticoids (GCs) are among the most prescribed drugs in the world and are widely used in chemotherapy either as a primary cytotoxic drug targeting cancer cells (as in acute leukemia, lymphoma and multiple myeloma) or to reduce inflammation, prevent allergic reactions, etc.1,2 However, when GCs are administered in high doses or used long-term, a series of side effects would be produced, such as growth stunting in children, hypothalamic-pituitary-adrenal (HPA) axis dysfunction, osteopenia, GC dependence and GC resistance (GCR).3–5 GCR remains a significant problem and is the most important reason for treatment failure of GCs. Although most studies have focused on asthma, GCR is found in many other autoimmune and inflammatory diseases. For instance, 30% of rheumatoid arthritis patients and almost all chronic obstructive pulmonary disease patients exhibit GCR.6 Therefore, how to increase the sensitivity to GCs and exploring new agents to overcome GCR is of great significance.
GC signaling has various effects on the immune system, and extensive research has been carried out on T lymphocytes.7 Loss of T cells via apoptosis is a major cause of the immunosuppressive actions of GCs.8 GCR is often associated with the development of resistant T cells that no longer respond to GC-evoked apoptosis.9 The primary action of GCs is mediated through their interactions with the glucocorticoid receptor (GR).1 GR protein down-regulation could be the result of many different mechanisms of GCR,10 including homologous down-regulation (ligand-induced down-regulation of GR), reduced transcription,11 decreased stability of GR mRNA12 and GR protein degradation.13 GR transcriptional activity correlates with susceptibility of cells to GC-evoked apoptosis.14 Alternative splicing of GR near the 30 UTR in exon 9 generates two major isoforms (GRα and GRβ). The classical human GRα consists of 777 amino acids, while GRβ consists of only 742 amino acids and is unable to bind GCs, resulting in a dominant negative protein. Subsequently, up-regulation of the dominant negative GRβ isoform has been found to be associated with GR insensitivity in many diseases.15 In addition, problems with chaperone proteins and cofactors involved in GR signaling can contribute to reduced GC sensitivity. In short, GC insensitivity could be primarily caused by problems in the GR protein itself.
According to traditional Chinese medicine (TCM) theories, the use of GCs in clinical practice affects kidney function and leads to kidney deficiency syndrome.16 The decrease in GR protein or GR binding caused by administration of GCs has been proved to play a key role in the pathogenesis of kidney deficiency syndrome. Hence, many TCM doctors adopt the method of tonifying the kidney to prevent GR impairment. Epimedii Folium (EF) and Ligustri Lucidi Fructus (LLF) have been documented as kidney-yang and kidney-yin replenishing herbs, respectively. In our previous research, we have demonstrated that the decoction of EF and LLF could prevent the decrease of mRNA and protein expression of GR induced by dexamethasone (Dex) in asthmatic rats,17 and the active ingredients of EF and LLF (total flavonoids and triterpenoids) can effectively enhance the sensitivity of asthmatic rats to budesonide (Bun) via increasing lymphocyte apoptosis and balancing GR/heat shock protein 90(HSP90).18 The active ingredients of EF and LLF primarily contain icariin and oleanolic acid (OA). Icariin is a major constituent of EF and exerts a wide range of pharmacological activities. It has been reported that icariin can alter the expression of GR, regulate the normalization of GR distribution between the cytoplasm and the nucleus and protect against corticosterone-induced apoptosis.19,20 OA is one of the best known bioactive pentacyclic triterpenoids and of great interest to scientists because of its several biological activities, including anti-HIV, diuretic, nephroprotective, antidiabetic, antioxidant and hepatoprotective activities. Recent research has demonstrated that OA prevents Dex-induced hypertension in rats through its antioxidant and nitric oxide releasing action.21,22 We speculate that the combination of icariin and OA has the function of increasing protein and mRNA expression of GR. In the present study, a combination of icariin and OA was administered to investigate its potential for sensitizing GC-resistant CEM-C1 cells to Dex, and the results showed that the combination could effectively sensitize GC-resistant CEM-C1 cells to Dex-induced apoptosis by activation of GR and regulate GR isoforms. Moreover, we selected ovalbumin (OVA)-induced asthma rats to explore the preventive effects of the combination of icariin and OA on Dex-induced GR impairment in vivo.
2. Materials and methods
2.1. Cell lines and treatment protocol
Human T-ALL cell lines of GC-sensitive CEM-C7-14 and GC-resistant CEM-C1-15 were purchased from Shanghai Huzhen Biological Technology Co., Ltd (Shanghai, China). We refer to these cell lines as CEM-C7 and CEM-C1 in the following text. Both CEM-C7 and CEM-C1 cells were maintained in RPMI-1640 medium supplemented with 10% fetal bovine serum, 2 mM glutamine and 100 U mL−1 penicillin/streptomycin and cultured at 37 °C in 5% CO2 humidified atmosphere.23 Cells were passaged once every 3 days. Cells in the logarithmic growth phase were seeded in a 6-well plate at a density of 4 × 105 mL−1 and then treated with 30 μL Dex (10−6 mol L−1), icariin (100 mg mL−1), OA (100 mg mL−1), icariin&OA (icariin 50 mg mL−1 and OA 50 mg mL−1), and Dex + icariin&OA (Dex 10−6 mol L−1, icariin 50 mg mL−1 and OA 50 mg mL−1) for 24 h. Dex was obtained from Sigma-Aldrich, USA. Icariin [98% purity, as verified by high-performance liquid chromatography (HPLC)] and OA (99.44% purity, as verified by HPLC) used in this study were purchased from Chengdu Biopurify Phytochemicals Ltd. (Chendu, China).
2.2. Animals and treatment protocol
SPF-grade male Sprague-Dawley rats, weighing 120 to 130 g with the average age of four or five weeks, were purchased from Vital River Laboratory Animal Technology Co. Ltd. (Beijing, China). The experiment complied with the Animal Management Rule of the Ministry of Public Health, China, and the experimental protocol was approved by the Ethics Committee of Capital Medical University (No. AEEI-2015-042). All the animals were cared for in the Experimental Animal Center of Capital Medical University. During the entire experiment, the animals were housed in stainless cages (three rats per cage) at conventional controlled conditions (temperature of 23 ± 2 °C, relative humidity of 50 ± 10%, and 12 h light–dark cycle). They were given free access to the standard laboratory food and tap water.
The rats were acclimated to conditions for one week before the experiment and randomly assigned to the following 5 groups (n = 6): normal control group (control), asthma model group (asthma), Dex group, icariin&OA group, and co-administration of Dex and icariin&OA group (Co-administration). OVA sensitization and challenge protocols were performed according to our previous methods.18 On days 1 and 8, all rats except those in the control group were actively sensitized with intraperitoneal injection (ip) and subcutaneous injection of 1 mg OVA and 100 μg aluminum hydroxide in 1 mL sterile physiological saline. The OVA-sensitized rats were exposed to 1% aerosolized OVA (1 g OVA in 100 mL sterile physiological saline in an ultrasonic nebulizer) for 30 min from day 15 to day 35. Rats in the control group were exposed to sterile physiological saline only. The asthma group received no treatment; Dex and Co-administration groups received 5 mg kg−1 Dex sodium phosphate injection (ip) (Rongsheng Pharmaceutical Co., Ltd, Henan, China); both icariin&OA and Co-administration groups received 100 mg kg−1 herbal ingredients via intragastric administration (ig). All treatments were conducted from day 15 to 35, once a day for 21 days.
On day 36, all the rats were bled and sacrificed with anaesthesia of ethyl carbamate 24 h after the last OVA exposure. Blood samples were collected by abdominal aortic puncture. Lungs were lavaged 3 times with ice-cold saline (3 mL, 3 mL and 4 mL) using a tracheal cannula and a 5 mL polyethylene syringe. The cell-debris pellets of the bronchoalveolar lavage fluid (BALF) samples were collected after centrifugation. Lymphocytes were separated from peripheral blood (PB) and BALF samples by assay of rat lymphocyte separation solution (Tinjin Haoyang Biological Manufacture Co., Ltd., Tianjin, China) and then resuspended with 1 mL of 4% paraformaldehyde-phosphate buffer solution.
2.3. Apoptosis assay by flow cytometry (FCM)
Apoptosis in CEM-C7 and CEM-C1 cells was detected using an Annexin-FITC apoptosis detection kit (MultiSciences Biotech Co., Ltd, Hangzhou, China). Cells were collected, washed and resuspended at 4 × 105 cells per mL in 500 μL binding buffer containing 5 μL Annexin V-FITC stock solution and 10 μL propidium iodide (PI). After incubation for 5 min at room temperature in the dark, apoptosis of cells was detected by FCM. Apoptosis in lymphocytes of PB and BALF of rats was determined using the assay of TdT–dUTP terminal nick-end labeling (TUNEL, MBL Inc., Nagoya, Japan) for FCM according to the manufacturer's instruction.23 A FACS Calibur flow cytometer (BD Biosciences, San Jose, CA, USA) was used to determine the lymphocyte apoptosis level through mean fluorescence intensity (MFI).
2.4. GR-mAb-FCM analysis and FITC labelled-Dex-FCM analysis
Anti-GR mAb and anti-mouse PE labelled IgG (Abcam, Cambridge, UK) were used for the detection of GR protein expression. PE-labelled mouse IgG1 isotype (Abcam, Cambridge, UK) was used for the control samples. CEM-C7 and CEM-C1 cells were washed with PBS twice and resuspended in T liquid (3% Triton-X 100 and 10% normal goat serum) and incubated on ice for 10 min. Resuspended lymphocytes in PB and BALF were washed with PBS twice and refixed in 100 μL of 4% buffered paraformaldehyde for 30 min at 4 °C. Nonspecific binding sites were blocked with 5% normal goat serum containing permeabilisation buffer for 30 min. Cells were then incubated with 100 μL permeabilisation buffer containing 50 μL anti-GR mAb (1
:
100) or mouse IgG1 (1
:
100) for 60 min, washed twice in the permeabilisation buffer, and then incubated with 100 μL permeabilisation buffer containing goat anti-mouse IgG-PE (Caltag, Burlingame, CA, USA) for 60 min. After extensive washing with the permeabilisation buffer to remove unbound secondary antibodies, cells were resuspended in 500 μL fixation buffer. Cells were run on a FACS Calibur flow cytometer.24,25 The relative quantity of GR protein (mean fluorescence) was expressed as MFI.
Resuspended lymphocytes in PB and BALF of rats or CEM-C7 and CEM-C1 cells were washed with PBS and resuspended in 100 μL PBS containing 2 × 10−5 M FITC-Dex (Molecular Probe®, Invitrogen, Carlsbad, CA, USA) for 60 min at 37 °C in the dark with gentle mixing every 10 min. As controls, another tube was prepared adding a 500-fold excess amount of unlabelled Dex 10 min before FITC-Dex. Finally, cells were washed twice and resuspended in 300 μL fixation buffer. Cell samples were run on a FACS Calibur flow cytometer.26 The relative quantity of GR binding (mean fluorescence) was expressed as MFI.
2.5. Immunofluorometric assay (IF)
CEM-C7 and CEM-C1 cells were fixed with 4% paraformaldehyde at room temperature. For staining, the cells were rinsed three times with PBS (3 min each time). After the final centrifugation, the cells were slowly suspended by retaining about 50 μL of liquid drip onto the slide, which was precooled for 10 min at −20 °C to, as far as possible, evenly distribute the cells. The slide was baked for about 10 min and blocked by 2% BSA. Then, the cell was incubated with anti-GRα or GRβ antibodies (Abcam, Cambridge, UK) at a concentration of 1
:
50 at 4 °C overnight. After washing, the cells were incubated with FITC-conjugated goat anti-rabbit IgG (1
:
200 dilution) at room temperature for 1 h.27
2.6. Immunohistochemistry assay (IHC)
GRα, GRβ and HSP90 were identified in paraffin-embedded sections of the lung tissue by IHC staining with anti-GRα antibody, anti-GRβ antibody or HSP90 antibody (Abcam, Cambridge, UK) overnight at 4 °C at a concentration of 1
:
100 (GRα and HSP90) or 1
:
200 (GRβ), followed by standard biotin-streptavidin-peroxidase immunostaining using a streptavidin-peroxidase kit (Zhong Shan Golden Bridge Biotechnology Co., Ltd., Beijing, China) according to the instructions provided by the manufacturer. Staining was completed by incubation with diaminobenzidine chromogen solution at room temperature. All measurements were performed with a Nikon ECLIPSE 80i biomicroscope and NIS-Elements BR 3.2 image analysis system. Three random images within a lung sample were recorded and further analyzed using zoomed-in field at 400× magnification. We measured the integral optical density (IOD) of GRα, GRβ and HSP90-positive cells under each examined field, and calculated the average number as the final result of the sample.
2.7. Western blotting analysis
Rat lung tissues were lysed on ice in RIPA lysis buffer with protease inhibitor cocktail for 15 min to extract total protein. Concentration of the protein was quantified by a quantitative bromochloroacetate (BCA) protein kit (Beijing Biosynthesis Biotechnology Co., Ltd., Beijing, China). The protein was mixed with loading buffer and boiled at 95 °C for 5 min to make it denatured. Equal amount of protein (30 μg per lane) was separated by 8% sodium dodecyl sulfatepolyacrylamide gel electrophoresis (SDS-PAGE) and transferred into 0.45 μm polyvinylidene fluoride (PVDF) membranes. After blocking with 5% nonfat-dried milk at room temperature for 2 h, the membranes were incubated overnight at 4 °C with primary antibodies: rabbit polyclonal to HSP90 antibody (1
:
5000), rabbit polyclonal to GRα antibody (1
:
2000), rabbit polyclonal to GRβ antibody (1
:
2000) and mouse monoclonal to β-actin antibody (1
:
2000). Membranes were detected with horseradish peroxidase-labeled goat anti-rabbit IgG (1
:
20
000) or goat anti-mouse IgG (1
:
40
000) as the secondary antibody for 1 h. The protein band was visualized by an electrochemiluminescent (ECL) reagent and exposed to X-film. β-Actin was used for normalization. The sum-density of each protein band was measured by Image J software (National Institutes of Health, USA).
2.8. Quantitative real-time PCR (qPCR)
Total RNA was isolated from the lung tissue using TRIzol reagent (Life Technologies, Carlsbad, CA, USA) according to the manufacturer's recommendations, followed by purification with an RNeasy kit (Qiagen, Valencia, CA, USA) according to the manufacturer's manual. M-MLV reverse transcription was used to synthesize complementary DNA (cDNA). The qPCR amplification was performed using the SYBR-green detection of PCR products in real time with an ABI-7500 sequence detection system (Applied Biosystems, Foster City, CA, USA). The primers used in the qPCR analysis are presented in Table 1. The PCR program was performed for 40 cycles with each cycle consisting of 5 min of predenaturation at 94 °C, 15 s of denaturation at 94 °C, and 30 s of annealing at 60 °C. The melting curves for each PCR reaction were generated to ensure the purity of the amplification product. A no-template negative control was included in each experiment. Gene expression was quantified by means of the comparative Ct method (ΔΔCt) and the relative quantification (RQ) was calculated as 2−ΔΔCt. Relative mRNA levels of GRα, GRβ and HSP90 were examined and normalized to 18S mRNA expression in each sample.
Table 1 Primers used for qPCR analysis
Primer |
Forward primer |
Reverse primer |
HSP90 |
CCTGGGAAGCCCCCG |
TTGTAGACATGAGCAGAGAGCC |
GRα |
GCGACAGAAGCAGTTGAGTCATC |
CCATGCCTCCACGTAACTGTTAG |
GRβ |
GCGCTTGAGGCTAAGATAGCT |
CCCATGTTTCTGCCTCTTTCTTTG |
18S |
CCGGTACAGTGAAACTGCGA |
GATAAATGCACGCGTTCCCC |
2.9. Statistical analysis
Results of all measurements were presented as standard deviation (SD). The data analysis was performed using the SPSS 13.0 software (SPSS Inc., Chicago, USA). All of the data were tested and cleared for normality using the Kolmogorov-Smirnov test. A one-way analysis of variance (ANOVA) was performed to determine whether there were statistically significant differences (P < 0.05) among the experimental groups. The least significant difference (LSD) test when the variances were equal or Tamhane's T2 test when the variances were unequal was used for comparisons between individual groups and to determine the means that differed statistically significantly (P < 0.05).
3. Results
3.1. Combination of icariin and OA sensitizes GC-resistant CEM-C1 cells to Dex-induced apoptosis
GCs are well recognized to negatively impact the development and function of T cells in the immune system by inducing apoptosis.28 This study investigated Dex-induced apoptosis in both GC-sensitive CEM-C7 and GC-resistant CEM-C1 cells by FCM. As shown in Fig. 1, compared with four drug control treatments, Dex, icariin, OA and icariin&OA, co-administration (combination of Dex and icariin&OA) increased the apoptotic rate from 26.43% (Dex), 10.87% (icariin), 12.01% (OA), and 17.98% (icariin&OA) to 39.33% in CEM-C7 cells, and from 4.90% (Dex), 6.92% (icariin), 7.31% (OA), and 8.70% (icariin&OA) to 30.13% in CEM-C1 cells (all P < 0.01). These results indicated that combination of icariin and OA augmented Dex-induced apoptosis not only in GC-sensitive CEM-C7 cells, but also in GC-resistant CEM-C1 cells.
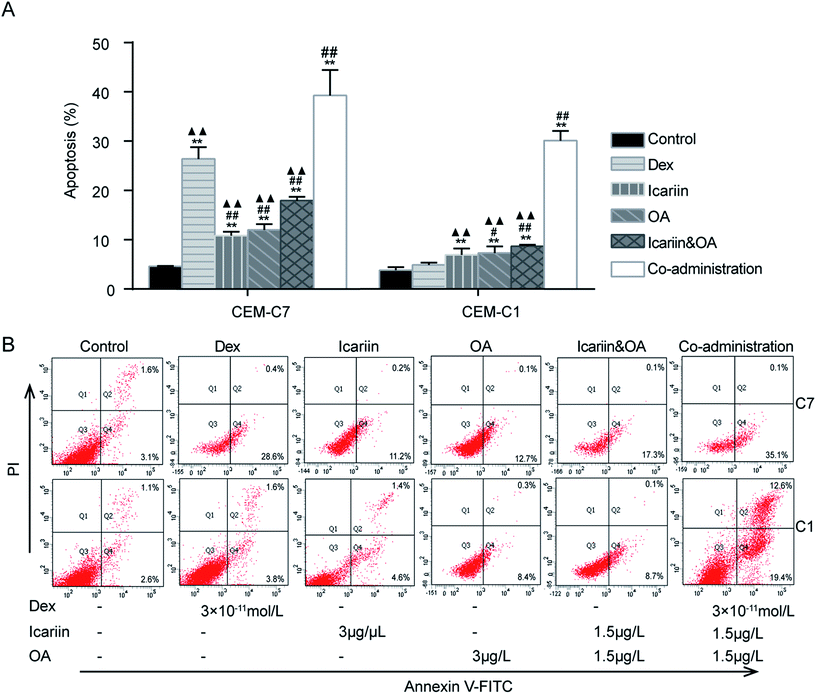 |
| Fig. 1 Combination of icariin and oleanolic acid (OA) enhanced dexamethasone (Dex)-induced apoptosis in both GC-sensitive CEM-C7 and GC-resistant CEM-C1 cells. The percentage of apoptotic cells was determined by Annexin V-FITC/PI staining and flow cytometry (FCM). (A) Levels of apoptosis rates in both GC-sensitive CEM-C7 and GC-resistant CEM-C1 cells. Percentages of each gated fraction (%) are marked on the figures. (B) Representative photographs of apoptosis by FCM. Mean ± SD, n = 3. **P < 0.01 vs. control; #P < 0.05, ##P < 0.01 vs. Dex; ▲▲P < 0.01 vs. co-administration. | |
3.2. Combination of icariin and OA modulates Dex-induced lymphocyte apoptosis in asthmatic rats
GCs have been used for decades to therapeutically modulate inflammation. Both anti-inflammatory effect and the side effects of GCs are related to inducing apoptosis in autologous mixed lymphocytes.29,30 Therefore, the level of lymphocyte apoptosis could indicate the sensitivity of anti-inflammatory effects of GCs. In this study, FCM was performed to determine lymphocyte apoptosis in PB and BALF with TUNEL assay. Rats in Dex group presented higher lymphocyte apoptosis in PB than those in the control or asthma groups (P < 0.05). Combination of icariin and OA could effectively reduce lymphocyte apoptosis in PB to nearly normal levels (P < 0.05). The results indicated that co-administration of the herbal ingredients and Dex could protect blood lymphocyte from excessive apoptosis (Fig. 2).
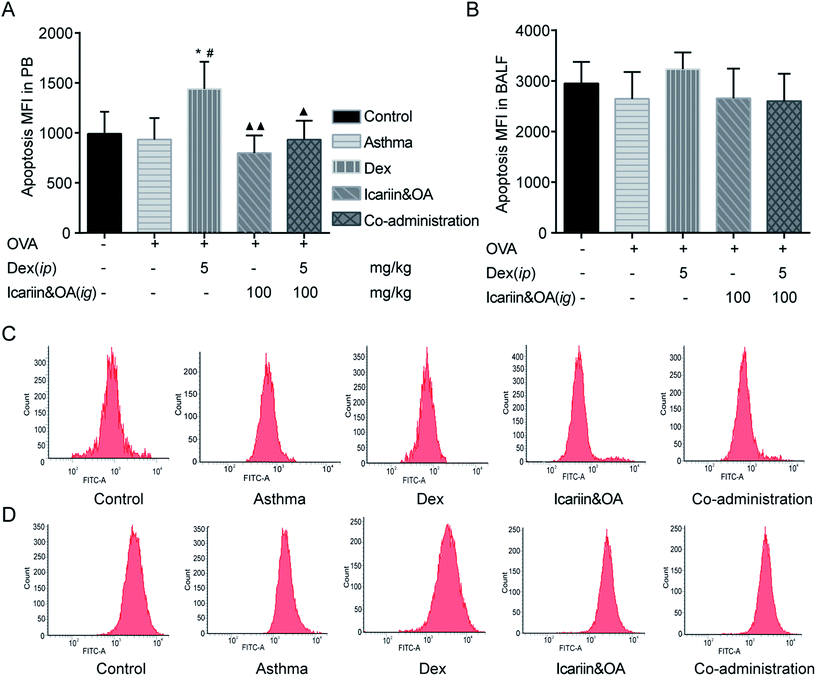 |
| Fig. 2 Combination of icariin and oleanolic acid (OA) regulated dexamethasone (Dex)-induced lymphocyte apoptosis in asthmatic rats. Viability of the levels of lymphocyte apoptosis in peripheral blood (PB, A) and bronchoalveolar lavage fluid (BALF, B) were detected by flow cytometry (FCM) with TUNEL assay and expressed as mean fluorescence intensity (MFI). Representative photographs of lymphocyte apoptosis in PB (C) and BALF (D) by FCM. Mean ± SD, n = 6. *P < 0.05 vs. control; #P < 0.05 vs. asthma; ▲P < 0.05, ▲▲P < 0.01 vs. Dex. | |
3.3. Combination of icariin and OA augmented Dex-induced activation of GR in CEM-C7 and CEM-C1 cells
The up-regulation of GR has been suggested as an essential step in GC-induced apoptosis of many types of cells.31 The abnormalities of GR seem to be related to GCR.32 With anti-GR mAb and FITC-labelled Dex, FCM was used to assess GR protein expression and GR binding in both GC-sensitive CEM-C7 and GC-resistant CEM-C1 cells. As shown in Fig. 3, GR protein greatly increased, while GR binding was not differential after Dex administration in both GC-sensitive CEM-C7 and GC-resistant CEM-C1 cells, indicating that the sufficiency of GR content could not induce the corresponding function of GC. Compared with Dex, GR protein and GR binding in the co-administration group significantly increased in both types of cells (P < 0.01), which indicated that co-administration of the herbal ingredients and Dex markedly augmented Dex-induced activation of GR.
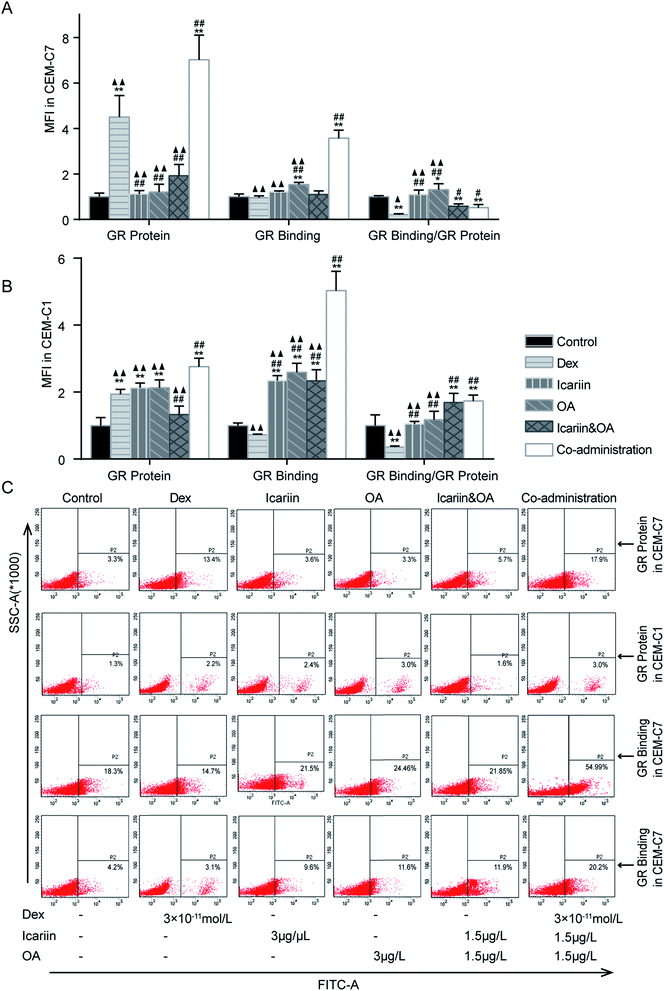 |
| Fig. 3 Combination of icariin and oleanolic acid (OA) augmented dexamethasone (Dex)-induced activation of GR in CEM-C7 and CEM-C1 cells. GR protein and GR binding were detected by flow cytometry (FCM) and expressed as mean fluorescence intensity (MFI). Fold changes of GR protein, GR binding and GR binding/GR protein relative to control group in CEM-C7 cell (A) and CEM-C1 cell (B). (C) Representative photographs of GR protein and GR binding in CEM-C7 and CEM-C1 cells; the percentage of positive cells of GR protein and FITC-dexamethasone (Dex) was determined relative to total cells. Mean ± SD, n = 3. *P < 0.05, **P < 0.01 vs. control; #P < 0.05, ##P < 0.01 vs. Dex; ▲P < 0.05, ▲▲P < 0.01 vs. co-administration. | |
3.4. Effect of combination of icariin and OA on Dex-induced activation of GR in asthmatic rats
We further confirmed the effects of the herbal ingredients on Dex-induced activation of GR in asthmatic rats. As shown in Fig. 4A, GR protein and GR binding in PB lymphocytes greatly decreased following repeated Dex administration vs. control group (P < 0.01), indicating insufficiency of GR function. Compared with the Dex group, significant increase in GR protein and GR binding were noticed in the co-administration group (P < 0.05 or P < 0.01), which indicated that co-administration of Dex and the herbal ingredients could markedly augment Dex-induced activation of GR in PB lymphocytes. As shown in Fig. 4B, GR binding in BALF lymphocytes remarkably decreased (P < 0.01), while GR protein could not be differentiated after repeated Dex administration compared with that in the control or asthma groups. Significant increase in GR binding of BALF lymphocyte was noticed in the co-administration group vs. Dex group (P < 0.01), indicating that co-administration of Dex and the herbal ingredients could markedly increase the binding capacity between GC and GR to protect Dex-induced impairment of GR activation.
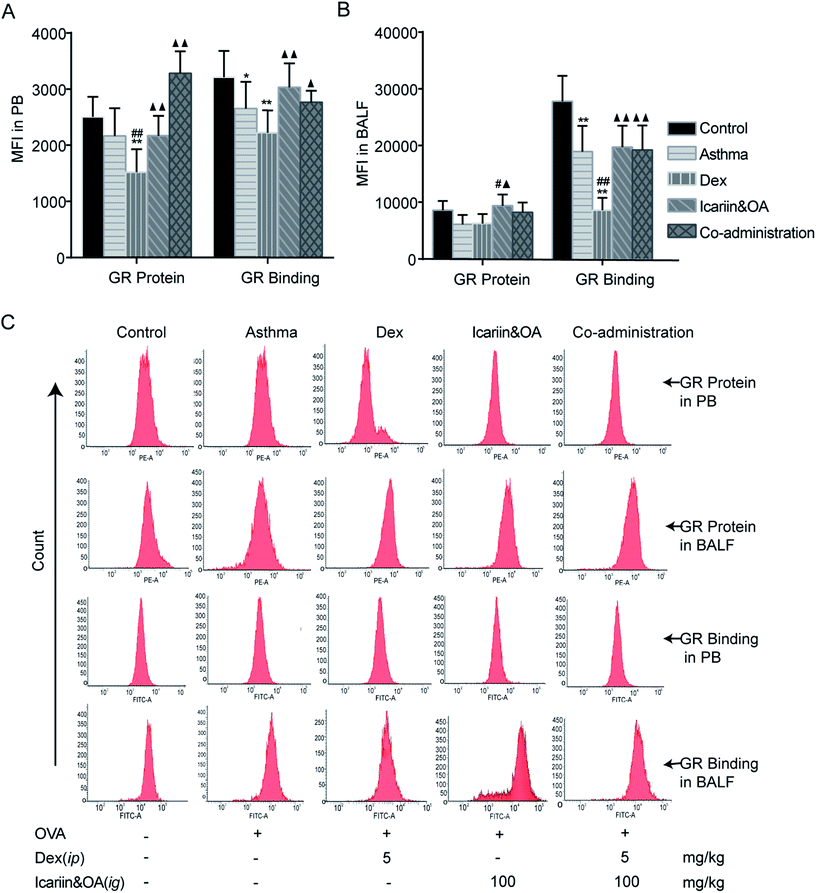 |
| Fig. 4 Effect of combination of icariin and oleanolic acid (OA) on dexamethasone (Dex)-induced activation of GR in asthmatic rats. Levels of GR protein and GR binding in lymphocytes of peripheral blood (PB, A) and bronchoalveolar lavage fluid (BALF, B) by flow cytometry (FCM) and expressed as mean fluorescence intensity (MFI). (C) Representative photographs of GR protein and GR binding in lymphocyte of PB and BALF by FCM. Mean ± SD, n = 6. *P < 0.05, **P < 0.01 vs. control; #P < 0.05, ##P < 0.01 vs. asthma; ▲P < 0.05, ▲▲P < 0.01 vs. Dex. | |
3.5. Combination of icariin and OA regulates Dex-induced GR isoforms in CEM-C7 and CEM-C1 cells
In the processes of alternative splicing of GR, 2 major mRNA isoforms, GRα and GRβ, are generated. GRα is ubiquitously expressed and is responsible for the induction and repression of target genes. GRβ acts as a dominant negative inhibitor of GRα-mediated transactivation and transrepression. Increased expression of GRβ, which competes with and thus inhibits activated GRα, can cause GC resistance.33 In this study, protein expressions of GRα and GRβ in both GC-sensitive CEM-C7 and GC-resistant CEM-C1 cells were tested by IF, and an altered ratio of GRβ to GRα was calculated to evaluate the regulation effect of combination of icariin and OA on Dex-induced GR isoforms. As shown in Fig. 5, GRα protein in the CEM-C7 cells significantly increased and GRβ protein in the CEM-C1 cells significantly increased after Dex administration vs. control group; then, the ratio of GRβ to GRα decreased in the CEM-C7 cells and increased in the CEM-C1 cells. This was consistent with the conclusion that GRβ can cause GCR. Compared with the Dex group, co-administration treatment increased GRα protein in both GC-sensitive CEM-C7 and GC-resistant CEM-C1 cells (P < 0.01) and decreased GRβ protein and ratio of GRβ to GRα in the CEM-C1 cells. These results suggest that combination of icariin and OA rebalances Dex-induced GRβ/GRα in GC-sensitive CEM-C7 and GC-resistant CEM-C1 cells.
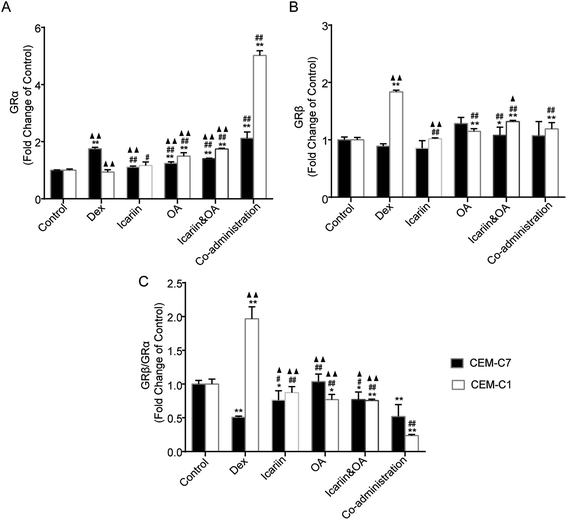 |
| Fig. 5 Combination of icariin and oleanolic acid (OA) regulated dexamethasone (Dex)-induced GR isoforms in both GC-sensitive CEM-C7 and GC-resistant CEM-C1 cells. Fold changes of GRα protein (A), GRβ protein (B) and GRβ/GRα (C) relative to control group in CEM-C7 and CEM-C1 cells by immunofluorometric assay. Mean ± SD, n = 3. *P < 0.05, **P < 0.01 vs. control; #P < 0.05, ##P < 0.01 vs. Dex; ▲P < 0.05, ▲▲P < 0.01 vs. co-administration. | |
3.6. Combination of icariin and OA regulates Dex-induced GR isoforms in asthmatic rats
Protein and mRNA expressions of GRα and GRβ in the lung tissue of asthmatic rats were tested by IHC, Western blotting and qPCR. After repeated Dex administration, GRα protein and mRNA significantly decreased (P < 0.05 or P < 0.01), while GRβ protein and mRNA increased vs. that in control group (P < 0.05 or P < 0.01). Compared with the Dex group, significant increments were noticed in GRα mRNA and protein and decrements were noticed in GRβ mRNA and protein in the co-administration group (P < 0.05 or P < 0.01). These results suggest that combination of icariin and OA regulates GR isoforms in the rat asthmatic model with the treatment of Dex. (Fig. 6)
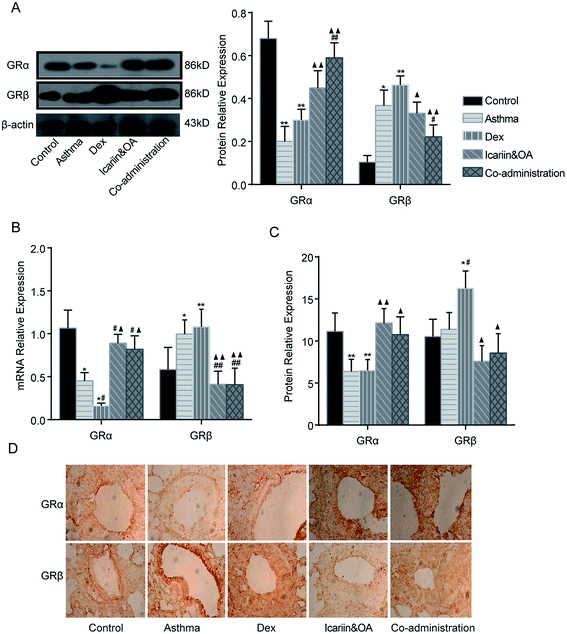 |
| Fig. 6 Combination of icariin and oleanolic acid (OA) regulated dexamethasone (Dex)-induced GR isoforms in asthmatic rats. (A) GRα and GRβ proteins in lung tissues of the rat asthmatic model were detected by western blotting. (B) mRNA expressions of GRα and GRβ in lung tissues of the rat asthmatic model were detected by quantitative real-time PCR (qPCR). (C) GRα and GRβ proteins in lung tissues of the rat asthmatic model were detected by immunohistochemistry assay (IHC). (D) Representative photographs of GRα and GRβ protein expression in lung tissues by IHC. Mean ± SD, n = 6. *P < 0.05, **P < 0.01 vs. control; #P < 0.05, ##P < 0.01 vs. asthma; ▲P < 0.05, ▲▲P < 0.01 vs. Dex. | |
3.7. Combination of icariin and OA up-regulates Dex-induced HSP90 in asthmatic rats
HSP90 associates selectively with unliganded GR, and the appropriate folding of GR depends on direct interactions with the chaperone, HSP90.34 Dissociation of GR-associated proteins accompanies hormone binding and leads to the exposure of its various functional domains. Although an association with HSP90 presumably masks the GR nuclear localization signal sequence, the recent demonstration of the co-import of GR and HSP90 into cell nuclei has led to the hypothesis that HSP90 facilitates GR interactions with the nuclear transport machinery. To gain insight into the mechanisms of GC regulation in a physiological context, the level of HSP90 in the lung tissues of asthmatic rats was examined by IHC, Western blotting and qPCR. The plot in Fig. 7 shows that the expressions of HSP90 protein and mRNA in the Dex group significantly decreased as compared to the control group (P < 0.05 or P < 0.01). In addition, compared to the Dex group, the expressions of protein and mRNA of HSP90 in the co-administration group significantly increased (P < 0.05 or P < 0.01). It could be inferred that the regulation mechanisms of the herbal ingredients on GC sensitivity were associated with the up-regulation of HSP90 expression.
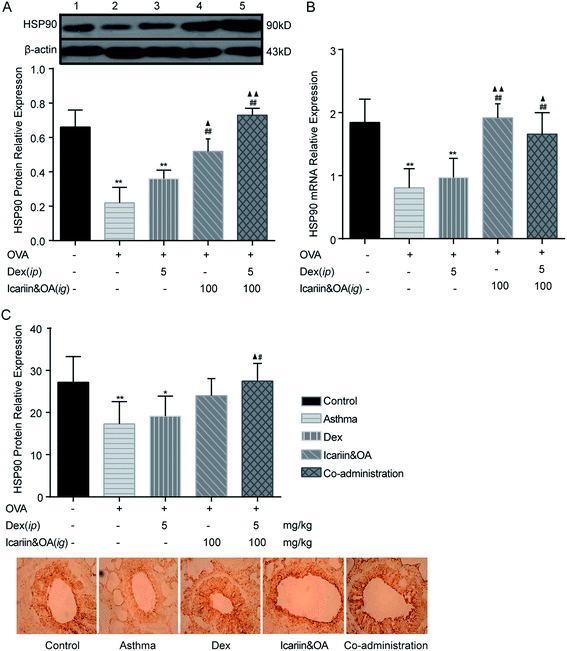 |
| Fig. 7 Combination of icariin and oleanolic acid (OA) up-regulated dexamethasone (Dex)-induced HSP90 in asthmatic rats. (A) HSP90 protein in lung tissues of the rat asthmatic model was detected by western blotting. (B) HSP90 mRNA in lung tissues of the rat asthmatic model was detected by quantitative real-time PCR (qPCR). (C) HSP90 protein in lung tissues of the rat asthmatic model was detected by immunohistochemistry assay (IHC). 1 = control; 2 = asthma; 3 = Dex; 4 = icariin&OA; 5 = Co-administration. Mean ± SD, n = 6. *P < 0.05, **P < 0.01 vs. control; #P < 0.05, ##P < 0.01 vs. asthma; ▲P < 0.05, ▲▲P < 0.01 vs. Dex. | |
4. Discussion
Because GCR occurs in multiple inflammatory and autoimmune diseases, it is widely accepted that GCR is a heterogeneous phenomenon with many underlying mechanisms.35 Many different molecular mechanisms of GCR have now been elucidated, but GR protein down-regulation can be the result of many different mechanisms of GCR.7 In our previous research, we demonstrated that the decoction and active ingredients (total flavonoids and triterpenoids) of EF and LLF could prevent the decrease of mRNA and protein expression of GR induced by Dex or Bun in asthmatic rats.14,15 We speculate that combination of icariin and OA, agents extracted from EF and LLF, respectively, has the function of protecting GR. Icariin possesses extensive therapeutic effects, such as protecting neurons from injury, regulating nuclear receptors, improving sexual dysfunction and bone morphogenesis, as well as anti-inflammation, anti-tumor and anti-depression functions.36 OA can protect against chemically induced liver injury and exhibits anti-inflammatory and anti-hyperlipidemic properties, anti-tumor promotion, toxicant activation inhibition and body defense system enhancement.37,38 However, combination of icariin and OA, as an agent to revert GCR, has not been explored yet.
Lymphocytes, particularly T cells, are the best candidates for cellular and molecular targets of GCs. GCs induce apoptosis in T cells and are potent suppressors of cytokine production in T cells.39 In addition, GCs stimulates apoptosis in all B-cell developmental subsets.40 Several animal models have been produced that have deleted the GR specifically in T cells, rendering them resistant to GC-induced apoptosis and elucidating the role of GC signaling in T-cell development.27,41 Our results show that combination of icariin and OA sensitized GC-resistant CEM-C1 cells to Dex-induced apoptosis. Interestingly, combination of icariin and OA has no influence on Dex-induced lymphocyte apoptosis of BALF in asthmatic rats after repeated Dex administration while effectively reducing lymphocyte apoptosis in PB. It is possible that complex microenvironmental factors influence the sensitivity of GCs to lymphocytes in vivo.
GC insensitivity can be primarily caused by problems in the GR protein itself. Alterations in the GR expression that mediate GC actions are one of the potential mechanisms that would explain reduced sensitivity of GC. Qualitative and quantitative abnormalities in GR binding to GCs have been described in the lymphocytes of patients with GC-resistant asthma or even in asthma in general.42 Reduction of GR protein and mRNA expression in the lungs of asthmatic mice has been reported.43 In the present study, we found that Dex induced significant increase in GR protein in GC-sensitive CEM-C7 and GC-resistant CEM-C1 cells, with the former being much stronger. However, no significant up-regulation of GR binding was noticed in the CEM-C7 and CEM-C1 cells. In vivo, GR protein in PB and GR binding in PB and BALF significantly decreased in asthmatic rats treated with Dex, which suggested that repeated Dex administration could induce GC insensitivity. Combination of icariin and OA could clearly increase GR protein and GR binding in vivo and in vitro to improve the sensitivity to GC.
GR gene expresses 2 splicing isoforms depending on the use of alternative exons 9α or 9β.44 A less-abundant GRβ has a truncated ligand binding domain, does not bind GCs, and is found to be a dominant negative inhibitor of classic GRα.45 An association between GC insensitivity and increased GRβ expression has been reported in asthma and other diseases.46,47 It has been proposed that GCR could result from the imbalance between GRα and GRβ, though there is a lack of information on whether the GRα/GRβ ratio determines the level of GC responsiveness.48 In addition, it has been shown that GRβ does not interfere with GR-mediated transrepression and excess GRα appears to overcome its inhibitory action.49 In the present study, we found that Dex induced a significant increase in GRα protein in the CEM-C7 cells, while in the CEM-C1 cells, Dex induced a significant increase in GRβ protein. Then, the ratio of GRβ to GRα decreased in the CEM-C7 cells and increased in the CEM-C1 cells. In vivo, GRα protein and mRNA significantly decreased, while GRβ increased in asthmatic rats after treatment with Dex for 21 days. It is suggested that repeated Dex administration can increase GRβ expression, further inhibiting the function of GRα, and lead to a decrease in GC sensitivity or an increase in the possibility of GCR. Combination of icariin and OA with Dex increased GRα expression and decreased GRβ expression, so that the imbalance of GRβ/GRα could be corrected in vivo and in vitro. It could be deduced that the possible mechanism of combination of icariin and OA to revert GCR is related to adjusting GR isoforms, including up-regulating the mRNA and protein expressions of GRα, down-regulating the mRNA and protein expressions of GRβ to suppress the combination between GC and GRα, and restoring the appropriate ratio of GRβ/GRα.
In the cytoplasm, the inactive form of GR appears to be bound to HSP90. This interaction facilitates binding of GC to GR and depends on the relative amounts of the interacting components, GR and HSP90. HSP90 content can confer tissue specificity and sensitivity to GCs.50,51 Abnormal expression of HSP90 leads to the inhibition of GR translocation to the nucleus and reduction in its transcription.52 To gain insight into the mechanisms of GC regulation in a physiological context, the level of HSP90 in lung tissues of asthmatic rats was examined. We found that HSP90 protein and mRNA significantly decreased in the asthmatic rats after repeated Dex administration, and the combination of icariin and OA up-regulated Dex-induced HSP90 inhibition. It could be inferred that the regulation mechanisms of the herbal ingredients on GCR were associated with the up-regulation of HSP90 expression.
5. Conclusions
In summary, our study suggests that combination of icariin and OA could revert GCR through regulating lymphocyte apoptosis and GR isoforms and augmenting GR binding and HSP90 expression, and this could be a valuable and affordable approach for GCR in the future.
Abbreviations
BALF | Bronchoalveolar lavage fluid |
Dex | Dexamethasone |
EF | Epimedii folium |
FCM | Flow cytometry |
FITC | Fluorescein isothiocyanate |
GC | Glucocorticoid |
GCR | Glucocorticoid resistance |
GR | Glucocorticoid receptor |
HSP90 | Heat shock protein 90 |
Ig | Intragastric administration |
IHC | Immunohistochemistry |
IOD | Integral optical density |
Ip | Intraperitoneal injection |
LLF | Ligustri lucidi fructus |
MFI | Mean fluorescence intensity |
OA | Oleanolic acid |
OVA | Ovalbumin |
PB | Peripheral blood |
PE | Phycoerythrin |
qPCR | Quantitative real-time PCR |
TCM | Traditional Chinese medicine |
TUNEL | TdT–dUTP terminal nick-end labeling |
Author contributions
Renhui Liu and Xiufeng Tang wrote the manuscript. Xiufeng Tang, Xiaoxi Li, Yuheng Chen, Yingying Gao and Ping Yu performed the experiments. Liping Xu and Renhui Liu conceived and designed the experiments.
Conflicts of interest
The authors declare no conflict of interest.
Acknowledgements
This research was supported by Natural Science Foundation of China (Grant No. 81373814 and 81673993), the Development of High-Caliber Talents Project of Beijing Municipal Institutions (Grant No. CIT&TCD201504097), and the Planned Project on Beijing Traditional Chinese Medicine Inheritance of “3 + 3 Programme” of the Beijing Chinese Medicine Administration Bureau (Grant No. 2012-SZ-C-42).
References
- J. A. Beach, L. J. Nary, R. Hovanessian and R. D. Medh, Biochem. Biophys. Res. Commun., 2014, 451, 382–388 CrossRef CAS PubMed
. - K. Dendoncker and C. Libert, Cytokine Growth Factor Rev., 2017, 35, 85–96 CrossRef CAS PubMed
. - C. Heuck, O. D. Wolthers, M. Hansen and G. Kollerup, Steroids, 1997, 62, 659–664 CrossRef CAS PubMed
. - E. W. Zöllner, C. Lombard, U. Galal, S. Hough, E. Irusen and E. J. Weinberg, Pediatr. Endocrinol. Metab., 2011, 24, 529–534 Search PubMed
. - K. N. Priftis, A. Papadimitriou, M. B. Anthracopoulos, A. Fretzayas and G. P. Chrousos, NeuroImmunoModulation, 2009, 16, 333–339 CrossRef CAS PubMed
. - P. J. Barnes and I. M. Adcock, Lancet, 2009, 373, 1905–1917 CrossRef CAS
. - M. Zen, M. Canova, C. Campana, S. Bettio, L. Nalotto, M. Rampudda, R. Ramonda, L. Iaccarino and A. Doria, Autoimmun. Rev., 2011, 10, 305–310 CrossRef CAS PubMed
. - S. Schmidt, J. Rainer, C. Ploner, E. Presul, S. Riml and R. Kofler, Cell Death Differ., 2004, 11, S45–S55 CrossRef CAS PubMed
. - M. J. Schaaf and J. A. Cidlowski, J. Steroid Biochem. Mol. Biol., 2002, 83, 37–48 CrossRef CAS PubMed
. - K. Pazdrak, C. Straub, R. Maroto, S. Stafford, W. I. White, W. J. Calhoun and A. Kurosky, J. Immunol., 2016, 197, 3782–3791 CrossRef CAS PubMed
. - P. J. Barnes, Handb. Exp. Pharmacol., 2017, 237, 93–115 Search PubMed
. - T. L. Green, S. M. Leventhal, D. Lim, K. Cho and D. G. Greenhalgh, Shock, 2017, 47, 148–157 CrossRef CAS PubMed
. - A. D. Wallace and J. A. J. Cidlowski, Biol. Chem., 2001, 276, 42714–42721 CrossRef CAS PubMed
. - R. D. Medh, M. S. Webb, A. L. Miller, B. H. Johnson, Y. Fofanov, T. Li, T. G. Wood, B. A. Luxon and E. B. Thompson, Genomics, 2003, 81, 543–555 CrossRef CAS PubMed
. - T. D. Hinds, B. Peck, E. Shek, S. Stroup, J. Hinson, S. Arthur and J. S. Marino, Int. J. Mol. Sci., 2016, 17, 232 CrossRef PubMed
. - P. Wang, L. P. Xu, X. H. Jin, J. Yang, R. H. Liu and X. J. Wang, Zhongxiyi Jiehe Xuebao, 2010, 8, 80–85 CAS
. - R. H. Liu, X. J. Wang, W. H. Zhang, Y. Yuan and P. Wang, China J. Tradit. Chin. Med. Pharm., 2013, 28, 3215–3219 CAS
. - X. Tang, X. Li, H. Nian, Y. Yang, Y. Chen, X. Wang, L. Xu, X. Yang and R. Liu, Evidence-Based Complementary Altern. Med., 2017, 2017, 7961231 Search PubMed
. - K. Wei, Y. Xu, Z. Zhao, X. Wu, Y. Du, J. Sun, T. Yi, J. Dong and B. Liu, Int. J. Mol. Med., 2016, 38, 337–344 CrossRef CAS PubMed
. - B. Liu, H. Zhang, C. Xu, G. Yang, J. Tao, J. Huang, J. Wu, X. Duan, Y. Cao and J. Dong, Brain Res., 2011, 1375, 59–67 CrossRef CAS PubMed
. - S. S. Bachhav, S. D. Patil, M. S. Bhutada and S. J. Surana, Phytother. Res., 2011, 25, 1435–1439 CrossRef CAS PubMed
. - T. B. Ayeleso, M. G. Matumba and E. Mukwevho, Molecules, 2017, 22, 232 CrossRef PubMed
. - Y. Liu, J. Ge, Q. Li, X. Guo, L. Gu, Z. G. Ma, X. H. Li and Y. P. Zhu, Leuk. Lymphoma, 2014, 55, 2179–2188 CrossRef CAS PubMed
. - A. R. Shibata, E. J. Troster and H. R. Wong, Pediatric critical care medicine, 2015, 16, e132–e140 CrossRef PubMed
. - M. Bergquist, F. Huss, J. Hästbacka, C. Lindholm, C. Martijn, C. Rylander, G. Hedenstierna and F. Fredén, Acta Anaesthesiol. Scand., 2016, 60, 213–221 CrossRef CAS PubMed
. - J. Du, M. Li, D. Zhang, X. Zhu, W. Zhang, W. Gu, Y. Feng, X. Zhai and C. Ling, Arthritis Res. Ther., 2009, 11, R108 Search PubMed
. - X. Yang, W. Yao, H. Liu, Y. Gao, R. Liu and L. Xu, Sci. Rep., 2017, 7, 1014 CrossRef PubMed
. - A. L. Gruver-Yates, M. A. Quinn and J. A. Cidlowski, Endocrinology, 2014, 155, 463–474 CrossRef PubMed
. - M. J. Olnes, Y. Kotliarov, A. Biancotto, F. Cheung, J. Chen, R. Shi, H. Zhou, E. Wang, J. S. Tsang, R. Nussenblatt and CHI Consortium, Sci. Rep., 2016, 6, 23002 CrossRef CAS PubMed
. - P. J. Chang, C. Michaeloudes, J. Zhu, N. Shaikh, J. Baker, K. F. Chung and P. K. Bhavsar, Am. J. Respir. Crit. Care Med., 2015, 191, 54–62 CrossRef CAS PubMed
. - A. L. Miller, M. S. Webb, A. J. Copik, Y. Wang, B. H. Johnson, R. Kumar and E. B. Thompson, Mol. Endocrinol., 2005, 19, 1569–1583 CrossRef CAS PubMed
. - K. Oneda, Arerugi, 1999, 48, 13–22 CAS
. - C. A. Butler, S. McQuaid, C. C. Taggart, S. Weldon, R. Carter, G. Skibinski, T. J. Warke, D. F. Choy, L. P. McGarvey, P. Bradding, J. R. Arron and L. G. Heaney, Thorax, 2012, 67, 392–398 CrossRef PubMed
. - X. Qian, Y. Zhu and W. Xu, Zhongxiyi Jiehe Xuebao, 2001, 81, 1496–1499 CAS
. - Y. Matsumura, Int. J. Biomed. Sci., 2010, 6, 158–166 CAS
. - Y. Chen, J. H. Huang, Y. Ning and Z. Y. Shen, Zhongxiyi Jiehe Xuebao, 2011, 9, 1179–1184 CAS
. - L. Žiberna, D. Šamec, A. Mocan, S. F. Nabavi, A. Bishayee, A. A. Farooqi, A. Sureda and S. M. Nabavi, Int. J. Mol. Sci., 2017, 18, pii: E643 CrossRef PubMed
. - C. Lin, X. Wen and H. Sun, Expert Opin. Ther. Pat., 2016, 26, 643–655 CrossRef CAS PubMed
. - R. M. Evans, Mol. Endocrinol., 2005, 19, 1429–1438 CrossRef CAS PubMed
. - A. L. Gruver-Yates, M. A. Quinn and J. A. Cidlowski, Endocrinology, 2014, 155, 463–474 CrossRef PubMed
. - M. J. Herold, K. G. McPherson and H. M. Reichardt, Cell. Mol. Life Sci., 2006, 63, 60–72 CrossRef CAS PubMed
. - P. Demoly, M. Mathieu, J. Bousquet, F. B. Michel and P. Godard, Presse Med., 1996, 25, 769–774 CAS
. - M. T. Bailey, S. G. Kinsey, D. A. Padgett, J. F. Sheridan and B. Leblebicioglu, Physiol. Behav., 2009, 98, 351–358 CrossRef CAS PubMed
. - N. Z. Lu and J. A. Cidlowski, Ann. N. Y. Acad. Sci., 2004, 1024, 102–123 CrossRef CAS PubMed
. - M. R. Yudt, C. M. Jewell, R. J. Bienstock and J. A. Cidlowski, Mol. Cell. Biol., 2003, 23, 4319–4330 CrossRef CAS PubMed
. - L. Pujols, J. Mullol and C. Picado, Front. Biosci., Landmark Ed., 2010, 15, 789–800 CrossRef CAS
. - T. Kino, Y. A. Su and G. P. Chrousos, Cell. Mol. Life Sci., 2009, 66, 3435–3448 CrossRef CAS PubMed
. - B. Jakieła, G. Bochenek and M. Sanak, Pol. Arch. Med. Wewn., 2010, 120, 214–222 Search PubMed
. - I. J. Brogan, I. A. Murray, G. Cerillo, M. Needham, A. White and J. R. Davis, Mol. Cell. Endocrinol., 1999, 157, 95–104 CrossRef CAS PubMed
. - N. O. Vamvakopoulos, Mol. Cell. Endocrinol., 1993, 98, 49–54 CrossRef CAS PubMed
. - D. Picard, B. Khursheed, M. J. Garabedian, M. G. Fortin, S. Lindquist and K. R. Yamamoto, Nature, 1990, 348, 166–168 CrossRef CAS PubMed
. - M. Matysiak, B. Makosa, A. Walczak and K. Selmaj, Mult. Scler., 2008, 14, 919–926 CrossRef CAS PubMed
.
|
This journal is © The Royal Society of Chemistry 2018 |