DOI:
10.1039/C8QM00320C
(Research Article)
Mater. Chem. Front., 2018,
2, 1853-1858
Efficient room-temperature phosphorescence based on a pure organic sulfur-containing heterocycle: folding-induced spin–orbit coupling enhancement†
Received
2nd July 2018
, Accepted 7th August 2018
First published on 8th August 2018
Abstract
The development of metal-free room-temperature phosphorescence (RTP) emitters is a very challenging task, due to one of the most critical issues in pure organic systems: very weak spin–orbit coupling (SOC). Herein, we report a novel mechanism of folding-induced SOC enhancement, which is mainly responsible for an efficient RTP of thianthrene (TA), a pure organic sulfur-containing heterocycle. In a rigid environment, SOC is significantly triggered by the folding along the S⋯S axis, arising from the orthogonality between the non-bonding pz-orbitals of the S atoms and the π-orbitals of the phenyl rings that results in a 1(n,σ*) transition configuration at the bend in essence. A single-molecule doped poly(methyl methacrylate) (PMMA) film of TA exhibits strong RTP emission once deoxygenated, which enables highly-sensitive oxygen-sensing. This work provides a novel strategy to design high-efficiency pure organic RTP materials using a folding-induced SOC enhancement mechanism.
Introduction
Metal-free pure organic room-temperature phosphorescence (RTP) materials are of great interest, owing to their numerous attractive applications (e.g., organic light-emitting diodes, sensors and bio-imaging),1 as well as their advantages of low cost and structural diversity compared to organometallic complexes.2 However, metal-free pure organic RTP is extremely inefficient under ambient conditions, which is ascribed to two main negative factors: one is the weak spin–orbit coupling (SOC), which disables the generation of the lowest triplet excitons (T1) via intersystem crossing (ISC) from the singlet excited states (Sm, m ≥ 1) to the triplet manifold (Tn, n ≥ 1); the other is the rapid non-radiative deactivation, which outmatches the radiative transition from the T1 exciton to the ground state (S0).3 To achieve efficient pure organic RTP, two effective strategies have been widely adopted: (i) the non-metallic heavy-atom effect (e.g., Br, I and deuterium) is used to promote SOC for an efficient ISC process,4 as well as the introduction of heteroatoms (e.g., B, O, N, S) to form an (n,π*) transition configuration for the enhancement of SOC5 according to the El-Sayed rule;6 and (ii) rigid environments are usually provided to suppress the non-radiative deactivations as far as possible, such as crystallization,7 H-aggregation,8 halogen bonding,9 host matrixes,10 metal–organic frameworks (MOF),11etc. Here, we focus on other new mechanisms and new strategies to effectively enhance SOC for efficient pure organic RTP materials, since SOC is one of the most fundamental and crucial issues in pure organic systems.
Thianthrene (TA), a sulfur-containing heterocycle, was primarily investigated in terms of its electrochemical and chemical properties in previous studies,12 but the understanding of its photophysics was obviously inadequate, especially for RTP.13 Therefore, we systematically characterized the photophysical properties of TA, and it was surprisingly found that TA not only exhibited strong RTP in both the crystalline state and a single-molecule doped film, but the RTP is also very sensitive to the oxygen in the doped film, which may enable a promising application in the field of oxygen-sensing. Experimental and theoretical investigations reveal that the bright RTP can be mainly ascribed to a novel mechanism of folding-induced SOC enhancement, as a result of the restricted folding geometry of TA in a rigid environment. Compared with the planar conformation, the SOC of folded TA between S1 and T1 is significantly enhanced by about two orders of magnitude, resulting from the extraction of the lone pair electrons of the pz-orbitals of the S atoms from the p–π conjugation, as well as the formation of a 1(n,σ*) transition configuration in the S1 state relative to 3(π,π*) of the T1 state. Different from the usual RTP, TA features a new mechanism of folding-induced SOC enhancement to facilitate RTP, which suggests a novel strategy to realize efficient RTP materials using TA as a prototype.
Results and discussion
Photophysical properties
Photoluminescence (PL) spectra of TA under different conditions were recorded. In Fig. 1a, TA in tetrahydrofuran (THF) solution shows blue emission with a PL peak at λmax = 436 nm, and it has identical PL spectra in different polar solvents, indicating a non-charge-transfer excited state. A time-resolved experiment of TA in THF solution (10 μM) reveals a mono-exponential decay with only one lifetime of 8.06 ns, demonstrating the fluorescence characteristic of the TA monomer. At room temperature, TA in dilute THF solution exhibits only fluorescence emission without RTP even after deoxygenation, and its PL efficiency (ηPL) is measured to be only 2%. However, strong phosphorescence emission was observed at 77 K with a peak wavelength λmax of 490 nm and a long lifetime (Fig. S1–S4, ESI†).
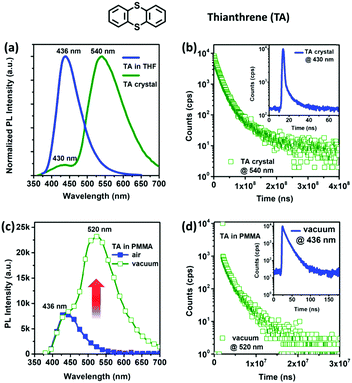 |
| Fig. 1 Photoluminescence spectra (a) and time-resolved spectra (b) of TA in dilute THF solution and as a crystal. Photoluminescence spectra (c) and time-resolved spectra (d) of TA in a PMMA film before and after degassing. | |
As shown in Fig. 1a and b, the TA crystal shows bright green emission under UV excitation (λ = 365 nm), whose PL spectrum is peaked at λmax = 540 nm together with a weak shoulder at λmax = 430 nm. Time-resolved PL reveals a long-lived species of 23.6 ms at λmax = 540 nm and a prompt one of 1.75 ns at λmax = 430 nm, corresponding to phosphorescence and fluorescence emissions, respectively. Compared with phosphorescence (λmax = 490 nm) in frozen THF solution, a redshift of 50 nm can be attributed to the aggregation effect with strong intermolecular π–π and S⋯S interactions in the crystal (Fig. S5, ESI†).14 Interestingly, the ηPL of the TA crystal is significantly increased up to 25% relative to that in the THF solution under ambient conditions, indicating more efficient phosphorescence than fluorescence (Fig. S6 and S7, ESI†).7 For the crystal, the RTP emission of TA is further confirmed by oxygen-quenching experiments, in which the phosphorescence band is markedly enhanced after deoxygenation (Fig. S8 and S9, ESI†).5c
For promising and practical applications, the amorphous film state of pure organic RTP systems is more highly desirable than the crystalline state, but it is usually more difficult to harvest efficient RTP due to the aggravated vibration dissipation of triplet exciton energy in the less rigid amorphous state.15 A TA-doped poly(methyl methacrylate) (PMMA) film (5 wt% TA) was prepared from its chloroform solution by drop-casting on quartz. The doped film demonstrates only fluorescence emission under ambient conditions (Fig. S10, ESI†). Once deoxygenated (shown in Fig. 1c and d), the doped film in a vacuum exhibits a strong RTP emission at λmax = 520 nm with a long lifetime of 2.41 ms (0.96 ms@50.69% and 3.09 ms@49.31%), along with the still existing fluorescence at λmax = 436 nm with a short lifetime of 10.02 ns (4.71 ns@33.12% and 12.65 ns@66.88%). It is noteworthy that the RTP of the doped film red-shifted to some extent relative to that in the frozen THF solution due to the reduced environmental rigidity in the doped film at room temperature, whereas it is obviously blue-shifted from that of the TA crystal owing to the absence of intermolecular π–π and S⋯S interactions in the doped film.
Interestingly, the RTP in the doped film is more sensitive to oxygen quenching than that in the crystal, as a result of the larger contact surface of oxygen in the loosely packed amorphous film than in the TA crystal. Moreover, obvious phosphorescence-to-fluorescence switching in the doped film was observed to enable a potential application in the field of oxygen-sensing, and after all, pure organic metal-free RTP doped films were rarely studied for optical oxygen sensors.16 As shown in Fig. 2, when the PMMA film was purged with nitrogen (N2) flow, bright green RTP (under UV excitation λ = 254 nm) was observed in sharp contrast to blue fluorescence in the unaffected areas of the doped film. We also placed the doped film in a quartz cell which was filled with nitrogen, and PL spectra were recorded upon equilibrium of adsorbed air and nitrogen. The totally reversible change in RTP intensity gives rise to a great change in color coordinates, which facilitates realization of high-sensitivity oxygen sensors.
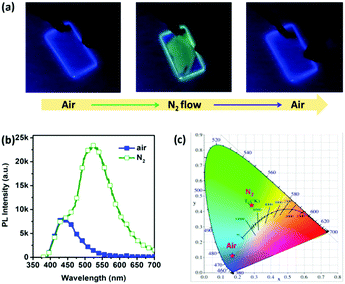 |
| Fig. 2 (a) Emission change and recovery of a PMMA film under N2 flow and in air, respectively. (b) PL spectra and (c) CIE coordinates of the PMMA film in air and a N2 atmosphere, respectively. | |
Theoretical analysis
In order to clarify the factor responsible for the efficient RTP in TA, both the excited state properties (energy and transition character) and SOC effect were calculated at the TA geometry directly taken from the crystal to first understand the generation of triplet excitons via an ISC process using the Beijing Density Function (BDF) program.17 The triplet states (Tn) that are close to or lower than the S1 state in energy are mainly focused on for SOC estimation, because the ISC (S1 → Tn) process is more likely to occur along these channels from S1 to the triplet manifold (Tn, n = 1–6) due to the favorable energy gap and sizeable SOC (Fig. S11 and Table S2, ESI†). In the meantime, the sizeable SOC is also beneficial to the electric transition dipole moment of μT1→S0, and the square of SOC is proportional to RTP radiation.18 To further understand the sizeable SOC, natural transition orbitals (NTOs) for S1 and Tn (n = 1–6) were used to describe their transition characters (Fig. S12, ESI†). Because of the folding conformation, the lone pair electron of the pz-orbital of the S atom is extracted from the p–π conjugation. As a result, the S1 state is mainly assigned to the transition character of 1(n,σ*), which consists of a single-center pz → px transition at the S atom and a pz → σC–C* transition at the bend.19 Instead, T1 and T2 primarily show the transition character of 3(π,π*), while the high-lying Tn (6 ≥ n ≥ 3) displays a mixture of 3(π,π*) and 3(n,π*).20 According to the El-Sayed rule, these SOCs can be affordable for efficient ISC in TA, because effective orbital overlapping occurs under the operation of the orbital angular momentum operator between different transition configurations, such as 1(n,σ*) and 3(π,π*).21
More intrinsically, due to the optimized geometry, TA is bent along the S⋯S axis with a folding dihedral angle (θ) of 136.15° in the ground state (S0), in good agreement with 129.44° from the crystal structure. Differently, both the excited state geometries of S1 and T1 are optimized into stable and approximately planar conformations, and their NTOs mainly show a typical transition character of (π,π*) due to the entire p–π conjugation between the S atoms and phenyl rings (Fig. S13–S15, ESI†). In order to elucidate the role of folding evolution, the SOC effect is further estimated as a function of θ at 10° intervals between 100° and 180°. Upon decreasing θ from 180° to 100°, the SOC (S1–T1) shows a fluctuating trend that is first constant, then increasing and finally decreasing, and a maximum is obtained at 110°–120°, as shown in Fig. 3. From 180° to 150°, the SOCs are too small to afford an efficient ISC process from S1 to T1, due to almost the same (π,π*) transition characters between S1 and T1 induced by the effective p–π conjugation in approximately planar conformations. When θ < 140°, the lone pair electrons of the pz-orbitals of the S atoms are extracted from p–π conjugation due to the orthogonality with the π-orbitals of the phenyl rings, and the pz → px transition starts to gradually join into the S1 state, together with the incremental formation of σC–C*. Thus, the increasing difference between 1(n,σ*) of the S1 state and 3(π,π*) of the T1 state leads to a rapid increase in SOC (S1–T1) as θ decreases. Further folding results in the maximum SOC coefficient between 110° and 120°, indicating the greatest difference of the transition character between S1 and T1. Once θ < 110°, the SOC is decreased dramatically due to the reoccupation of both S1 and T1 by the (π,π*) transition character. As a whole, the transition character of S1 (hole and particle) is very sensitive to the θ, whereas T1 basically stays in the same 3(π,π*) from 180° to 100°. As a result, the distinct transition characters between S1 and T1 are definitely responsible for the enhancement of SOC according to the El-Sayed rule (Fig. S16–S24, ESI†). As for the reason, the formation of 1(n,σ*) can be ascribed to the gradual orthogonal orientation between the lone pair electron pz-orbital of the S atoms and π-orbital of the phenyl rings with decreasing θ, leading to the extraction of the pz-orbital from p–π conjugation, the formation of σC–C* and the participation of the px-orbital at the bend.22 In a sharp contrast, the SOC between S1 and T1 (10.5653 cm−1) of TA at θ = 120° is about two orders of magnitude larger than that of the planar one (0.1261 cm−1), revealing a novel mechanism of folding-induced SOC enhancement. It is noteworthy that the square of SOC contributes to about four orders of magnitude promotion of the ISC rate in the folding conformation relative to the planar one according to the golden-rule approximation.21 As a matter of fact, TA exhibits very efficient RTP in both the doped film and crystalline state, which can be better understood in terms of the folding-induced SOC enhancement as a result of the restricted relaxation of the folding conformation in rigid environments.
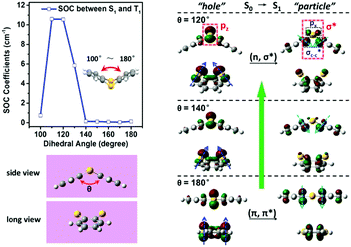 |
| Fig. 3 SOC between S1 and T1 as a function of the folding dihedral angle (θ). Some transition characters of S1 (120°, 140° and 180°) from different views are put together. | |
Experimental analysis
What is more, the low-temperature (LT, 78 K) experiments provide solid evidence for the novel mechanism of folding-induced SOC enhancement. Compared with those at room temperature (RT), both the TA doped film and crystal at LT exhibit blue-shifted and greatly enhanced phosphorescence emission (Fig. S26, ESI†), indicating restricted geometrical relaxation from the folded conformation to a planar one. In Fig. 4, the TA crystal displays a slightly larger blue shift than its doped film from RT to LT, due to the effect of temperature-affected intermolecular interactions in the crystal. Importantly, in the normalized PL spectra, the PL intensity ratio between RTP and fluorescence remains almost unchanged from LT to RT in the crystal, implying an acceptable fact that both S1 and T1 excitons have almost the same temperature-dependent non-radiative decay. Simultaneously, in a more rigid medium, the almost unchanged PL intensity ratio between RTP (Icry-P) and fluorescence (Icry-F) can also be ascribed to the nearly constant SOC, as a result of the very limited change of θ of TA from LT to RT in the crystal. As a comparison, the TA doped film exhibits an obvious decrease of the RTP/fluorescence (Ifilm-P/Ifilm-F) PL intensity ratio from LT to RT in the normalized PL spectra, which can be assigned to a significant decrease in SOC due to the increased θ of single molecule TA in the excited state in the PMMA matrix. As for the reason, the PMMA matrix acts as a less rigid medium than the crystal. Upon increasing the temperature, the different rigidity of the surrounding medium will restrict the geometrical relaxation of the TA excited state to a different extent, leading to a big change of θ in the PMMA matrix but a small change in the crystal. Thus, the enlarged θ of TA will give rise to an obvious decrease of SOC and even hinder ISC generation and radiation of T1 excitons in the doped film, corresponding to a remarkable decrease in the RTP/fluorescence PL intensity ratio from LT to RT, as shown in Fig. 4. Therefore, the RTP/fluorescence intensity ratio from LT to RT confirms the novel mechanism of folding-induced SOC enhancement.23
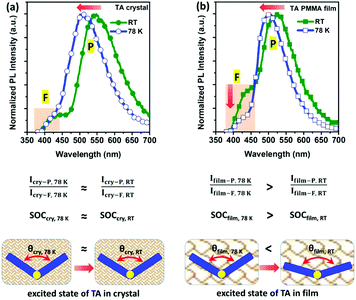 |
| Fig. 4 Normalized PL spectra of the (a) TA crystal and (b) TA-PMMA doped film at RT and 78 K. Diagrams of the change in the folding dihedral angle (θ) are shown, and I represents the spectral intensity. | |
In order to verify the grafting ability of the folding-induced SOC enhancement mechanism, two other TA derivatives were synthesized for the examination of their RTP properties (Fig. S27–S30, ESI†). Both 1,2′-bithianthrene (1TA2TA) and 1,1′-bithianthrene (1TA1TA) are chemically bonded TA dimers with different bonding sites. In crystal structures, a gradual increase in the θ of the TA unit is found from TA and 1TA2TA to 1TA1TA (Fig. 5a). Although only an ∼10° difference exists between the maximum (139.88° for 1TA1TA) and the minimum (129.44° for TA) folding dihedral angle, a significant change of SOC will be introduced, as shown in Fig. 3. As a result, a gradual decrease in the RTP/fluorescence PL intensity ratio is observed (Fig. 5b), together with a gradual decline in ηPL in crystals from TA (25%) and 1TA2TA (19%) to 1TA1TA (11%), which is consistent with the folding-induced SOC enhancement very well.
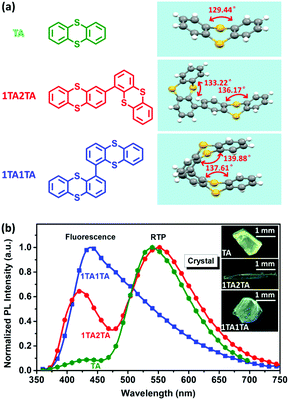 |
| Fig. 5 (a) Molecular structures and folding conformations in crystals of TA, 1TA2TA and 1TA1TA compounds. (b) PL spectra and crystal photographs of TA, 1TA2TA and 1TA1TA crystals. | |
Conclusions
In conclusion, we systematically investigated the photophysical properties of TA in solution, as a crystal and in a single-molecule doped film. Efficient RTP emission was observed in the TA crystal and the degassed TA-doped PMMA film. As an efficient RTP emitter, the TA-doped PMMA film is found to be highly sensitive to oxygen, which may enable its featured applications in the field of oxygen-sensing and facilitate the high-resolution measurement of the trace oxygen partial pressure. Both experimental and theoretical investigations reveal that a new mechanism of folding-induced SOC enhancement, which is responsible for the efficient RTP of TA in rigid media. In essence, with decreasing θ, the gradual orthogonal orientation between the lone pair electron of pz-orbital of the S atom and the π-orbital of the phenyl ring leads to the extraction of the pz-orbital from p–π conjugation and the formation of 1(n,σ*) at the bend, which contributes to the enhancement of SOC. Furthermore, both the temperature-dependent experiment and TA derivatives with different θ values consistently confirm the folding-induced SOC enhancement mechanism through the increased RTP/fluorescence PL intensity ratio with decreasing θ. As a simple and efficient RTP model, TA can act as the indicator to guide the extensive exploration of highly efficient RTP emitters in sulfur-containing heterocycles using a folding-induced SOC enhancement mechanism.
Conflicts of interest
There are no conflicts to declare.
Acknowledgements
This work was supported by the National Basic Research Program of China (2015CB655003 and 2016YFB0401001), the National Natural Science Foundation of China (51673083, 51473063 and 51873077), the Postdoctoral Innovation Talent Support Project (BX201700097 and BX20180121), the China Postdoctoral Science Foundation (2017M620108) and the Open Project Foundation of the State Key Laboratory of Luminescence and Applications (Changchun Institute of Optics, Fine Mechanics and Physics of the Chinese Academy of Sciences, SKLA-2016-04).
Notes and references
-
(a) R. Kabe, N. Notsuka, K. Yoshida and C. Adachi, Adv. Mater., 2016, 28, 655 CrossRef PubMed;
(b) D. Chaudhuri, E. Sigmund, A. Meyer, L. Roeck, P. Klemm, S. Lautenschlager, A. Schmid, S. R. Yost, T. Van Voorhis, S. Bange, S. Hoeger and J. M. Lupton, Angew. Chem., Int. Ed., 2013, 52, 13449 CrossRef PubMed;
(c) G. Zhang, J. Chen, S. J. Payne, S. E. Kooi, J. N. Demas and C. L. Fraser, J. Am. Chem. Soc., 2007, 129, 8942 CrossRef PubMed;
(d) P. Xue, J. Sun, P. Chen, P. Wang, B. Yao, P. Gong, Z. Zhang and R. Lu, Chem. Commun., 2015, 51, 10381 RSC;
(e) Z. Mao, Z. Yang, Y. Mu, Y. Zhang, Y.-F. Wang, Z. Chi, C.-C. Lo, S. Liu, A. Lien and J. Xu, Angew. Chem., Int. Ed., 2015, 54, 6270 CrossRef PubMed;
(f) B. Xu, H. Wu, J. Chen, Z. Yang, Z. Yang, Y.-C. Wu, Y. Zhang, C. Jin, P.-Y. Lu, Z. Chi, S. Liu, J. Xu and M. Aldred, Chem. Sci., 2017, 8, 1909 RSC;
(g) H. Wu, C. Hang, X. Li, L. Yin, M. Zhu, J. Zhang, Y. Zhou, H. Agren, Q. Zhang and L. Zhu, Chem. Commun., 2017, 53, 2661 RSC;
(h) S. Hirata, K. Totani, H. Kaji, M. Vacha, T. Watanabe and C. Adachi, Adv. Opt. Mater., 2013, 1, 438 CrossRef;
(i) J. Wei, B. Liang, R. Duan, Z. Cheng, C. Li, T. Zhou, Y. Yi and Y. Wang, Angew. Chem., Int. Ed., 2016, 55, 15589 CrossRef PubMed.
-
(a) S. Mukherjee and P. Thilagar, Chem. Commun., 2015, 51, 10988 RSC;
(b) R. Kabe and C. Adachi, Nature, 2017, 550, 384 CrossRef PubMed.
-
(a) Y. Xie, Y. Ge, Q. Peng, C. Li, Q. Li and Z. Li, Adv. Mater., 2017, 29, 1606829 CrossRef PubMed;
(b) S. Hirata, Adv. Opt. Mater., 2017, 5, 1700116 CrossRef;
(c) J. Zhao, W. Wu, J. Sun and S. Guo, Chem. Soc. Rev., 2013, 42, 5323 RSC;
(d) M. Baroncini, G. Bergamini and P. Ceroni, Chem. Commun., 2017, 53, 2081 RSC.
-
(a) G. D. Gutierrez, G. T. Sazama, T. Wu, M. A. Baldo and T. M. Swager, J. Org. Chem., 2016, 81, 4789 CrossRef PubMed;
(b) S. d'Agostino, F. Grepioni, D. Braga and B. Ventura, Cryst. Growth Des., 2015, 15, 2039 CrossRef;
(c) S. Hirata, K. Totani, T. Watanabe, H. Kaji and M. Vacha, Chem. Phys. Lett., 2014, 591, 119 CrossRef;
(d) S. Hirata, K. Totani, J. Zhang, T. Yamashita, H. Kaji, S. R. Marder, T. Watanabe and C. Adachi, Adv. Funct. Mater., 2013, 23, 3386 CrossRef.
-
(a) Z. Chai, C. Wang, J. Wang, F. Liu, Y. Xie, Y.-Z. Zhang, J.-R. Li, Q. Li and Z. Li, Chem. Sci., 2017, 8, 8336 RSC;
(b) J. Yang, Z. Ren, Z. Xie, Y. Liu, C. Wang, Y. Xie, Q. Peng, B. Xu, W. Tian, F. Zhang, Z. Chi, Q. Li and Z. Li, Angew. Chem., Int. Ed., 2017, 56, 880 CrossRef PubMed;
(c) Z. Yang, Z. Mao, X. Zhang, D. Ou, Y. Mu, Y. Zhang, C. Zhao, S. Liu, Z. Chi, J. Xu, Y.-C. Wu, P.-Y. Lu, A. Lien and M. R. Bryce, Angew. Chem., Int. Ed., 2016, 55, 2181 CrossRef PubMed;
(d) J. Chen, Y. Chen, Y. Wu, X. Wang, Z. Yu, L. Xiao, Y. Liu, H. Tian, J. Yao and H. Fu, New J. Chem., 2017, 41, 1864 RSC;
(e) C. Zhou, S. Zhang, Y. Gao, H. Liu, T. Shan, X. Liang, B. Yang and Y. Ma, Adv. Funct. Mater., 2018, 28, 1802407 CrossRef;
(f) Z. Yu, Y. Wu, L. Xiao, J. Chen, Q. Liao, J. Yao and H. Fu, J. Am. Chem. Soc., 2017, 139, 6376 CrossRef PubMed;
(g) G. Bergamini, A. Fermi, C. Botta, U. Giovanella, S. Di Motta, F. Negri, R. Peresutti, M. Gingras and P. Ceroni, J. Mater. Chem. C, 2013, 1, 2717 RSC;
(h) A. Fermi, G. Bergamini, R. Peresutti, E. Marchi, M. Roy, P. Ceroni and M. Gingras, Dyes Pigm., 2014, 110, 113 CrossRef.
-
(a) M. El-Sayed, J. Chem. Phys., 1963, 38, 2834 CrossRef;
(b) M. El-Sayed, J. Chem. Phys., 1963, 38, 3032 CrossRef.
-
(a) W. Z. Yuan, X. Y. Shen, H. Zhao, J. W. Y. Lam, L. Tang, P. Lu, C. Wang, Y. Liu, Z. Wang, Q. Zheng, J. Z. Sun, Y. Ma and B. Z. Tang, J. Phys. Chem. C, 2010, 114, 6090 CrossRef;
(b) C. Li, X. Tang, L. Zhang, C. Li, Z. Liu, Z. Bo, Y. Q. Dong, Y.-H. Tian, Y. Dong and B. Z. Tang, Adv. Opt. Mater., 2015, 3, 1184 CrossRef;
(c) Y. Gong, G. Chen, Q. Peng, W. Z. Yuan, Y. Xie, S. Li, Y. Zhang and B. Z. Tang, Adv. Mater., 2015, 27, 6195 CrossRef PubMed;
(d) Z. He, W. Zhao, J. W. Y. Lam, Q. Peng, H. Ma, G. Liang, Z. Shuai and B. Z. Tang, Nat. Commun., 2017, 8, 416 CrossRef PubMed.
-
(a) Z. An, C. Zheng, Y. Tao, R. Chen, H. Shi, T. Chen, Z. Wang, H. Li, R. Deng, X. Liu and W. Huang, Nat. Mater., 2015, 14, 685 CrossRef PubMed;
(b) X. Zhen, Y. Tao, Z. An, P. Chen, C. Xu, R. Chen, W. Huang and K. Pu, Adv. Mater., 2017, 29, 1606665 CrossRef PubMed;
(c) S. Cai, H. Shi, Z. Zhang, X. Wang, H. Ma, N. Gan, Q. Wu, Z. Cheng, K. Ling, M. Gu, C. Ma, L. Gu, Z. An and W. Huang, Angew. Chem., Int. Ed., 2018, 57, 4005 CrossRef PubMed;
(d) S. Cai, H. Shi, D. Tian, H. Ma, Z. Cheng, Q. Wu, M. Gu, L. Huang, Z. An, Q. Peng and W. Huang, Adv. Funct. Mater., 2018, 28, 1705045 CrossRef.
-
(a) O. Bolton, K. Lee, H.-J. Kim, K. Y. Lin and J. Kim, Nat. Chem., 2011, 3, 205 CrossRef PubMed;
(b) D. Lee, O. Bolton, B. C. Kim, J. H. Youk, S. Takayama and J. Kim, J. Am. Chem. Soc., 2013, 135, 6325 CrossRef PubMed;
(c) O. Bolton, D. Lee, J. Jung and J. Kim, Chem. Mater., 2014, 26, 6644 CrossRef;
(d) M. S. Kwon, D. Lee, S. Seo, J. Jung and J. Kim, Angew. Chem., Int. Ed., 2014, 53, 11177 CrossRef PubMed.
-
(a) H. Chen, X. Ma, S. Wu and H. Tian, Angew. Chem., Int. Ed., 2014, 53, 14149 CrossRef PubMed;
(b) D. Li, F. Lu, J. Wang, W. Hu, X.-M. Cao, X. Ma and H. Tian, J. Am. Chem. Soc., 2018, 140, 1916 CrossRef PubMed.
- H. Mieno, R. Kabe, N. Notsuka, M. D. Allendorf and C. Adachi, Adv. Opt. Mater., 2016, 4, 1015 CrossRef.
-
(a) M. S. Park, K. Ha Park, K. Jun, S. R. Shin and S. W. Oh, J. Heterocycl. Chem., 2002, 39, 1279 CrossRef;
(b) J. B. Edson and D. M. Knauss, J. Polym. Sci., Part A: Polym. Chem., 2004, 42, 6353 CrossRef;
(c) K. Cho and W. K. Lee, Bull. Korean Chem. Soc., 2007, 28, 911 CrossRef;
(d) H. Morita, Y. Oida, T. Ariga, S. Fukumoto, M. C. Sheikh, T. Fujii and T. Yoshimura, Tetrahedron, 2011, 67, 4672 CrossRef;
(e) M. C. Sheikh, T. Iwasawa, A. Nakajima, A. Kitao, N. Tsubaki, R. Miyatake, T. Yoshimura and H. Morita, Synthesis, 2014, 42 Search PubMed;
(f) J. M. Lovell and J. A. Joule, J. Chem. Soc., Perkin Trans. 1, 1996, 2391 RSC;
(g) T. Fujita, H. Kamiyama, Y. Osawa, H. Kawaguchi, B. J. Kim, A. Tatami, W. Kawashima, T. Maeda, A. Nakanishi and H. Morita, Tetrahedron, 2007, 63, 7708 CrossRef;
(h) W. Adam, C. M. Mitchell, C. R. Saha-Möller, T. Selvam and O. Weichold, J. Mol. Catal. A: Chem., 2000, 154, 251 CrossRef;
(i) G. Cosa, M. S. Galletero, L. Fernández, F. Márquez, H. García and J. Scaiano, New J. Chem., 2002, 26, 1448 RSC;
(j) R. T. Tjahjanto, M. F. Peintinger, T. Bredow and J. Beck, Eur. J. Inorg. Chem., 2012, 3625 CrossRef.
-
(a) A. Arena, S. Campagna, A. Mezzasalma, R. Saija and G. Saitta, II Nuovo Cimento D, 1993, 15, 1521 CrossRef;
(b) W. Holzer, A. Penzkofer, A. Lux, H.-H. Hörhold and E. Kley, Synth. Met., 2004, 145, 119 CrossRef;
(c) H. Okamura, R. Matsumori and M. Shirai, J. Photopolym. Sci. Technol., 2004, 17, 131 CrossRef;
(d) H. Okuyama, H. Naito and M. Shirai, J. Photopolym. Sci. Technol., 2008, 21, 285 CrossRef;
(e) P. Pander, A. Swist, J. Soloducho and F. B. Dias, Dyes Pigm., 2017, 142, 315 CrossRef.
-
(a) J. Yang, Z. Ren, B. Chen, M. Fang, Z. Zhao, B. Z. Tang, Q. Peng and Z. Li, J. Mater. Chem. C, 2017, 5, 9242 RSC;
(b) J. Yang, X. Zhen, B. Wang, X. Gao, Z. Ren, J. Wang, Y. Xie, J. Li, Q. Peng, K. Pu and Z. Li, Nat. Commun., 2018, 9, 840 CrossRef PubMed.
-
(a) H. Chen, X. Yao, X. Ma and H. Tian, Adv. Opt. Mater., 2016, 4, 1397 CrossRef;
(b) X. Ma, C. Xu, J. Wang and H. Tian, Angew. Chem., Int. Ed., 2018, 57, 10854–10858 CrossRef PubMed.
-
(a) G. Zhang, G. M. Palmer, M. Dewhirst and C. L. Fraser, Nat. Mater., 2009, 8, 747 CrossRef PubMed;
(b) E. G. Ermolina, R. T. Kuznetsova, Y. V. Aksenova, R. M. Gadirov, T. N. Kopylova, E. V. Antina, M. B. Berezin and A. S. Semeikin, Sens. Actuators, B, 2014, 197, 206 CrossRef;
(c) Y. Feng, J. Cheng, L. Zhou, X. Zhou and H. Xiang, Analyst, 2012, 137, 4885 RSC;
(d) Y. Yu, M. S. Kwon, J. Jung, Y. Zeng, M. Kim, K. Chung, J. Gierschner, J. H. Youk, S. M. Borisov and J. Kim, Angew. Chem., Int. Ed., 2017, 56, 16207 CrossRef PubMed;
(e) P. Lehner, C. Staudinger, S. M. Borisov and I. Klimant, Nat. Commun., 2014, 5, 4460 CrossRef PubMed.
-
(a) W. Liu, F. Wang and L. Li, J. Theor. Comput. Chem., 2003, 2, 257 CrossRef;
(b) Z. Li, B. Suo, Y. Zhang, Y. Xiao and W. Liu, Mol. Phys., 2013, 111, 3741 CrossRef;
(c) Z. Li, Y. Xiao and W. Liu, J. Chem. Phys., 2012, 137, 154114 CrossRef PubMed;
(d) Z. Li, Y. Xiao and W. Liu, J. Chem. Phys., 2014, 141, 054111 CrossRef PubMed.
-
(a) H. Ma, W. Shi, J. Ren, W. Li, Q. Peng and Z. Shuai, J. Phys. Chem. Lett., 2016, 7, 2893 CrossRef PubMed;
(b) R. Duan, Q. Peng, X. Shen, G. Han, Y. Guo, Y. Zeng and Y. Yi, J. Phys. Chem. C, 2018, 122, 3748 CrossRef.
- S. Webster, D. Peceli, H. Hu, L. A. Padilha, O. V. Przhonska, A. E. Masunov, A. O. Gerasov, A. D. Kachkovski, Y. L. Slominsky, A. I. Tolmachev, V. V. Kurdyukov, O. O. Viniychuk, E. Barrasso, R. Lepkowicz, D. J. Hagan and E. W. Van Stryland, J. Phys. Chem. Lett., 2010, 1, 2354 CrossRef.
- W. Zhao, Z. He, J. W. Y. Lam, Q. Peng, H. Ma, Z. Shuai, G. Bai, J. Hao and B. Z. Tang, Chem, 2016, 1, 592 Search PubMed.
-
(a) D. Beljonne, Z. Shuai, G. Pourtois and J. L. Bredas, J. Phys. Chem. A, 2001, 105, 3899 CrossRef;
(b) C. M. Marian, WIREs Comput. Mol. Sci., 2012, 2, 187 CrossRef.
- S. Kim, Y. Kwon, J.-P. Lee, S.-Y. Choi and J. Choo, J. Mol. Struct., 2003, 655, 451 CrossRef.
-
(a) T. G. Pavlopoulos, J. Chem. Phys., 1969, 51, 2936 CrossRef;
(b) A. C. Albrecht, J. Chem. Phys., 1963, 38, 354 CrossRef;
(c) T. J. Penfold and G. A. Worth, Chem. Phys., 2010, 375, 58 CrossRef;
(d) J. Tatchen, N. Gilka and C. M. Marian, Phys. Chem. Chem. Phys., 2007, 9, 5209 RSC;
(e) A. Rodriguez-Serrano, V. Rai-Constapel, M. C. Daza, M. Doerr and C. M. Marian, Photochem. Photobiol. Sci., 2012, 11, 1860 RSC;
(f) D. Danovich, C. M. Marian, T. Neuheuser, S. D. Peyerimhoff and S. Shaik, J. Phys. Chem. A, 1998, 102, 5923 CrossRef.
Footnotes |
† Electronic supplementary information (ESI) available: Measurement details, photophysical data, theoretical data, crystal structures and other information. CCDC 1836801, 1838721 and 1838722. For ESI and crystallographic data in CIF or other electronic format see DOI: 10.1039/c8qm00320c |
‡ Haichao Liu and Yu Gao contributed equally to this work. |
|
This journal is © the Partner Organisations 2018 |