DOI:
10.1039/C7RA09564C
(Paper)
RSC Adv., 2018,
8, 1427-1435
Photolysis of sulfamethazine using UV irradiation in an aqueous medium
Received
29th August 2017
, Accepted 18th December 2017
First published on 4th January 2018
Abstract
Although many studies have been focused on the photochemistry of antibiotics, the roles of reactive species in photolysis and the effects of dissolved substances on antibiotic photochemical behavior have been poorly examined. The photolytic behaviors of sulfamethazine (SMN) in pure water were investigated via adding different scavengers to quench the active species. Results showed that decomposition of the triplet-excited state of SMN (3SMN*) by direct photolysis was the main path of SMN photolysis in water. Moreover, self-sensitized SMN cannot be ignored during SMN photodegradation. The main photoproducts of SMN were identified by LC-MS/MS, which indicated that SMN could not be mineralized although the photolysis under UV was effective. The effects of Cl−, NO3−, and fulvic acid (FA) (common substances in natural water) on SMN photolytic behaviors were also studied. The triplet-induced halogenation of SMN increases the ionic strength and reduces the ground state SMN; these are the primary causes of promotion of SMN photolysis by Cl−. More ˙OH produced in the presence of NO3− could promote SMN photolysis. Competitive absorption of photons of FA with SMN and ROS scavenged by FA were the main reasons for the inhibition of SMN photolysis. The research findings are helpful for further studies on the environmental risks of ACs in natural waters and promoting the development of AC pollution treatment technology.
1. Introduction
In the last few decades, large amounts of veterinary antibiotics (ACs) have been used in animal husbandry as therapeutic medicine and feed additives for growth promotion. Moreover, one of the most popular groups of ACs, which are mainly used in livestock, are sulfonamides (SNs).1,2 In China, AC use in stock raising was nearly 92
700 tons, and usage of SNs was nearly 7920 tons in 2013.3 In the USA, 16
000 tons of ACs, including 2.3% SNs, were consumed per year. In Europe, the output of SNs ranged from 11% to 23% of the total production of ACs.4 SNs may be excreted from the body in their parent form without absorption and metabolism by animals. Because of their high stability and solubility, conventional sewage disposal technology used in livestock farming is not efficient for SN removal. The average removal ratio of SNs by activated sludge was found to be 24%,1 which meant that a mass of these drugs was introduced into the biosphere every year.1,5 In this case, ACs were detected in rivers, lakes, estuarine, and coastal waters many years ago.6–8 The highest concentration of sulfamethazine (SMN) in manure of swine farms in South China was up to 0.250 mg kg−1.9 The concentration of ACs in the aquatic environment was relatively low (ng L−1 to μg L−1),10,11 whereas its presence in AC manufacturing effluents might reach mg L−1 levels.12 The bacterial resistance and photo-modified toxicities of ACs could cause severe negative impact on human health and the environment.1,13–18 ACs have been classified as particularly dangerous pollutants for the environment.1
The obstinate nature of ACs residues interferes with the removal of these compounds from the environment by traditional biological treatments. Finding effective methods to eliminate the ACs residues is required for environmentally sustainable development. Heterogeneous photocatalysis, one of the typical advanced oxidation techniques (AOPs), is an efficient treatment to promote degradation of organic pollutants.19 Further, the performance of photocatalytic technology could be effectively improved via photocatalyst modification,20 electrochemical, microwave or ultrasonic-assisted photocatalytic technology.21–23 The efficiency of photocatalysis is based on the formation of reactive species through the irradiation of light energy on catalysts. During photocatalytic degradation of organic contaminants, photodegradation may play an important role, and photochemical decomposition has also been proven to be a major transformation pathway for organic pollutants in surface waters.24,25 Therefore, it is significant to carry out a study on photodecomposition of pollutants.
Photolysis of ACs is mainly caused by ultraviolet wavelength irradiation, and ACs can undergo not only direct or indirect photolysis, but also self-sensitized photo-oxidation.2 During direct photolysis, photon absorption promotes electrons from the initial ground state of ACs to produce electronically excited species in the singlet state (AS*) or triplet state (3AS*). On the one hand, the excited species can be decomposed into photoproducts. On the other hand, by self-sensitization, 3AS* can transfer the energy to the ground state of 3O2 or H2O to form reactive oxygen species (ROS, e.g., ˙OH and 1O2).26,27 In addition to the direct and self-sensitized photolysis, indirect photolysis is a significant elimination pathway for many aquatic pollutants; it is initiated by ROS, formed via optical absorption by photosensitizers (e.g., dissolved organic matter (DOM), Fe species, halide ions, and H2O2).28–30 However, controversy still exists about which path should play the leading role in different research. Studies indicated that many byproducts were still retained during the photolysis of antibiotics, the intermediates showed an increasing toxicity, and the solutions after photolysis still had certain residual antibacterial activity.31–35 Because the photolytic byproducts will be different due to different photolytic reaction paths of ACs, studies on the main factors for photolysis and the reaction paths of ACs under UV irradiation are helpful for a further study on the environmental risks of ACs in natural waters and promoting the development of AC pollution treatment technology.
In this study, we employed SMN as a proxy AC to investigate photolytic behavior under UV irradiation and detect the role of triplet-excited state and ROS in the photodegradation of ACs. Furthermore, the photodecomposition pathway of SMN was proposed. The influence of common dissolved substances in natural water on the photodecomposition of SMN was also discussed in this study.
2. Materials and methods
2.1 Chemicals
High purity standard SMN (99%), used as a target pollutant in photochemical experiments and as a standard in high-performance liquid chromatography (HPLC) analysis, was purchased from Aladdin Industrial Corporation (Shanghai, China). Acetonitrile and isopropanol were of HPLC grade and obtained from Chengdu Best Reagent (Chengdu, China). Sorbic acid (t,t-HDA, 99%), sodium chloride, sodium nitrate, fulvic acid (FA), and other chemical reagents were of analytical grade and obtained from Chengdu Best Reagent. Ultrapure water was used to prepare SMN solutions and HPLC eluent.
2.2 Experimental set-up
A merry-go-round photochemical reactor with a magnetic stirrer (illustrated in Fig. 1) was employed. The high pressure Hg lamp was in a quartz sleeve and located at the center of the reactor. The temperature of the reaction system was 25 °C. The 50 mL SMN solutions were put in quartz tubes around and equidistant from the Hg lamp, irradiated under UV over a period of 60 min, and aliquots were withdrawn for analysis at scheduled time intervals. To investigate the hydrolytic degree of SMN during photolysis, non-irradiated experiments were conducted simultaneously. The SMN solutions were wrapped in an aluminum foil, and other conditions were kept the same as those for the unwrapped samples. All experiments were performed in triplicate.
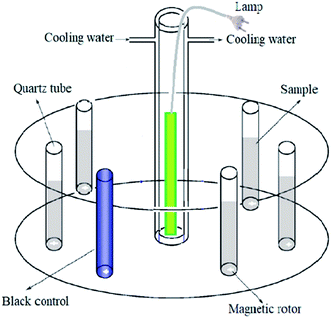 |
| Fig. 1 A schematic of the experimental setup. | |
2.3 Analytical methods
The concentration of SMN at different irradiation times was determined by HPLC (LC-2010HT, Shimadzu) coupled with UV/VIS detection (LC-UV/VIS). The parameters of the analysis were set according to the research of Batista et al.2 a C18 5 μm to 100 Å column (250 mm × 4.60 mm). The detection wavelength of SMN was found to be at 268 nm. The sample injection volume was 50.0 μL. The eluents were (A) H2O + 0.200% acetic acid and (B) acetonitrile at 80
:
20 ratio, and the flow rate was set at 1.00 mL min−1. The detection and quantification limits were 0.170 mg L−1 and 0.500 mg L−1, respectively.
The products of photolysis were identified by a Shimadzu LC-20A liquid chromatography system coupled with a Shimadzu LCMS-8030 triple quadrupole mass spectrometer (LC-MS/MS). The eluent and column used were the same as those of LC-UV/VIS, but the ratio of A and B eluents was altered during the analysis: it started with 10% of B, then it increased to 60% after 10 min, then to 90% in another 8 min, and finally the content of B dropped to 10% in another 10 min and remained at this ratio until the end of the run. The detection was performed using an electrospray ionization (ESI) source operating in the positive mode. The parameters were set as follows: capillary voltage 4000 V, drying gas temperature 300 °C, drying gas flow 12 mL min−1, and nebulization gas 35 psi. Fragmentor voltages were adjusted between 10 and 30 V to obtain precursor ions of degradation products.
The total organic carbon (TOC) was detected by a TOC analyzer (TOC4100, Shimadzu).
3. Results and discussions
3.1 The determination of initial concentration of SMN and the light source
The concentration of SMN in the aquatic environment (ng L−1 to μg L−1) is relatively low. A pre-concentration step is required prior to quantification using HPLC.36,37 Although previous research has reported that the solid phase extraction (SPE) technique can improve the sensitivity of the analytical methods by HPLC, the use of SPE for quantification of sulfonamides in aqueous samples has not been examined in detail.36 During photodegradation of ACs, different initial concentrations of ACs caused different amounts of reactant molecules to be photo-excited; this affected the rate of photolysis, but had no impact on the reaction mechanism.2,6 Furthermore, Nassar et al.38 evaluated the photodecomposition of four different ACs under irradiation of sunlight and a single wavelength UV source (254 nm). The results showed that UV irradiation was effective to degrade these drugs, and photolysis of ACs under simulated solar irradiation was caused by AC absorbing the UV light from sunlight.
Due to all the abovementioned reasons, the selected initial concentration of SMN and the light source in this study were 20 mg L−1 and a 300 W high pressure Hg lamp, respectively. Although these two parameters were significantly different from the antibiotic environment in natural water bodies, the conclusions of this study could provide theoretical reference for the photochemical behavior of antibiotic residues in natural aquatic environments.
3.2 Photolysis of SMN in pure water
To investigate the photolytic process of SMN, we explored SMN photolysis in pure water under 300 W UV radiation. The experiments have been carried out at pH 7 at which the neutral form of SMN dominates (>80%).39 Previous studies found that photo-excited pharmaceutical compounds, formed by the photosensitization process, interacted with molecular oxygen to form ˙OH, 1O2, and O2˙−.40 During the photolytic experiment, we selected 2-propanol, sodium azide (NaN3), and p-quinone as scavengers to quench ˙OH, 1O2, and O2˙− respectively.41 SMN degradation and the logarithm of relative SMN concentration versus irradiation time are displayed in Fig. 2. SMN photolysis followed pseudo-first-order kinetics in pure water. The degradation ratio of SMN achieved a value of 78% after 60 min irradiation, and the degradation rate constant (k) of SMN was 2.58 × 10−2 min−1 in pure water. The values of k decreased to 2.19 × 10−2 min−1, 2.33 × 10−2 min−1, and 2.37 × 10−2 min−1 and the degradation ratios were 72%, 74%, and 75% in the presence of 2-propanol, NaN3, and p-quinone, respectively. The results indicated that ˙OH, 1O2, and O2˙− were formed during SMN photolysis in pure water via the photosensitization process of SMN, and the impact of ˙OH was more noticeable than that of 1O2 and O2˙− on the photolysis of SMN, which were consistent with the early research conclusions that hydroxyl radicals played a critical role in the photochemical transformation of organic pollutants.42–44 However, the ratio and rate constants (k) of SMN molecules had no evident decline after adding scavengers for ˙OH, 1O2, and O2˙−; this implied that direct photolysis was the main path for photochemical behavior of SMN in pure water.
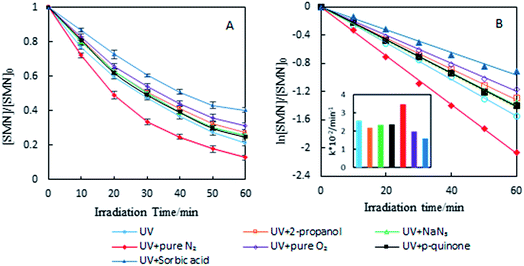 |
| Fig. 2 Degradation ratio (A) and kinetics (B) of SMN photolysis under different conditions. | |
To further understand the effect of self-sensitized behavior on SMN photolytic reactions, comparative trials under deoxygenation by N2-sparging, oxygen filling, and adding sorbic acid as a scavenger to quench 3SMN* were carried out in SMN photolysis. The results are also shown in Fig. 2. The value of k increased to 3.31 × 10−2 min−1 from 2.75 × 10−2 min−1 after deoxygenation, but decreased to 1.99 × 10−2 min−1 and 1.60 × 10−2 min−1 after oxygen filling and addition of sorbic acid, respectively (Fig. 2). Removal of 3O2 from solution by N2-sparging could lead to a decreased formation rate of 1O2 as well as lower quenching rate of 3SMN*, and oxygen filling was an opposite process to strengthen the self-sensitization. Based on these results, we could state that the self-sensitized behavior had an inhibiting effect on SMN photolysis. The reduction of k after adding sorbic acid further demonstrated that the steady state concentration of 3SMN* was very important during SMN photolysis, and the direct decomposition of 3SMN* was the main pathway of SMN photolysis.
To investigate the hydrolysis of SMN, non-irradiated experiments were conducted simultaneously. No obvious loss of SMN was observed in pure water solution of SMN; this implied that hydrolysis of SMN was negligible during the SMN aquatic environmental behavior.
3.3 Pathways of SMN photolysis in pure water
In our experiment, we found that the main path of formation of SMN photoproducts in water was 3SMN* decomposition by direct photolysis. Moreover, species of ROS formed by self-sensitized SMN could degrade SMN, which could not be ignored during SMN photochemical behaviors. To determine the mineralization degree of SMN and identify the products of SMN photolysis, TOC was monitored along with SMN photolysis under UV irradiation. The solutions obtained after 60 min of SMN photodecomposition were analyzed using LC-MS/MS. As shown in Fig. 3, no significant removal of TOC was observed even after 60 min; this meant that most of the organic carbon was still retained although the SMN photolysis under UV was effective.
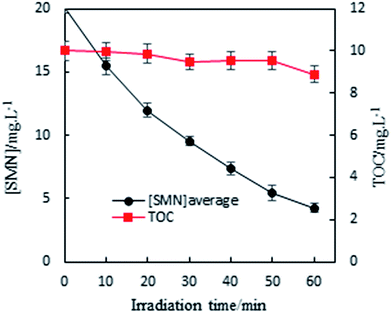 |
| Fig. 3 TOC with the SMN photolysis under UV irradiation. | |
The specific ion mass spectra of major intermediates are depicted in Fig. 4. There were three main photoproducts that could be observed: SMN-1, SMN-2, and SMN-3 with m/z of 124, 215, and 295, respectively. ˙OH radicals formed during the self-sensitization process of SMN attacked the benzene ring or the dimethyl pyrimidine group of SMN; this resulted in the formation of SMN-3. SMN-2 was derived from SO2 removal from SMN. SMN-1 was produced from the broken carbon–nitrogen bond via intermediate photolysis. These results were consistent with the previous research on photolytic mechanism of sulfonamide antibiotics.2,38 The proposed pathways of SMN photolysis in pure water are displayed in Scheme 1.
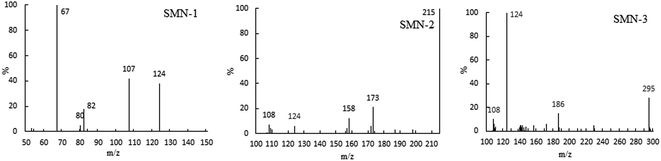 |
| Fig. 4 Major photolytic intermediate ion mass spectra of SMN. | |
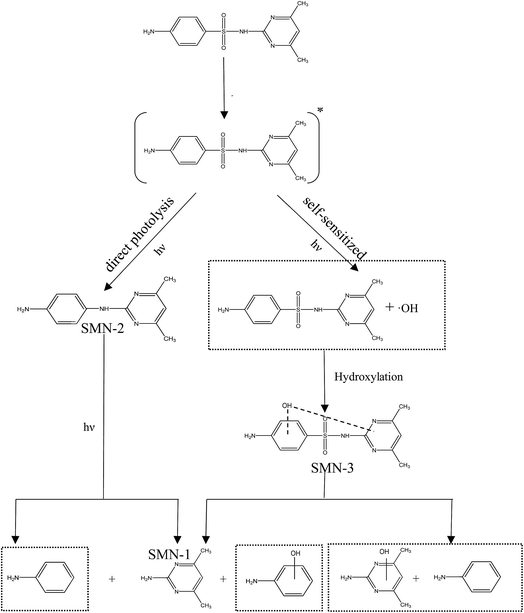 |
| Scheme 1 Proposed degradation pathways of SMN in pure water. | |
3.4 Effect of soluble substances on SMN photolysis
Halide ions, nitrate, and dissolved organic matter (DOM) are ordinary soluble substances in natural waters, which can be converted into free radicals under sunlight.6,45 Indirect photolytic behaviors of SMN caused by soluble substances are common photodecomposition behavior of SMN. We selected Cl−, NO3−, and FA as probes to explore the effect of photoactive substances on SMN photolysis in water. In view of SMN having been frequently detected in both freshwater and seawater, the concentrations of Cl−, NO3−, and FA were set according to their content in natural waters (Table 1).6
Table 1 Concentrations of Cl−, NO3−, and TOC for freshwater and seawater
Parameters |
Freshwater |
Seawater |
Cl− (mM) |
3.4 |
469 |
NO3− (μM) |
93.6 |
29.0 |
TOC (mg C L−1) |
4.0 |
6.1 |
As the content of Cl− is very low in freshwater and exhibits gradient variation in estuarine waters, we set different Cl− concentrations (100, 300, and 500 mM) relevant to estuarine and sea conditions6 to explore the effects on the photolysis of SMN. Experiments showed that the k values of SMN photolysis increased with an increase in Cl− concentration (Fig. 5). In pure water, the main photolysis pathway is 3SMN* disintegration to photoproducts by direct photolysis. Hence, the steady state concentration of triplet-excited state (Tss) is very important for degradation. Previous studies indicated the Tss of 3SAs* was higher at a higher ionic strength.46,47 ET was employed to evaluate the electron accepting ability of the 3SAs*.48,49 SMN exists in natural water (pH = 6.5–8.5) as two different species: neutral state (SMN0) and anionic state (SMN−).39 The ET values are 2.45 V and 2.25 V, which let 3SMN* oxidize Cl− [E(Cl2˙−/Cl−) = 2.0 V].6 Thus, the deactivation of 3SMN* induced by Cl− may lead to the formation of Cl2˙− and halogenated intermediates via the pathway demonstrated in Scheme 2. Triplet-induced halogenation of SMN will increase the ionic strength effect, and at the same time reduce the ground state SMN; this can explain why the rate of SMN photolysis is faster in the presence of Cl− as compared to that in the presence of pure water.
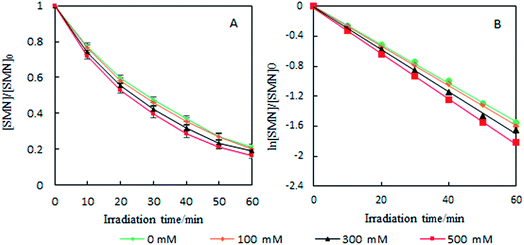 |
| Fig. 5 Effects of Cl− on degradation ratio (A) and kinetics (B) of SMN photolysis. | |
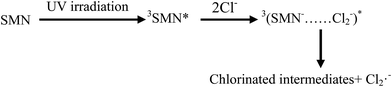 |
| Scheme 2 Reaction pathway of SMN induced by chloride ion. | |
The role of NO3− was paradoxical: either its absorption (200–400 nm) covering the SMN absorption range (200–330 nm) resulted in a decrease of photolytic rate of SMN or produced reactive species (e.g., ˙OH, NO2˙) via sensitization effects (eqn (1)–(3)); this could increase the photolytic rate of SMN.
|
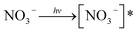 | (1) |
|
 | (2) |
|
[NO3−]* → NO2− + O(3P)
| (3) |
Our experimental results are shown in Fig. 6. In the concentration range of NO3− in natural water, the existence of NO3− led to the increase of SMN photolytic rate. To know whether ˙OH formed by NO3− sensitization played an important role during SMN photolysis, we added 2-propanol to the reaction system; the experimental findings are exhibited in Fig. 7. The k value of SMN photolysis decreased than that in pure water. The experiment confirmed that more production of ˙OH in the presence of NO3− as compared to that in pure water was the main cause of NO3− promoting the SMN photolysis.
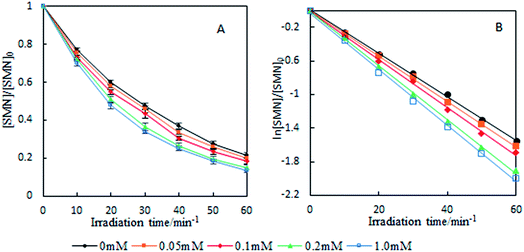 |
| Fig. 6 Effects of NO3− on degradation ratio (A) and kinetics (B) of SMN photolysis. | |
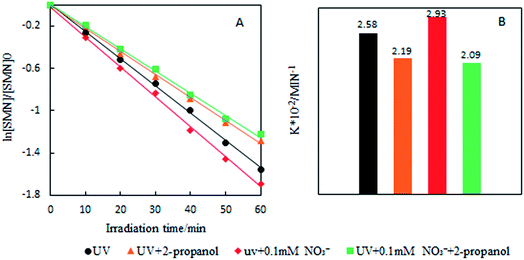 |
| Fig. 7 The k values of SMN photolysis after adding NO3− and 2-propanol. | |
FA is a ubiquitous DOM in natural waters that has strong photochemical activity. In this study, following the concentration of DOM in natural waters,50 different concentrations of FA (3.0, 6.0, and 9.0 mg C L−1) were added to pure water to investigate the effect of DOM on the photolysis of SMN. As shown in Fig. 8, photolysis rate constants of SMN decreased with an increase in FA concentration.
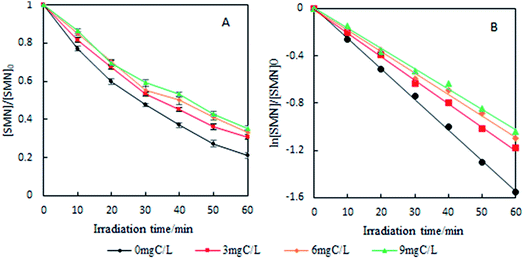 |
| Fig. 8 Effects of FA on degradation ratio (A) and kinetics (B) of SMN photolysis. | |
These conclusions were not consistent with previous studies. Others found that FA formed the excited state 3FA* and ROS under irradiation, which further induced the degradation of organic compounds and was a significant promoter of organic compound photolysis.51 The photolysis pathway of FA in SMN solution is displayed in eqn (4)–(6).
|
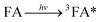 | (4) |
|
 | (5) |
|
FA + ROS → photolytic products
| (6) |
In our case, the inhibiting effect of FA on SMN photolysis might have two factors: one, the steady state concentration of 3SMN* was very important for SMN photolysis; the direct decomposition of 3SMN* has been proven to be the main pathway of SMN photolysis. Therefore, the competitive absorption for photons (200–400 nm) by FA could form 3FA*, which inhibited the production of 3SMN*. Another, the 3FA* was quenched by dissolved oxygen in the water. This process takes 3FA* back to the ground state (FA). FA not only acts as a ROS scavenger,52 but also inhibits SMN from absorbing the photons.
4. Conclusion
In this study, we investigated the photolytic behaviors of SMN via adding different scavengers to quench active species in pure water. The research indicated that 3SMN* played an important role in SMN photolysis: the main photolytic path of SMN in water was 3SMN* direct photolysis. Moreover, ˙OH formed by self-sensitized SMN could degrade SMN, which could not be ignored during study of SMN photochemical behaviors. The main photoproducts of SMN were identified by LC-MS/MS, which showed that SMN could not be mineralized although photolysis under UV was effective. Via studying the effects of ordinary soluble substances in natural water on SMN photolysis, we also found that the rate of SMN photolysis was faster in the presence of Cl− and NO3−. The triplet-induced halogenation of SMN increased the ionic strength and reduced the ground state SMN; these were the primary causes of promotion of SMN photolysis by Cl−. More ˙OH produced in the presence of NO3− could be of advantage to SMN photolysis. A low concentration of FA in water inhibited SMN photolysis. Competitive absorption of photons by FA with SMN and ROS scavenged by FA were considered to be the main reasons. The environmental risks of intermediates during SMN photolysis in waters must cause concern.
Conflicts of interest
There are no conflicts of interest to declare.
Acknowledgements
The authors are grateful for the financial support provided by the Sci-Tech Support Plan Foundation of Sichuan Province, China (Grant No. 2017RZ0035), Scientific Research Foundation of the Education Department of Sichuan Province, China (Grant No. 15ZB0261), Key Project of Technological Innovation of Leshan Normal University, Sichuan Province, China (Grant No. Z1413), Project of Introduction of teachers of Leshan Normal University, Sichuan Province, China (Grant No. Z1517), and Scientific Research Foundation of Leshan Science & Technology Bereau, Sichuan Province, China (Grant No. 15ZDYJ0144).
References
- W. Baran, E. Adamek, J. Ziemiánska and A. Sobczak, Effects of the presence of sulfonamides in the environment and their influence on human health, J. Hazard. Mater., 2011, 196, 1–15 CrossRef CAS PubMed.
- A. P. S. Batista, F. C. C. Pires and A. C. S. C. Teixeira, The role of reactive oxygen species in sulfamethazine degradation using UV-based technologies and products identification Ana, J. Photochem. Photobiol., A, 2014, 290, 77–85 CrossRef CAS.
- Q. Q. Zhang, et al., Comprehensive evaluation of antibiotics emission and fate in the river basins of China: source analysis, multimedia modeling, and linkage to bacterial resistance, Environ. Sci. Technol., 2015, 49, 6772–6782 CrossRef CAS PubMed.
- A. K. Sarmah, M. T. Meyer and A. B. Boxall, A global perspective on the use, sales, exposure pathways, occurrence, fate and effects of veterinary antibiotics (VAs) in the environment, Chemosphere, 2006, 65, 725–759 CrossRef CAS PubMed.
- K. Chen and J. L. Zhou, Occurrence and behavior of antibiotics in water and sediments from the Huangpu River, Shanghai, China, Chemosphere, 2014, 95, 604–612 CrossRef CAS PubMed.
- Y. J. Li, et al., Effects of halide ions on photodegradation of sulfonamide antibiotics: formation of halogenated intermediates, Water Res., 2016, 102, 405–412 CrossRef CAS PubMed.
- A. Shimizu, et al., Ubiquitous occurrence of sulfonamides in tropical Asian waters, Sci. Total Environ., 2013, 452–453 Search PubMed.
- R. Zhang, et al., Occurrence and risk of antibiotics in the coastal aquatic environment of the Yellow Sea. North China, Sci. Total Environ., 2013, 450–451, 197–204 CrossRef CAS PubMed.
- L. J. Zhou, et al., Excretion masses and environmental occurrence of antibiotics in typical swine and dairy cattle farms in China, Sci. Total Environ., 2013, 444, 183–195 CrossRef CAS PubMed.
- A. L. Batt, D. D. Snow and D. S. Aga, Occurrence of sulfonamide antimicrobials in private water wells in Washington County, Idaho, USA, Chemosphere, 2006, 64, 1963–1971 CrossRef CAS PubMed.
- M. J. García-Galán, et al., Application of fully automated online solid phase extraction-liquid chromatography electrospray-tandem mass spectrometry for the determination of sulfonamides and their acetylated metabolites in groundwater, Anal. Bioanal. Chem., 2011, 399, 795–806 CrossRef PubMed.
- I. Arslan-Alaton, S. Dogruel, E. Baykal and G. Gerone, Combined chemical and biological oxidation of penicillin formulation effluent, J. Environ. Manage., 2004, 73, 155–163 CrossRef PubMed.
- K. Kümmerer, Antibiotics in the aquatic environment a review part I, Chemosphere, 2009, 75, 417–434 CrossRef PubMed.
- A. G. Trovó, et al., Degradation of sulfamethoxazole in water by solar photo-Fenton. Chemical and toxicological evaluation, Water Res., 2009, 43, 3922–3931 CrossRef PubMed.
- M. J. García-Galán, M. Silvia Díaz-Cruz and D. Barceló, Identification and determination of metabolites and degradation products of sulfonamide antibiotics, Trends Anal. Chem., 2008, 27, 1008–1022 CrossRef.
- M. J. García-Galán, M. S. Díaz-Cruz and D. Barceló, Combining chemical analysis and ecotoxicity to determine environmental exposure and to assess risk from sulfonamides, Trends Anal. Chem., 2009, 28, 804–819 CrossRef.
- A. Białk-Bielinska, et al., Ecotoxicity evaluation of selected sulfonamides, Chemosphere, 2011, 85, 928–933 CrossRef PubMed.
- L. H. M. L. M. Santos, et al., Ecotoxicological aspect As related to the presence of pharmaceuticals in the aquatic environment, J. Hazard. Mater., 2010, 175, 45–95 CrossRef CAS PubMed.
- T. W. Tzeng, et al., Photolysis and photocatalytic decomposition of sulfamethazine antibiotics in an aqueous solution with TiO2, RSC Adv., 2016, 6, 69301–69310 RSC.
- U. Riaz, S. M. Ashraf and J. Kashyap, Enhancement of photocatalytic properties of transitional metal oxides using conducting polymers: A mini review, Mater. Res. Bull., 2015, 71, 75–90 CrossRef CAS.
- U. Riaz, S. M. Ashraf and A. Ruhela, Catalytic degradation of orange G under microwave irradiation with a novel nanohybrid catalyst, J. Environ. Chem. Eng., 2015, 3, 20–29 CrossRef CAS.
- U. Riaz, et al., Sonochemical Facile Synthesis of Self-Assembled Poly(o-phenylenediamine)/Cobalt Ferrite Nanohybrid with Enhanced Photocatalytic Activity, Ind. Eng. Chem. Res., 2016, 55, 6300–6309 CrossRef CAS.
- U. Riaz and S. M. Ashraf, Synergistic effect of microwave irradiation and conjugated
polymeric catalyst in the facile degradation of dyes, RSC Adv., 2014, 4, 47153 RSC.
- X. H. Wang and A. Y. C. Lin, Photo transformation of cephalosporin antibiotics in an aqueous environment results in higher toxicity, Environ. Sci. Technol., 2012, 46, 12417–12426 CrossRef CAS PubMed.
- S. Yan and W. Song, Photo-transformation of pharmaceutically active compounds in the aqueous environment: a review, Environ. Sci.: Processes Impacts, 2014, 16, 697–720 CAS.
- L. E. Jacobs, et al., Fulvic acid mediated photolysis of ibuprofen in water, Water Res., 2011, 45, 4449–4458 CrossRef CAS PubMed.
- M. M. Kelly and W. A. Arnold, Direct and indirect photolysis of the phytoestrogens genistein and daidzein, Environ. Sci. Technol., 2012, 46, 5396–5403 CrossRef CAS PubMed.
- J. Li, et al., Effects of nitrate and humic acid enrofloxacin photolysis in an aqueous system under three light condition: kinetics and mechanism, Environ. Chem., 2014, 11, 333–340 CrossRef.
- R. R. Chowdhury, P. A. Charpentier and M. B. Ray, Photodegradation of 17-estradiol in aquatic solution under solar irradiation: kinetics and influencing water parameters, J. Photochem. Photobiol., A, 2011, 219, 67–75 CrossRef CAS.
- T. Zeng and W. A. Arnold, Pesticide photolysis in prairie potholes: probing photo-sensitized processes, Environ. Sci. Technol., 2013, 47, 6735–6745 CrossRef CAS PubMed.
- A. G. Trovó, et al., Photodegradation of sulfamethoxazole in various aqueous media: Persistence, toxicity and photoproducts assessment, Chemosphere, 2009, 77, 1292–1298 CrossRef PubMed.
- L. K. Ge, et al., New insights into the aquatic photochemistry of fluoroquinolone antibiotics: Direct photodegradation, hydroxyl-radical oxidation, and antibacterial activity changes, Sci. Total Environ., 2015, 527–528, 12–17 CrossRef CAS PubMed.
- M. Sturini, et al., Sunlight-induced degradation of fluoroquinolones in wastewater effluent: Photoproducts identification and toxicity, Chemosphere, 2015, 134, 313–318 CrossRef CAS PubMed.
- S. J. Jiao, et al., Aqueous photolysis of tetracycline and toxicity of photolytic products to luminescent bacteria, Chemosphere, 2008, 73, 377–382 CrossRef CAS PubMed.
- Y. Chen, et al., Photolysis of chlortetracycline in aqueous solution: Kinetics, toxicity and products, J. Environ. Sci., 2012, 24, 254–260 CrossRef CAS.
- V. K. Balakrishnan, K. A. Terry and J. Toito, Determination of sulfonamide antibiotics in wastewater: a comparison of solid phase micro extraction and solid phase extraction methods, J. Chromatogr. A, 2006, 1131, 1–10 CrossRef CAS PubMed.
- L. Sun, et al., Analysis of sulfonamides in environmental water samples based on magneticmixed hemimicelles solid-phase extraction coupled with HPLC-UV detection, Chemosphere, 2009, 77, 1306–1312 CrossRef CAS PubMed.
- R. Nassar, et al., Photodegradation of sulfamethazine, sulfamethoxypiridazine, amitriptyline, and clomipramine drugs in aqueous media, J. Photochem. Photobiol., A, 2017, 336, 176–182 CrossRef CAS.
- M. K. Li, et al., Sulfamethazine degradation in water by the VUV/UV process: Kinetics, mechanism and antibacterial activity determination based on a mini fluidic VUV/UV photoreaction system, Water Res., 2017, 108, 348–355 CrossRef CAS PubMed.
- D. E. Moore, Mechanisms of photosensitization by phototoxic drugs, Mutat. Res., 1998, 422, 165–173 CrossRef CAS PubMed.
- S. Canonica, U. Jans, K. Stemmler and J. Hoigne, Transformation kinetics of phenols in water: Photosensitization by dissolved natural organic material and aromatic ketones, Environ. Sci. Technol., 1995, 29, 1822–1831 CrossRef CAS PubMed.
- J. Wenk, U. Gunten and S. Canonica, Effect of dissolved organic matter on the transformation of contaminants induced by excited triplet states and the hydroxyl radical, Environ. Sci. Technol., 2011, 45, 1334–1340 CrossRef CAS PubMed.
- M. M. Dong and F. L. Rosario-Ortiz, Photochemical formation of hydroxyl radical from effluent organic matter, Environ. Sci. Technol., 2012, 46, 3788–3794 CrossRef CAS PubMed.
- Y. J. Li, et al., Photodegradation mechanism of sulfonamides with excited triplet state dissolved organic matter: A case of sulfadiazine with 4-carboxybenzophenone as a proxy, J. Hazard. Mater., 2015, 290, 9–15 CrossRef CAS PubMed.
- S. Bahnmüller, U. Gunten and S. Canonica, Sunlight-induced transformation of sulfadiazine and sulfamethoxazole in surface waters and wastewater effuents, Water Res., 2014, 57, 183–192 CrossRef PubMed.
- G. J. Janz, B. G. Oliver, G. R. Lakshminarayanan and G. E. Mayer, Electrical conductance, diffusion, viscosity, and density
of sodium nitrate, sodium perchlorate, and sodium thiocyanate in concentrated aqueous solutions, J. Phys. Chem., 1970, 74, 1285–1289 CrossRef CAS.
- E. R. Nightingale Jr, Viscosity of aqueous sodium perchlorate solutions, J. Phys. Chem., 1959, 63, 742–743 CrossRef.
- S. Canonica, B. Hellrung and J. Wirz, Oxidation of phenols by triplet aromatic ketones in aqueous solution, J. Phys. Chem. A, 2000, 104, 1226–1232 CrossRef CAS.
- D. Jornet, et al., Experimental and theoretical studies on the mechanism of photochemical hydrogen transfer from 2-aminobenzimidazole to np and pp aromatic ketones, J. Phys. Chem. B, 2010, 114, 11920–11926 CrossRef CAS PubMed.
- H. S. Yang, X. Yang, L. Xu and A. Q. Zhang, The progress of humic substances photochemistry in natural waters, Photogr. Sci. Photochem., 2004, 2, 22 Search PubMed.
- J. Werner, et al., Environmental photochemistry of tylosin: efficient, reversible photoisomerization to a less active isomer, followed by photolysis, J. Agric. Food Chem., 2007, 55, 7062–7068 CrossRef CAS PubMed.
- Y. Li, J. F. Niu and W. L. Wang, Photolysis of Enrofloxacin in aqueous systems under simulated sunlight irradiation: Kinetics, mechanism and toxicity of photolysis products, Chemosphere, 2011, 85, 892–897 CrossRef CAS PubMed.
|
This journal is © The Royal Society of Chemistry 2018 |