DOI:
10.1039/C7QM00615B
(Research Article)
Mater. Chem. Front., 2018,
2, 1004-1008
Paramagnetism enhancement by in situ electrochemical hole doping into a Prussian Blue thin film†
Received
27th December 2017
, Accepted 4th March 2018
First published on 5th March 2018
Abstract
A Prussian Blue (PB) film formed on an indium tin oxide substrate was incorporated in an electrolysis cell with an ionic-liquid midlayer (N,N-diethyl-N-methyl-N-(2-methoxyethyl)ammonium bis(trifluoromethanesulfonyl)imide) as an electrolyte. The ionic-liquid electrolysis cell mounted on a SQUID magnetometer was examined under DC bias voltage up to 4.0 V by injecting the charge carriers electrochemically into the PB film. Electrochemical hole doping increased the FeIII/FeII ratio in the PB film, resulting in a remarkable enhancement of magnetization accompanied by quasi-reversible electrochromism.
Introduction
Recently, electrical manipulation of magnetization and magnetic properties of the materials has been attempted by many researchers aiming the application to practical devices.1–4 An outcome of the spintronics is known utilizing the control of magnetization by injecting spin-polarized current to the magnetic tunnel junction structures, enabling high-density memory devices.5,6 Another demonstration is the thin-film magnetic pickup in an information storage device, in which a giant magnetoresistive effect has been studied since 1990.7
In the present study, the hole doping into the Prussian Blue (PB) thin films was attempted in the ionic-liquid electrolysis cells, enabling the electrochemical control of an iron-valence state; the electric-field-induced magnetization was demonstrated in situ.
PB and its analogues,8–12 the oldest 3-dimensional coordination polymers,13,14 have drawn much attention over 300 years15 as pigments16,17 and also as functional materials, showing electrochemical polychromatism characteristics in the mixed-valence compounds18–22 and various magnetic properties, including the switching of magnetization by external stimuli.8–10,23–25 Also noted are the applications to rechargeable batteries,22,26 sensors, and biosensors.11,27 The cyanide-bridged coordination polymers show valence-dependent magnetism (e.g., FeII-LS–C
N–FeII-HS, Prussian White (TC < 1.7 K); FeII-LS–C
N–FeIII-HS, PB (TC = 4.2 K); FeIII-LS-C
N–FeIII-HS, Berliner Brown (TN = 12 K)).23 Sato et al. have reported the first example of the electrolysis-induced magnetization using the PB films.23 However, in situ switching of the magnetization by wet electrolysis in a SQUID magnetometer was not achieved simultaneously.
To achieve the in situ electrolysis-induced magnetization of the PB film, we adopted ionic liquids as the electrolytes due to their non-volatility.28N,N-Diethyl-N-methyl-N-(2-methoxyethyl)ammonium (DEME) is an aliphatic quaternary ammonium compound, belonging to a family of ionic liquids with higher cathodic stability and wider potential windows than other ionic liquids consisting of aromatic cations.29 Among the DEME-based ionic liquids, TFSI salt (TFSI = bis(trifluoromethanesulfonyl)imide anion) was chosen because it can form a uniform vitreous state suitable for the SQUID measurements at low temperature (Tg ∼ −91 °C).29
Experimental
Materials
The ionic liquid DEME–TFSI was purchased from Kanto Kagaku and dried in vacuum at 120 °C for 2 h before purification. Iron(III) chloride hexahydrate (FeCl3·6H2O), potassium hexacyanoferrate(III) (K3FeIII(CN)6), and the solvents were purchased from Kishida Chemical, Nacalai Tesque, and Wako Pure Chemical Industries, respectively, and used without further purification. The indium tin oxide (ITO)-coated glass substrates were also commercially available (glass thickness of 0.7 mm, ITO thickness of 200 nm, 25 × 7 mm2, GEOMATEC 0006-ITO-10SQ-25×7).
Electrodeposition of the PB film on the ITO substrate
The ITO glass substrates were ultrasonicated in deionized water, acetone, and 2-propanol for 10 min, in sequence. The dried ITO substrates were cleaned beforehand by UV/ozone irradiation. Constant-current electrolysis (120 μA/120 s) was carried out using a couple of the ITO substrates as the electrodes in a mixed aqueous solution of FeCl3 (20 mM) and K3FeIII(CN)6 (20 mM) and a uniform PB film was deposited on the cathode substrate.19 The PB film on the ITO substrate was dried in vacuum at 70 °C for 3 h before assembling the film into an electrolysis cell in order to suppress the irreversible change of the cell due to the decomposition of water in the film by applying the DC bias voltages on the cell (see Fig. S6, ESI† and Results and discussion).
Characterization of the intact PB film formed on the ITO substrate
The X-ray diffraction (XRD) patterns of the intact PB film on the ITO substrate were obtained via an Intelligent XRD system (SmartLab, Rigaku) using CuKα radiation (λ = 1.5405 Å) with and without baking in vacuum at 70 °C for 3 h.
The X-ray photoelectron spectra (XPS) of the PB film were recorded on a photoelectron spectrometer (AXIS-165×, Shimadzu) using a MgKα radiation.
Inductively coupled plasma-atomic emission spectroscopy (ICP-AES) analysis was performed to measure the content of K+ and FeII,III ions in the PB films using an ICP emission spectrometer (Series ICP-7510, Shimadzu). Three intact PB thin films on the ITO substrates were heated with 69% HNO3 (2 mL) in a test tube at 120 °C for 2 h and then cooled down to room temperature. The solution was diluted to 20 mL with 0.1 M HNO3 and served to the analysis. A calibration curve was drawn using three standard samples with (K+, FeIII) = (0 ppm, 0 ppm), (0.50 ppm, 2.00 ppm), and (1.25 ppm, 5.00 ppm), which were prepared by mixing iron standard solution (Fe 1000, Wako Pure Chemical Industries) and potassium standard solution (K 1000, Wako Pure Chemical Industries).
Cyclic voltammetry (CV) measurements of the intact PB film in 0.1 M KCl aqueous solution were performed using an electrochemistry potentiostat (Series 2450-EC, Keithley) by sweeping the potential from −1.0 to 1.2 V vs. Ag/AgCl at a scan rate of 40 mV s−1. The PB film deposited on the ITO substrate was used as the working electrode with a platinum counter electrode (CPT0.5, International chemistry Co.) and an Ag/AgCl (3 M NaCl) reference electrode (RE-1B, BAS). The experiment was carried out at room temperature.
Fabrication of the PB-film electrolysis cells
The PB-film electrolysis cell was fabricated by sandwiching an ionic liquid layer between a PB-film-deposited ITO and a blank ITO substrate across a Kapton®-tape spacer of 35 μm thickness (Fig. 1 and Fig. S1, ESI†). The side slit of the device was left open to allow gas to escape.
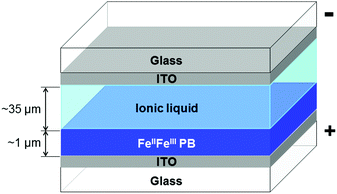 |
| Fig. 1 Schematic cross-section of the PB-film electrolysis cell. | |
Electrochromism of the PB-film electrolysis cells
Electrochromism of the electrolysis cells was observed over 300–1000 nm wavelength range on a UV/vis/NIR spectrophotometer (V-570, Jasco) in the double-beam geometry with a reference of a blank cell (ITO/air/ITO). In the measurement of DC bias dependence of the UV/vis absorption spectra, DC bias VDC from +0.0 to +4.0 V was supplied from a source measurement unit (Series 2400, Keithley) and the wavelength sweep was allowed to run 5 min after the onset of VDC on the device. Time evolution of the UV/vis absorption spectra of the cell under VDC = +3.0 V on the PB-film side was also recorded in the same way.
In situ magnetic measurements of the PB-film electrolysis cell
Magnetic measurements of the PB-film electrolysis cell were performed on a SQUID magnetometer (MPMS-1S and MPMS-5S, Quantum Design) with or without bias voltage. An electrolysis cell was fixed on one end of the brass sample rod equipped with a four-terminal feedthrough connector and a small amount of silver paste was used for wiring.
The temperature-dependence measurements were carried out under 0.1 T magnetic field from 30 to 2 K with VDC from +0.0 to +3.2 V. VDC changes were made always at 300 K, which was much higher than the glass transition. After 10 min-equilibration time, the cell was cooled down to 100 K and then, the magnetic field was applied to start the magnetization measurements.
The DC bias-dependence measurements at 300 K were performed under 1.0 T magnetic field with VDC from +0.0 to +4.0 V. Each magnetization measurement was followed by 3 min transient wait after the onset of VDC.
Results and discussion
Characterization of the intact PB film on the ITO substrate
In an attempt to elucidate the crystalline phase of the intact PB film electrodeposited on the ITO substrate, the XRD analysis was performed for the PB film after it was dried in the air at room temperature and in a vacuum at 70 °C for 3 h (see Fig. S2, ESI†). The former revealed the diffractions at 2θ = 17.5°, 24.9°, 35.5°, and 39.9°, which were indexed to the face-centered cubic structure with the lattice parameter of 10.18 Å,30 confirming the normal PB crystal phase. After dehydration of the PB film in vacuum, all peaks showed remarkable broadening, suggesting the distortion of the lattice of PB due to the loss of the coordinated water molecules at the cyanide vacancy sites,31 which is consistent with the previously reported changes in the XRD patterns of the PB analogues in bulk after dehydration.32,33
The FeIII/FeII ratio in the intact PB film was determined by XPS. The Fe 2p photoelectron spectrum is shown in Fig. S3 (ESI†). The peak areas assigned to different valence ions34 were revealed to be FeII2p3/2
:
FeIII2p3/2 ∼ 1.0
:
2.0 and FeII2p1/2
:
FeIII2p1/2 ∼ 1.0
:
1.9, suggesting the FeIII-richer composition of the PB film rather than the ratio expected for the ideal insoluble bulk PB, viz., FeII
:
FeIII ∼ 3
:
4 (see ESI†).
The absolute amounts of K and Fe atoms in the PB film were obtained by ICP-AES (Table S1, ESI†), giving the concentration ratio K+
:
FeII,III ∼ 1
:
2. Although cyclic voltammetry is another method to determine the absolute amount of Fe ions deposited on the PB film on the substrate, it revealed that the voltammetric charge involved in the total redox process of the PB film provided an underestimated value for the Fe content, which is not useful in the present discussion (Fig. S4, ESI†).
Assuming that the FeIII/FeII ratio in the intact PB film was estimated by XPS (FeII
:
FeIII ∼ 1
:
2), the FeII ions deposited on the ITO substrate was estimated to be ∼170 nmol, which was well compared to the amount of the electrolyzed charge (Q ∼ 14.5 mC (150 nmol)) based on the PB-film electrodeposited condition (I = 120 μA, t = 120 s).
Electrochromism of the PB-film electrolysis cells
To confirm whether the control of the iron-valence state in the PB film was achieved by applying the DC bias voltages on the cell, we examined the DC bias dependence of the UV/vis absorption spectra of the PB-film electrolysis cells and observed remarkable electrochromism as shown in Fig. 2a. By applying a DC bias voltage from +0.0 to +4.0 V on the PB-film side, the intensity of the intervalence charge transfer (IVCT) band at around 700 nm decreased considerably, which was attributed to the electrochemical deintercalation of the K+ ion from the PB film, resulting in the oxidation of FeII to FeIII ions.20 Up to 2.4 V bias, an isosbestic point is found at 508 nm, which is consistent with the previously reported electrochromic behavior in the PB film under electrolysis.20 When a higher bias up to 4.0 V was applied, the isosbestic behavior was lost because of the irreversible electrolytic change (vide infra).
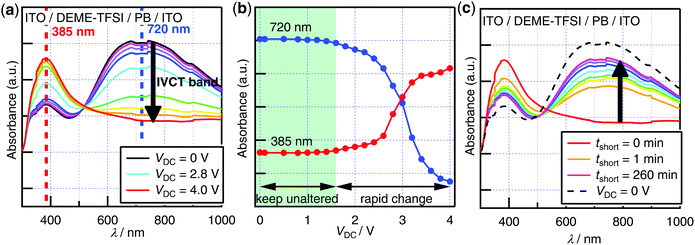 |
| Fig. 2 Electrochromism of the PB-film electrolysis cells: (a) DC bias dependence of the UV/vis absorption spectra on PB-film; (b) DC bias dependence of the representative two bands at 720 and 385 nm; (c) recovery of the UV/vis absorption spectra after short-circuiting the PB-film electrolysis cell. | |
Bias dependence of the band maxima is also plotted in Fig. 2b. Up to 1.6 V bias, the absorbance values of the maxima at 720 and 385 nm were unaltered. When a higher bias of up to 4.0 V was applied, rapid change in absorbance values was observed, suggesting the presence of the threshold bias, which was estimated as ∼1.6 V.
Fig. 2c shows the recovery of the UV/vis absorption spectra after short-circuiting the PB-film electrolysis cell held at VDC = +4.0 V. The IVCT band was restored, reaching ∼80% of the initial intensity after 260 min short-circuiting, suggesting the reintercalation of K+ ions in the ionic liquid layer into the PB film. This quasi-reversible electrochromic behavior indicated that the irreversible electrolytic change in the device occurred only partially. It was also confirmed by visual inspection of the cell, revealing the occurrence of small bubbles in the ionic liquid layer and the less recovery of the film around the sides of the cell than at the center (Fig. S5, ESI†). To reveal the cause of the irreversible change, the cell was fabricated using the air-dried PB film and DEME–TFSI without purification.
Numerous bubbles were generated on applying VDC = +3.0 V on the cell, which was attributed to the electrolysis of water in the cell (Fig. S6, ESI†). The less recovery of the film around the side-slit suggested the presence of the irreversible redox reaction of the film with oxygen or water in air (Fig. S5, ESI†).
To clarify the kinetics of the electrolysis, a time-course measurement of the UV/vis absorption spectra of the PB-film electrolysis cell under VDC = +3.0 V on the PB-film side was also conducted (Fig. S7, ESI†). On applying DC bias voltage of +3.0 V on the PB-film side, the intensity of the IVCT band suppressed drastically with time (Fig. S7a, ESI†), indicating the oxidation of FeII to FeIII ions in the PB film (Fig. S8, ESI†). Electrolysis over 200 min was required to achieve the saturation of the spectrum change, suggesting that the oxidation process from FeII to FeIII ions was dominated by the slow diffusion of the K+ ions. The isosbestic behavior was lost after 200 min from the onset of VDC = +3.0 V.
The time evolution of the band maxima was also plotted for tracking the oxidation process of the PB thin film in detail (Fig. S7b, ESI†). The absorbance at 751 nm, corresponding to the IVCT band, showed an exponential decay (τ ∼ 2000 s), while the absorbance at 394 nm synchronously increased.
Magnetic responses of the PB film against electrolysis
Ferrimagnetic magnetization as a function of electrochemical doping.
Berliner Brown has a ferrimagnetic long-range order below TN = 12 K23 and a large magnetization change is expected in this temperature range, accompanying with hole-doping in the PB film (Fig. S9, ESI†). Temperature dependence of magnetization increments ΔM(VDC) = M(VDC) − M(0 V) under DC bias (VDC) from 0 to +3.2 V on the PB-film side is shown in Fig. 3. Above the threshold DC bias of +2.2 V, the increase in spontaneous magnetization and a gradual rise of Néel temperature TN (Fig. S10, ESI†) is found, which is consistent with the electrochromism observed in the DC-bias dependence of the IVCT band. This suggested that the intact PB film (FeIIFeIII) was partially oxidized by the bias, yielding Berliner Brown (FeIIIFeIII) with ferrimagnetic long-range order.23
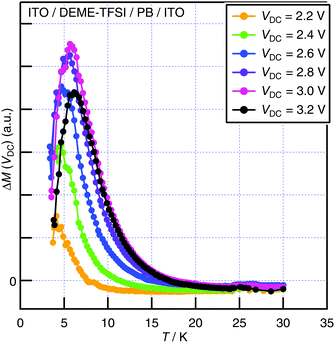 |
| Fig. 3 Low-temperature magnetization ΔM induced by VDC up to +3.2 V. Magnetic field of 0.1 T was used. | |
In situ DC bias dependence of magnetization of the PB-film electrolysis cell at T = 300 K
In order to observe in situ bias-enhanced paramagnetism, DC bias dependence of magnetization was examined at constant temperature (T = 300 K). Since the structural elements of the cell contributed to magnetic susceptibility unignorably relative to the PB film at 300 K, the distortion of the raw SQUID voltage curves was inevitable and resulted in a failure in the automatic centering of the sample. Therefore, instead of using the longitudinal magnetization calculated by the measurement software, we analyzed the raw SQUID voltage outputs in the manner described in ESI.†
Fig. S11a (ESI†) shows the raw SQUID voltage outputs V(x) with and without DC bias VDC, in which the slight difference of the curves was detected, suggesting the hole-doping in the PB film by applying VDC. The differences of the raw voltage curves ΔV(VDC) = V(VDC) − V(0 V) were calculated for VDC = +0.1 to +4.0 V (Fig. 4a). Since the peak height of the curve ΔV(VDC) was proportional to the induced magnetization of the PB film under the DC bias voltage ΔM(VDC), it was obtained by the procedure shown in Fig. S11b (see ESI†).35 ΔM(VDC) at 300 K is plotted in Fig. 4b. Bias-enhanced paramagnetism is clearly observed at 300 K, which is attributed to the increase in the paramagnetic iron(III) ions by the hole doping in the PB film.
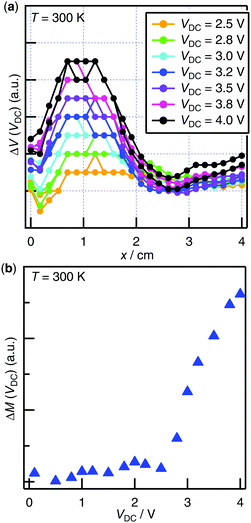 |
| Fig. 4 (a) Enhancement of the raw SQUID signal V(x) under DC bias at 300 K with 1.0 T magnetic field; (b) DC bias dependence of the induced magnetization ΔM at 300 K with 1.0 T magnetic field. | |
Conclusions
The oxidation of FeII to FeIII ions in the PB film was successfully achieved by electrochemical hole doping under DC bias VDC from +0.0 to +4.0 V on the PB-film side, which was confirmed by quasi-reversible electrochromism. The magnetic measurements of the cell were performed on a SQUID magnetometer, demonstrating the switching of the film from a paramagnet to a ferrimagnet with large magnetic response at low temperatures and paramagnetism enhancement of the film at 300 K in situ. Along with the advantage of ease of fabrication, the PB-film electrolysis cells were revealed to show significant magnetic response on application of DC bias voltage.
Conflicts of interest
There are no conflicts to declare.
Acknowledgements
We thank Dr Naoto Matsuyama for the XPS and Prof. Hiroki Akutsu and Satoshi Yamashita (Graduate School of Science, Osaka University) for the CV. We are also grateful to Prof. Akinori Saeki (Graduate School of Engineering, Osaka University) allowing us to use the UV/vis/NIR spectrometer and to Prof. Taro Uematsu (Graduate School of Engineering, Osaka University) for help in the ICP-AES. We would like to thank Ms Yuko Fujiie for helping in the fabrication of the SQUID sample rod. This study is Contribution No. 57 from the Research Center for Structural Thermodynamics.
Notes and references
- F. Matsukura, Y. Tokura and H. Ohno, Nat. Nanotechnol., 2015, 10, 209 CrossRef CAS PubMed.
- H. Ohno, D. Chiba, F. Matsukura, T. Omiya, E. Abe, T. Dietl, Y. Ohno and K. Ohtani, Nature, 2000, 408, 944 CrossRef CAS PubMed.
- D. Chiba, S. Fukami, K. Shimamura, N. Ishiwata, K. Kobayashi and T. Ono, Nat. Mater., 2011, 10, 853 CrossRef CAS PubMed.
- K. Shimamura, D. Chiba, S. Ono, S. Fukami, N. Ishiwata, M. Kawaguchi, K. Kobayashi and T. Ono, Appl. Phys. Lett., 2012, 100, 122402 CrossRef.
- J. C. Slonczewski, J. Magn. Magn. Mater., 1996, 159, L1 CrossRef CAS.
- L. Berger, Phys. Rev. B: Condens. Matter Mater. Phys., 1996, 54, 9353 CrossRef CAS.
- M. N. Baibich, J. M. Broto, A. Fert, F. Nguyen Van Dau, F. Petroff, P. Eitenne, G. Creuzet, A. Friederich and J. Chazelas, Phys. Rev. Lett., 1988, 61, 2472 CrossRef CAS PubMed.
- H. Tokoro and S. Ohkoshi, Dalton Trans., 2011, 40, 6825 RSC.
- M. Verdaguer, A. Bleuzen, V. Marvaud, J. Vaissermann, M. Seuleiman, C. Desplanches, A. Scuiller, C. Train, R. Garde, G. Gelly, C. Lomenech, I. Rosenman, P. Veillet, C. Cartier and F. Villain, Coord. Chem. Rev., 1999, 190–192, 1023 CrossRef CAS.
- D. R. Talham and M. W. Meisel, Chem. Soc. Rev., 2011, 40, 3356 RSC.
- F. Ricci and G. Palleschi, Biosens. Bioelectron., 2005, 21, 389 CrossRef CAS PubMed.
- A. A. Karyakin, Electroanalysis, 2001, 13, 813 CrossRef CAS.
- H. J. Buser, D. Schwarzenbach, W. Petter and A. Ludi, Inorg. Chem., 1977, 16, 2704 CrossRef CAS.
- T. R. Cook, Y.-R. Zheng and P. J. Stang, Chem. Rev., 2013, 113, 734 CrossRef CAS PubMed.
- A. Kraft, Bull. Hist. Chem., 2008, 33, 61 CAS.
- A. Ludi, J. Chem. Educ., 1981, 58, 1013 CrossRef CAS.
- M. Ware, J. Chem. Educ., 2008, 85, 612 CrossRef CAS.
- V. D. Neff, J. Electrochem. Soc., 1978, 125, 886 CrossRef CAS.
- K. Itaya, H. Akahoshi and S. Toshima, J. Electrochem. Soc., 1982, 129, 1498 CrossRef CAS.
- C. A. Lundgren and R. W. Murray, Inorg. Chem., 1988, 27, 933 CrossRef CAS.
- K.-C. Cheng, F.-R. Chen and J.-J. Kai, Electrochim. Acta, 2007, 52, 3330 CrossRef CAS.
- J. Wang, L. Zhang, L. Yu, Z. Jiao, H. Xie, X. W. Lou and X. W. Sun, Nat. Commun., 2014, 5, 4921 CrossRef CAS PubMed.
- O. Sato, S. Hayami, Y. Einaga and Z.-Z. Gu, Bull. Chem. Soc. Jpn., 2003, 76, 443 CrossRef CAS.
- M. Okubo, D. Asakura, Y. Mizuno, T. Kubo, H. Zhou, A. Okazawa, N. Kojima, K. Ikedo, T. Mizokawa and I. Honma, Angew. Chem., Int. Ed., 2011, 50, 6269 CrossRef CAS PubMed.
- T. Yamada, K. Morita, H. Wang, K. Kume, H. Yoshikawa and K. Awaga, Angew. Chem., Int. Ed., 2013, 52, 6238 CrossRef CAS PubMed.
- M. Okubo, D. Asakura, Y. Mizuno, J.-D. Kim, T. Mizokawa, T. Kubo and I. Honma, J. Phys. Chem. Lett., 2010, 1, 2063 CrossRef CAS.
- B. Kong, C. Selomulya, G. Zheng and D. Zhao, Chem. Soc. Rev., 2015, 44, 7997 RSC.
-
H. Ohno, in Electrochemical Aspects of Ionic Liquids, ed. H. Ohno, John Willey & Sons, Inc., Hoboken, New Jersey, 2nd edn, 2011, Importance and Possibility of Ionic Liquids, pp. 1–3 Search PubMed.
- T. Sato, G. Masuda and K. Takagi, Electrochim. Acta, 2004, 49, 3603 CrossRef CAS.
- F. Shiba, Colloids Surf., A, 2010, 366, 178 CrossRef CAS.
- F. Herren, P. Fischer, A. Ludi and W. Hälg, Inorg. Chem., 1980, 19, 956 CrossRef CAS.
- S. Ohkoshi, K. Arai, Y. Sato and K. Hashimoto, Nat. Mater., 2004, 3, 853 CrossRef PubMed.
- A. Yuan, C. Chu, H. Zhou, P. Yuan, K. Liu, L. Li, Q. Zhang, X. Chen and Y. Li, Eur. J. Inorg. Chem., 2010, 866 CrossRef CAS.
- G. K. Wertheim and A. Rosencwaig, J. Chem. Phys., 1971, 54, 3235 CrossRef CAS.
- Quantum Design, MPMS Application Note 1014-213, available online: https://www.qdusa.com/sitedocs/appNotes/mpms/1014-213.pdf (accessed September 2017).
Footnote |
† Electronic supplementary information (ESI) available: Experimental details, XRD patterns, XPS, ICP-AES data, time evolution of UV/vis absorption spectra of the cell under VDC, photographs of the cell before and after electrolysis, and additional magnetic data. See DOI: 10.1039/c7qm00615b |
|
This journal is © the Partner Organisations 2018 |