Fluorescence response from the surface states of nitrogen-doped carbon nanodots: evidence of a heterogeneous population of molecular-sized fluorophores†
Received
18th February 2018
, Accepted 29th September 2018
First published on 1st October 2018
Abstract
Fluorescent Nitrogen-doped Carbon Nanodots (NCDs) of ∼4 nm diameter were prepared by acid-driven microwave irradiation of DMF solvent. Spectroscopic studies of the NCDs demonstrated that excitation of the carbon core did not contribute any fluorescence emission. Instead, the emission originated exclusively from the surface states. The fluorescence featured a prominent red edge excitation shift (REES), while changing the excitation wavelength over ∼0.5 eV indicated the emergence of different emitter species in the temporal evolution of fluorescence. These results combined to indicate a large degree of heterogeneity in the population of these surface-localized emitters, so that working with different excitation energies produced different sets of excited surface fluorophores that evolved independently of each other. Fluorescence anisotropy dynamics in the NCDs was attributable to the reorientational motion of the surface fluorophores which was decoupled from the rotational diffusion of the carbon core of the NCD. The anisotropy decay rates revealed that the fluorophores had size comparable to typical organic fluorophores, irrespective of excitation energy.
Introduction
Fluorescent nanomaterials have drawn great attention due to their widespread applications in science and technology that have come up in recent years.1–7 Among these nanomaterials, carbon dots (CDs) are particularly promising due to their biocompatibility and low toxicity in comparison to luminescent semiconductor quantum dots.8–14 Moreover, their unique optical properties have ensured their extensive usage in biological labeling, bioimaging, drug delivery, fabrication of optoelectronic devices etc.10,14–22 Several methods have been reported to produce CDs such as dehydration of carbohydrates,23–25 the oxidation of carbon nanotubes26 or candle soot with nitric acid,27 laser ablation of graphite,11,16,17,20 chemical oxidation of activated carbon or graphite,28,29 thermal treatment of suitable molecular precursors,23,30,31 which may be tethered on silica nanospheres.18 Microwave-assisted preparation of CDs deserves special mention, as it offers the advantage of being a rapid, facile, eco-friendly synthetic route.32–36 In microwave synthesis, generally a precursor containing some polar moiety is irradiated over a period of time, giving rise to a visible change in colour of the sample solution, indicating the formation of CDs.
In terms of morphology, a CD essentially consists of a carbon core with an outlying surface region. The carbon core is a two-dimensional graphene-like matrix of sp2-hybridized C atoms, which may be interrupted by diamond-like inclusions of sp3-hybridized C atoms.36–38 The matrix is terminated at the periphery by heteroatomic functional groups, giving rise to the surface region. Fluorescence emission from the CDs may arise from several sources.
One principal source is the carbon core, where absorption in the UV induces π–π* transition producing hole–electron excitons. Radiative recombination of these charge carriers may produce fluorescence, as it happens in semiconductor quantum dots. If the recombination occurs exclusively within the carbon core, then quantum confinement effect is expected, i.e., the emission peak should undergo a red-shift as the core size increases. Moreover, the emission spectra must be largely insensitive to solvation and fluorescence polarization is unlikely, except when the CDs themselves are highly anisotropic, like quantum rods. These characteristics have indeed been reported by several workers.39–45 However, the charge carriers may also diffuse out of the core before recombination takes place, and access the surface region of the CDs, which is rich with surface defects as well as heteroatomic functional groups. These sites can promote charge-carrier decay, thereby significantly modifying the fluorescence properties.46–48
The other principal source of CD fluorescence consists of the functionalized organic moieties at the surface region, which act as localized small molecule emitters. In this case, each single CD behaves as an assembly of individual local emitters, just like a dye-doped polymer molecule.36 Moreover, the emission may be markedly affected by solvation dynamics, depending on the degree of interaction between the solvent and the surface fluorophore moieties. Also, this emission could be polarized and could show anisotropy dynamics.
The functionalized surface fluorophores emerge as peripheral residues around the carbon core during the latter's formation via the dehydration and carbonization of the precursor molecules.38,39 Following a pyrolytic preparation of CDs from citric acid and ethanolamine, Krysman et al. demonstrated that the carbon core is predominantly formed only at higher pyrolysis temperatures.38 Thus, for CDs synthesized at higher temperatures, the carbon core is mainly responsible for the emission. On the other hand, for CDs synthesized at lower temperatures, the emission originates mostly from surface organic fluorophores.
A well observed spectroscopic characteristics of CDs is the excitation wavelength dependence on the fluorescence emission spectra.25,34,35 Usually, a red edge emission shift (REES) is observed, which implies that as the excitation wavelength increases the fluorescence emission spectra exhibits a red-shift. However, there is little consensus about the origin of the REES. Hsu et al. noted that there might be two distinct causes behind such excitation wavelength dependent fluorescence (EDF) and the associated spectral relaxation.49 One is solvation dynamics, which is known to produce time-dependent emission Stokes shift. The other is the presence of a manifold of emissive states originating from CDs of different sizes, different band-edge structures, and carrying a wide range of functional groups at the surface. As part of their studies, they collected the fluorescence emission spectra of CDs at cryogenic temperature where solvation dynamics is frozen out, but observed EDF even under those conditions. Hence, they attributed the EDF due to the presence of multiple emissive states.
A related type of material is the Nitrogen-doped Carbon Nanodot (NCD), prepared from nitrogen containing precursors, following nearly the same method as CDs.32,50–56 The XPS data of NCDs clearly reveal the presence of several different N species – principally: graphitic, pyridinic and pyrrolic – inserted around the sp2-hybridized C atom matrix.57 While NCDs have properties similar to the CDs, they offer an additional advantage: their fluorescence parameters like quantum yield and lifetime correlate with their N-content.57–60 Strauss et al. performed theoretical calculations on both doped and un-doped CDs, modelled as amide group capped graphene ribbons.60 They found that increasing the CD size caused a red-shift in emission as expected from the quantum confinement effect. Heteroatom doping also heavily alters the spectral parameters. Implanting pyridinic N atoms at the edge of the CDs leads to emission blue-shift and increase in the radiative rate-constant. Most importantly, their calculations reveal that the fluorescence dynamics of the CDs ought to follow a poly-exponential decay even for mono-disperse particles. Moreover, they found no effect of trap states on the dynamics. However, the calculations stipulated that fluorescence decay should be faster at longer emission wavelengths, while experimental results revealed the exact opposite.
In a recent study, Dhenadayalan and co-workers dwelt upon the differences in the photophysics of CDs and NCDs in aqueous solution. They synthesized two sets of citric-acid derived carbon-dots, containing different surface functional groups: –COOH in one set, and –NH2 in the other. By changing the excitation wavelength, they examined the fluorescence characteristics of the carbon core and the surface fluorophores. For both sets, they found evidence of electron-transfer between the carbon core and the surface groups.37
In this work, we have synthesized NCDs according to a reported method,32 by acid-driven microwave irradiation of N,N-dimethylformamide (DMF). In contrast to the works reported earlier, here, we chose to initiate a probe into the population of surface fluorophores only. For this purpose, we selected optical excitation wavelengths where the carbon core remains unaffected, whereas the surface fluorophores contribute exclusively to fluorescence response. We then attempted to analyze this response in order to elucidate the nature and photophysics of these fluorophores.
Experimental details
Chemicals and sample preparation
DMF and H2SO4 were purchased from Spectrochem and Merck respectively. Both were used as received. In a typical synthesis, 0.5 mL H2SO4 was added into 5 mL DMF. Then the solution was kept in domestic microwave oven and irradiated at 180 W for 1 min. The resultant pale yellow solution was dispersed into water and purified in a centrifuge (5000 rpm, 20 min), followed by dialysis for three days with dialysis membrane of MWCO = 500–1000 Da (Spectrum Laboratories). This step was performed to ensure that the NCD solution is free of any low molecular weight, conventional organic molecular fluorophores. The purified NCDs exhibit green colour under UV-light (λ = 366 nm).
Spectroscopy
Absorption and fluorescence spectra were recorded using a Hitachi UV spectrophotometer (U-3501) and PerkinElmer (LS 55) spectrofluorimeter respectively. Picosecond fluorescence dynamic studies of the fluorophore solutions was performed using a time correlated single photon counting (TCSPC) system by employing a picosecond diode laser operating at λex = 375 nm and 450 nm, and a pulse width of ∼60 ps. The shape and size of the as prepared NCDs were characterized in Transmission Electron Microscopy (TEM), conducted in a 200 kV instrument (JEOL JEM 2100 HR) at the Centre for Research in Nanoscience and Nanotechnology (CRNN), University of Calcutta. FTIR spectra were recorded using a Perkin–Elmer RXI FTIR spectrophotometer (400–4000 cm−1).
Results and discussion
TEM analysis and size distribution
TEM images show that NCDs are well dispersed and spherical in shape with an average diameter of ∼4 nm (Fig. 1a and b). Moreover certain crystallinity is observed from both high-resolution TEM (HRTEM) image (Fig. 1c) and SAED pattern (inset of Fig. 1c). NCDs consists of parallel crystal planes with interplanar spacing of 0.22 nm and 0.31 nm which correspond to (100) and (002) facets of graphite carbon respectively.47,51 Size distribution histogram is given in Fig. 1d and it was fitted using Gaussian function which determines the average particle size of ∼4.05 nm.
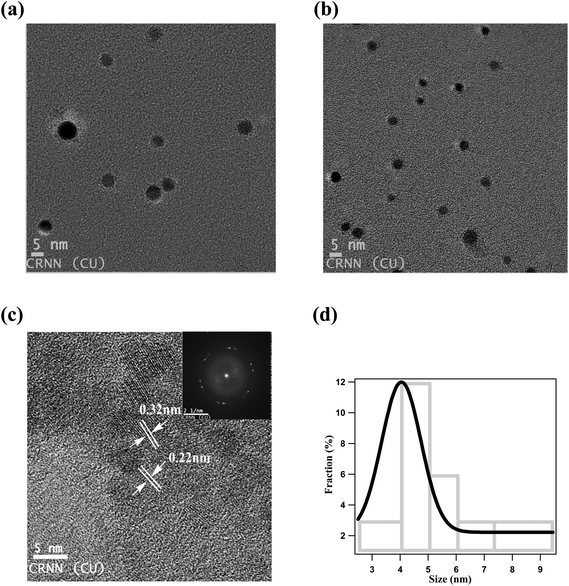 |
| Fig. 1 (a, b, c) TEM and HRTEM images and (d) size distribution of NCDs in solution. | |
FTIR study
The FTIR spectra (Fig. SF1, ESI†) reveals two bands at 1062 cm−1 and 1498 cm−1 due to presence of C–N and C
N bonds respectively. Another two bands at 2932 cm−1 and 1668 cm−1 are attributed to the stretching of N–H group. So IR study indicates the presence of functional groups on the surface of the synthesized NCDs.
Steady-state spectroscopy
Fluorescence emission and excitation spectra of the NCDs are presented in Fig. 2a and b respectively. The absorption spectrum – appended to Fig. 2b – rises around 350 nm and extends deep into the UV below 300 nm, covering a broad wavelength range. Following previous reports, the absorption at <300 nm is assigned to π–π* transition of the C
C groups in the carbon core, while the absorption band at ∼350 nm is assigned to n–π* transition involving the surface functional groups (–CN, –NH).12,37
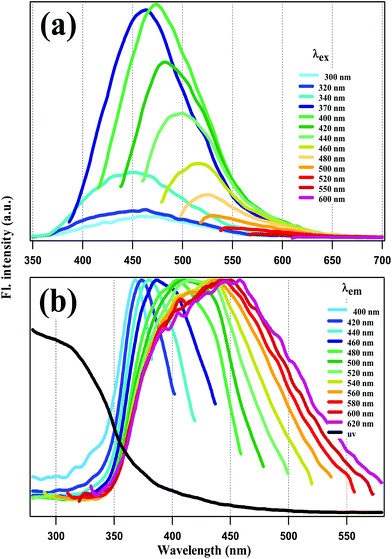 |
| Fig. 2 (a) Fluorescence emission and (b) normalized excitation spectra of NCDs at different excitation (λex) and emission wavelengths (λem) respectively. | |
Emission spectra were recorded with λex = 240 nm to 600 nm. However, emission intensity for λex < 300 nm was nil. This is highly remarkable, since it implies that core excitation did not produce any fluorescence. For λex = 300 nm to 370 nm, the emission spectra have a peak around 450 nm which does not shift much. However, for λex ≥ 370 nm, REES is observed in Fig. 2a, i.e., as λex increases the emission spectra get red-shifted and narrower as well. Excitation spectra were recorded at emission wavelengths covering the emission spectral range from 400 to 600 nm. In this range of emission wavelengths, the excitation spectra in Fig. 2b show a gradual peak shift from 370 nm to 450 nm, with no peaks at <300 nm. This again reinforces the contention made above: that the fluorescence of our NCDs does not originate from core excitation.
In the light of our results, we conclude that the surface fluorophores are predominantly responsible for the fluorescence emission of our NCDs, since carbon core excitation does not lead to any tangible emission intensity. As mentioned above, REES has often been ascribed to a variety of factors like the presence of several emissive states.45,47–49 Therefore, the REES observed in Fig. 2 indicates that the surface fluorophores are a heterogeneous lot, encompassing a broad range of absorption and corresponding emission wavelengths.
To get a more concrete picture, we decided to identify the various major and minor peak positions of the spectra by lognormal fitting. In general, simple fluorescence spectra are often fitted with the lognormal function.61 However, for the spectra in Fig. 2, which are broad and often comprise several peaks, fitting was done with a lognormal sum function L(ε) given by:
where
Li(
ε) is the lognormal component with relative amplitude
hi, peak emission energy
εi,max, asymmetry parameter
γi and bandwidth
Δi. Specifically,
but
Li(ε) = 0 for 2γi(ε − εi,max)/Δi < −1. |
The fitting results are shown in Fig. SF2 and SF3 of the ESI.† The emission and excitation peak positions (in eV) are plotted against the corresponding excitation and emission energies, respectively, in Fig. 3. The plots confirm the observations made above. Thus, for the emission spectra, the major peak (90–100% of total intensity) position is invariant for excitation between 4.13 to 3.35 eV (300 nm–370 nm), but shows REES at excitation energies lower than 3.35 eV, where the surface groups are predominantly excited. For the excitation spectra, the major peak (100–85% of total intensity) shifts towards lower energies as the emission energy is lowered, reflecting the same trend as the emission spectra. However, the excitation peaks never exceeds 3.4 eV, which implies transitions at <350 nm.
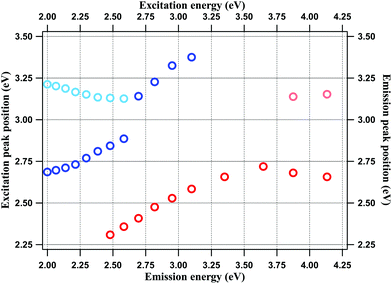 |
| Fig. 3 Emission (red circles) and excitation peak (blue circles) energies of NCDs plotted against excitation and emission energies respectively. | |
As a control experiment we checked the steady-state spectra of DMF itself and also the sample solution before microwave irradiation. Both of them were non fluorescent, confirming that the fluorescence originates from the prepared NCDs. The fluorescence quantum yield of NCDs was determined and it was ∼20%.
Time-resolved fluorescence spectroscopy
Time-resolved emission spectroscopy has been reported for CDs and NCDs in aqueous solution, using λex = 280 nm and 400 nm, respectively, with a ∼50 ps time-resolution.37 The excitation wavelengths 280 nm and 400 nm were chosen so as to predominantly excite the carbon core and the surface states, respectively. However, our steady state spectral results provide ample evidence that core excitation does not produce fluorescence in our NCDs. Thus, it is the surface fluorophores that are responsible for the fluorescence emission as well as the REES. In other words, as λex is scanned from 370 nm upward, different sets of excited states are formed among these fluorophores, which give rise to emissive states emitting at different wavelength ranges. In order to follow the dynamics of the surface fluorophores exclusively, we selected two excitation wavelengths at 375 nm and 450 nm, none of which are expected to affect the carbon core. The emission time-profiles are displayed in Fig. 4 for different emission wavelengths. All the time-profiles at both excitation wavelengths were fitted using multi-exponential decay function:
F(t) = ∑ai exp(−t/τi) |
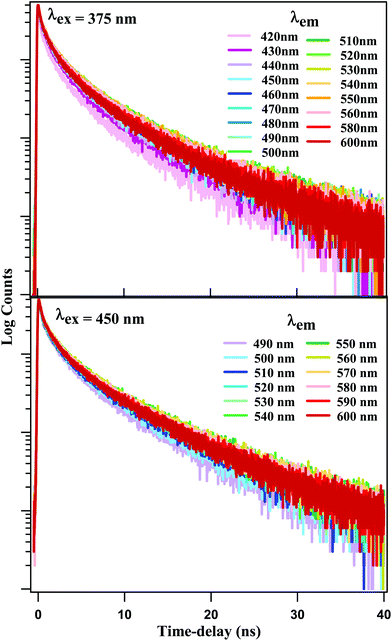 |
| Fig. 4 Fluorescence time-profiles at various emission wavelengths with λex = 375 nm and 450 nm. | |
Detailed fitting parameters are given in Tables ST1 and ST2 of the ESI.† Emission time-profiles generated with different λex = 375 nm and 450 nm, but recorded at some identical emission wavelength are shown in Fig. SF4.† We note that the time-profiles for λex = 375 nm and 450 nm exhibit a small but persistent difference. At shorter emission wavelengths, the latter exhibit faster decay, while the reverse is true at longer emission wavelengths.
The fitting data were utilized to generate the time-resolved emission spectra (TRES) and time-resolved area normalized emission spectra (TRANES) of the NCDs at λex = 375 nm and 450 nm. The TRES and TRANES are displayed in Fig. 5 and 6 respectively. The TRES in Fig. 5a and b are quite broad, and show slight time-dependent Stokes shift. From the TRANES curves in Fig. 6, the shifts are ∼800 cm−1 and ∼500 cm−1 for λex = 375 nm and 450 nm, respectively, over a time-interval of 20 ns. However, compared to the typical width of the TRANES curves (∼5000 cm−1 and ∼3000 cm−1 for λex = 375 nm and 450 nm, respectively), these shifts are not remarkable.
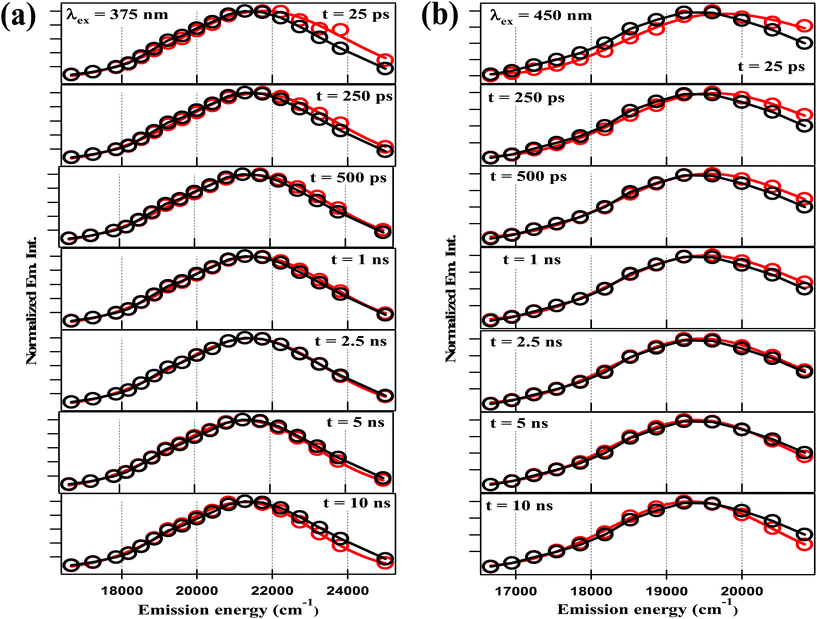 |
| Fig. 5 TRES of NCDs with (a) λex = 375 nm and (b) λex = 450 nm at different time-delays (red curve) and steady-state spectrum (black curve) are plotted together for visual comparison. | |
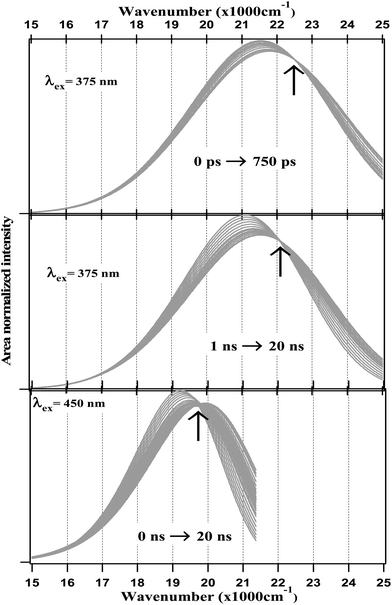 |
| Fig. 6 TRANES of NCDs at different time scales with λex = 375 nm (≈26 667 cm−1) and 450 nm (≈22 222 cm−1). | |
On the other hand, the TRANES clearly reveal the existence of isoemissive points. For λex = 375 nm, the isoemissive point appears at 22
446 cm−1 (445 nm) in a <1 ns time-scale, but subsequently shifts to 22
050 cm−1 (454 nm) in a time-scale of 1 ns to 20 ns. For λex = 450 nm, the isoemissive point appears at 19
800 cm−1 (505 nm) throughout the 0 to 20 ns time-scale. It has been demonstrated that isoemissive points may appear at different positions at different time-scales, if the system contains a mixture of more than two emitters with sharply differing fluorescence lifetimes.62 Thus, the fact that λex = 375 nm is associated with a ∼9 nm shift in the isoemissive point in contrast to λex = 450 nm, where the isoemissive point is nearly static, indicates that a more heterogeneous excited state is obtained as we use a higher excitation energy. This agrees well with the fact that the steady-state emission spectra become narrower as the excitation wavelength is gradually changed from 300 nm to 600 nm. Incidentally, using a λex = 400 nm – which is intermediate between 375 nm and 450 nm. Dhenadhayalan et al. reported two isoemissive points for NCDs at 450 nm and 507 nm,37 which are close to those we detected.
Time-resolved fluorescence anisotropy
Anisotropy time-profiles of NCDs were recorded with both λex = 375 nm and 450 nm. The time-profiles for several selected emission wavelengths are shown in Fig. 7. The curves are similar in all cases, and could be fitted with a single exponential decay function:
r(t) = r(0) × exp(−t/τrot) |
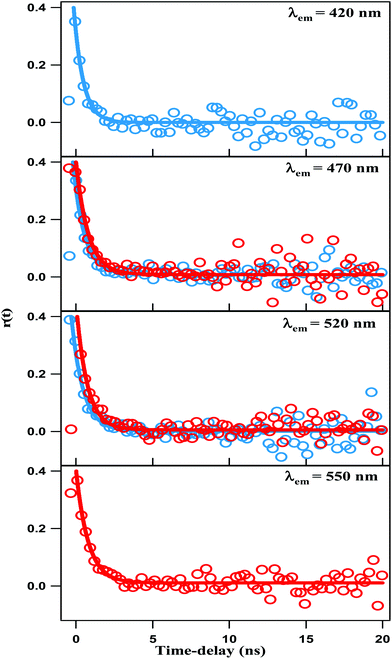 |
| Fig. 7 Time-resolved fluorescence anisotropy profile of NCDs solution at λex = 375 nm (blue line) and λex = 450 nm (red line). | |
The fitting results give r(0) ∼0.4 and τrot ∼0.7 ns, irrespective of excitation and emission wavelengths. The τrot of several 100 ps is characteristic of the rotational diffusion of common organic fluorophores in a liquid solvent like DMF. We recall that TEM images reveal that the NCDs are spherical particles with average diameter of ∼4 nm. Hence, if the emission were from the carbon core of the NCDs, the anisotropy decay would be comparable to that of a sphere of diameter ∼4 nm in the same solvent. The rotational time-constant could then be calculated from the Stokes–Einstein equation:
where
V is the hydrodynamic volume of the sphere,
i.e.,
V = (4/3)π
r3,
η is the viscosity (
ηDMF = 0.92 mPa s) and
C is the rotational coupling factor. For stick boundary conditions,
C = 1, while for slip boundary conditions, 0 <
C < 1. Since the surface groups are polar in nature, they have a favorable interaction with the polar solvent, implying that the former is appropriate in the present case. Hence, using the stick boundary condition,
τrot could be calculated as >7 ns at ambient temperatures. The vast discrepancy between the calculated and experimental values of
τrot clearly indicates that the fluorescence anisotropy loss is not due to the rotation of the NCD particles as a whole but rather due to the local reorientational motion of the fluorophore units at the NCD surface. Moreover, the latter is decoupled from the former since there is a ∼10-fold difference in time-scales between the two.
Conclusion
The results presented above help in clarifying the nature of the fluorescence in the NCDs. Firstly, our results help us to conclude that the carbon core is not responsible for the fluorescence emission in our NCDs, in spite of having a strong absorption. This indicates the presence of sites promoting efficient non-radiative decay of the charge carriers produced by π–π* absorption in the core. It is already known that such sites may include the functional groups of the surface fluorophores.46–48 Our results also preclude any FRET-like energy transfer between the charge-carriers and the surface fluorophores, because in that case, core excitation should at least yield surface fluorophore emission, which is not found.
Secondly, once we eliminate the possibility of emission from the core, the fluorescence response of our NCDs must originate predominantly from the surface fluorophores. This automatically implies that the observed REES is due to the heterogeneous nature of the surface fluorophores, and not due to any variation in the core size.
Thirdly, using two different excitation wavelengths (with energies ∼0.5 eV apart), the fluorescence dynamics are not exactly the same. This is further emphasized in the TRANES, where isoemissive points emerged at different emission wavelengths for λex = 375 nm and 450 nm. Therefore, under these two different conditions, different sets of surface fluorophores are excited, which emit independently. The situation is summarized in the scheme given in Fig. 8. Thirdly, the fluorescence anisotropy decay time-constants recorded with λex = 375 nm and 450 nm were found to be much shorter than that expected for rotational diffusion of the carbon core, and rather more comparable to those found for small molecular fluorophores. This confirms that the surface fluorophores behave as small molecular entities attached onto the carbon core. The anisotropy decay rates were similar for λex = 375 nm and 450 nm, indicating that the size of these fluorophores are comparable, even if their natures are different.
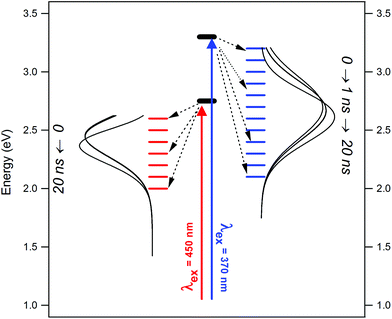 |
| Fig. 8 Scheme depicting the evolution of excited states and TRANES curves for NCDs under different excitation condition. | |
Our results stipulate that the REES observed in NCDs are a consequence of the heterogeneous nature of the surface fluorophores, which have dimensions similar to molecular scales. Different sets of these fluorophores emit independently of the carbon core and even of each other, as indicated by the sharp differences in the TRANES curves obtained with λex = 375 nm and 450 nm. Using several more excitation wavelengths (>300 nm) can lead to a better estimation of the degree of heterogeneity in the surface fluorophores. Another important factor is the nature of the solvent, since interactions between the solvent and the NCD surface will obviously modulate the electronic state of the latter. In the present case, we confined ourselves to only one solvent – DMF – which has been known for ultra-fast solvation dynamics in small, polar fluorophores. Naturally, we did not observe major time-dependent Stokes shift. However, the situation may be modified under conditions of slow, nanosecond-scale solvent relaxation. Moreover, factors like polarity and H-bonding capacity of the solvent may play a significant role in deciding the photophysics of the NCDs. These issues need to be addressed in future work.
Conflicts of interest
There are no conflicts of interest to declare.
Acknowledgements
Financial support of this work was obtained from DST-SERB, India (Project No. EMR/2017/000387), and CSIR, India (Project No. 01(2390)/10/EMR – II). Time-resolved picosecond spectroscopic experiments were carried out at the University of Calcutta with the TCSPC instrument purchased under the DST-FIST Program, India. N. B. thanks University Grants Commission, India, for awarding research fellowship.
References
- L. Gonzalez-Urbina, K. Baert, B. Kolaric, J. Perez-Moreno and K. Clays, Linear and Nonlinear Optical Properties of Colloidal Photonic Crystals, Chem. Rev., 2012, 112, 2268–2285 CrossRef CAS PubMed.
- J. A. Hubbell and A. Chilkoti, Nanomaterials for Drug Delivery, Science, 2012, 337, 303–305 CrossRef PubMed.
- M. Roming, H. Luensdorf, K. E. J. Dittmar and C. Feldmann, ZrO(HPO4)1-x(FMN)x : Quick and Easy Synthesis of a Nanoscale Luminescent Biomarker, Angew. Chem., Int. Ed., 2010, 49, 632–637 CrossRef CAS PubMed.
- M. S. T. Goncalves, Fluorescent Labeling of Biomolecules with Organic Probes, Chem. Rev., 2009, 109, 190–212 CrossRef CAS PubMed.
- J. L. West and N. J. Halas, Engineered Nanomaterials for Biophotonics Applications: Improving Sensing, Imaging, and Therapeutics, Annu. Rev. Biomed. Eng., 2003, 5, 285–292 CrossRef CAS PubMed.
-
P. N. Prasad, Introduction to Biophotonics, Wiley-Interscience, 2003, ISBN: 978-0-471-28770-4 Search PubMed.
- H. Feng and Z. Qian, Functional Carbon Quantum Dots: A Versatile Platform for Chemosensing and Biosensing, Chem. Rec., 2018, 18, 491–505 CrossRef CAS PubMed.
- K. Hola, Y. Zhang, Y. Wang, E. P. Giannelis, R. Zboril and A. L. Rogach, Carbon Dots-Emerging Light Emitters for Bioimaging, Cancer Therapy and Optoelectronics, Nano Today, 2014, 402, 1–14 Search PubMed.
- L. Zheng, Y. Chi, Y. Dong, J. Lin and B. Wang, Electrochemiluminescence of Water-Soluble Carbon Nanocrystals Released Electrochemically from Graphite, J. Am. Chem. Soc., 2009, 131, 4564–4565 CrossRef CAS PubMed.
- S. C. Ray, A. Saha, N. R. Jana and R. Sarkar, Fluorescent Carbon Nanoparticles: Synthesis, Characterization, and Bioimaging Application, J. Phys. Chem. C, 2009, 113, 18546–18551 CrossRef CAS.
- Y.-P. Sun, B. Zhou, Y. Lin, W. Wang, K. A. S. Fernando, P. Pathak, M. J. Meziani, B. A. Harruff, X. Wang, H. F. Wang, P. J. G. Luo, H. Yang, M. E. Kose, B. L. Chen, L. M. Veca and S.-Y. Xie, Quantum-Sized Carbon Dots for Bright and Colorful Photoluminescence, J. Am. Chem. Soc., 2006, 128, 7756–7757 CrossRef CAS PubMed.
- S. N. Baker and G. A. Baker, Luminescent Carbon Nanodots: Emergent Nanolights, Angew. Chem., Int. Ed., 2010, 49, 6726–6744 CrossRef CAS PubMed.
- K. Wang, Z. C. Gao, G. Gao, Y. Wo, Y. Wang, G. Shen and D. Cui, Systematic Safety Evaluation on Photoluminescent Carbon Dots, Nanoscale Res. Lett., 2013, 8, 122–129 CrossRef PubMed.
- C.-C. Fu, H.-Y. Lee, K. Chen, T.-S. Lim, H.-Y. Wu, P.-K. Lin, P.-K. Wei, P.-H. Tsao, H.-C. Chang and W. Fann, Characterization and Application of Single Fluorescent Nanodiamonds as Cellular Biomarkers, Proc. Natl. Acad. Sci. U. S. A., 2007, 104, 727–732 CrossRef CAS PubMed.
- X. Wang, L. Cao, F. Lu, M. J. Meziani, H. Li, G. Qi, B. Zhou, B. A. Harruff, F. Kermarrec and Y.-P. Sun, Photoinduced Electron Transfers with Carbon Dots, Chem. Commun., 2009, 3774–3776 RSC.
- L. Cao, X. Wang, M. J. Meziani, F. Lu, H. Wang, P. G. Luo, Y. Lin, B. A. Harruff, L. M. Veca, D. Murray, S.-Y. Xie and Y.-P. Sun, Carbon Dots for Multiphoton Bioimaging, J. Am. Chem. Soc., 2007, 129, 11318–11319 CrossRef CAS PubMed.
- S.-T. Yang, L. Cao, P. G. Luo, F. Lu, X. Wang, H. Wang, M. J. Meziani, Y. Liu, G. Qi and Y.-P. Sun, Carbon Dots for Optical Imaging in Vivo, J. Am. Chem. Soc., 2009, 131, 11308–11309 CrossRef CAS PubMed.
- R. Liu, D. Wu, S. Liu, K. Koynov, W. Knoll and Q. Li, An Aqueous Route to Multicolor Photoluminescent Carbon Dots Using Silica Spheres as Carriers, Angew. Chem., Int. Ed., 2009, 48, 4598–4601 CrossRef CAS PubMed.
- S. J. Yu, M. W. Kang, H. C. Chang, K. M. Chen and Y. C. Yu, Bright Fluorescent Nanodiamonds: No Photobleaching and Low Cytotoxicity, J. Am. Chem. Soc., 2005, 127, 17604–17605 CrossRef CAS PubMed.
- S.-T. Yang, X. Wang, H. Wang, F. Lu, P. G. Luo, L. Cao, M. J. Meziani, J.-H. Liu, M. Chen, Y. Huang and Y.-P. Sun, Carbon Dots as Nontoxic and High-Performance Fluorescence Imaging Agents, J. Phys. Chem. C, 2009, 113, 18110–18114 CrossRef CAS PubMed.
- C. Tang, J. Zhou, Z. Qian, Y. Ma, Y. Huanga and H. Feng, A Universal Fluorometric Assay Strategy for Glycosidases Based on Functional Carbon Quantum Dots: β-galactosidase Activity Detection in Vitro and in Living Cells, J. Mater. Chem. B, 2017, 5, 1971–1979 RSC.
- G. Chen, H. Feng, X. Jiang, J. Xu, S. Pan and Z. Qian, Redox-Controlled Fluorescent Nanoswitch Based on Reversible Disulfide and Its Application in Butyrylcholinesterase Activity Assay, Anal. Chem., 2018, 90, 1643–1651 CrossRef CAS PubMed.
- H. Peng and J. Travas-Sejdic, Simple Aqueous Solution Route to Luminescent Carbogenic Dots from Carbohydrates, Chem. Mater., 2009, 21, 5563–5565 CrossRef CAS.
- J. Zhang, W. Shen, D. Pan, Z. Zhang, Y. Fang and M. Wu, Controlled Synthesis of Green and Blue Luminescent Carbon Nanoparticles with High Yields by the Carbonization of Sucrose, New J. Chem., 2010, 34, 591–593 RSC.
- H. Zhu, X. Wang, Y. Li, Z. Wang, F. Yang and X. Yang, Microwave Synthesis of Fluorescent Carbon Nanoparticles with Electrochemiluminescence Properties, Chem. Commun., 2009, 5118–5120 RSC.
- X. Xu, R. Ray, Y. Gu, H. J. Ploehn, L. Gearheart, K. Raker and W. A. Scrivens, Electrophoretic Analysis and Purification of Fluorescent Single-Walled Carbon Nanotube Fragments, J. Am. Chem. Soc., 2004, 126, 12736–12737 CrossRef CAS PubMed.
- H. Liu, T. Ye and C. Mao, Fluorescent Carbon Nanoparticles Derived from Candle Soot, Angew. Chem., Int. Ed., 2007, 46, 6473–6475 CrossRef CAS PubMed.
- Z.-A. Qiao, Y. Wang, Y. Gao, H. Li, T. Dai, Y. Liu and Q. Huo, Commercially Activated Carbon as the Source for Producing Multicolor Photoluminescent Carbon Dots by Chemical Oxidation, Chem. Commun., 2010, 46, 8812–8814 RSC.
- J.-L. Chen and X.-P. Yan, Ionic Strength and pH Reversible Response of Visible and Near-infrared Fluorescence of Graphene Oxide Nanosheets for Monitoring the Extracellular pH, Chem. Commun., 2011, 47, 3135–3137 RSC.
- A. B. Bourlinos, A. Stassinopoulos, D. Anglos, R. Zboril, V. Georgakilas and E. P. Giannelis, Photoluminescent Carbogenic Dots, Chem. Mater., 2008, 20, 4539–4541 CrossRef CAS.
- F. Wang, S. Pang, L. Wang, Q. Li, M. Kreiter and C. Liu, One-Step Synthesis of Highly Luminescent Carbon Dots in Noncoordinating Solvents, Chem. Mater., 2010, 22, 4528–4530 CrossRef CAS.
- S. Liu, L. Wang, J. Tian, J. Zhai, Y. Luo, W. Lua and X. Sun, Acid-driven, Microwave-Assisted Production of Photoluminescent Carbon Nitride dots from N,N-dimethylformamide, RSC Adv., 2011, 1, 951–953 RSC.
- X. Wang, K. Qu, B. Xu, J. Rena and X. Qu, Microwave Assisted One-step Green Synthesis of Cell-permeable Multicolor Photoluminescent Carbon Dots without Surface Passivation Reagents, J. Mater. Chem., 2011, 21, 2445–2450 RSC.
- A. Jaiswal, S. S. Ghosh and A. Chattopadhyay, One Step Synthesis of C-dots by Microwave Mediated Caramelization of Poly(Ethylene Glycol), Chem. Commun., 2012, 48, 407–409 RSC.
- J. Jiang, Y. He, S. Li and H. Cui, Amino Acids as the Source for Producing Carbon Nanodots: Microwave Assisted One-step Synthesis, Intrinsic Photoluminescence Property and Intense Chemiluminescence Enhancement, Chem. Commun., 2012, 48, 9634–9636 RSC.
- M. O. Dekaliuk, O. Viagin, M. Malyukin and A. P. Demchenko, Fluorescent Carbon Nanomaterials: “Quantum Dots” or Nanoclusters?, Phys. Chem. Chem. Phys., 2014, 16, 16075–16084 RSC.
- N. Dhenadhayalan, K.-C. Lin, R. Suresh and P. Ramamurthy, Unravelling the Multiple Emissive States in Citric Acid-Derived Carbon-Dots, J. Phys. Chem. C, 2016, 120, 1252–1261 CrossRef CAS.
- M. J. Krysmann, A. Kelarakis, P. Dallas and E. P. Giannelis, Formation Mechanism of Carbogenic Nanoparticles with Dual Photoluminescence Emission, J. Am. Chem. Soc., 2012, 134, 747–750 CrossRef CAS PubMed.
- S. Zhu, Y. Song, X. Zhao, J. Shao, J. Zhang and B. Yang, The Photoluminescence Mechanism in Carbon Dots (Graphene Quantum Dots, Carbon Nanodots, and Polymer Dots): Current State and Future Perspective, Nano Res., 2015, 8, 355–381 CrossRef CAS.
- H. Li, X. He, Z. Kang, H. Huang, Y. Liu, J. Liu, S. Lian, C. H. A. Tsang, X. Yang and S.-T. Lee, Water-Soluble Fluorescent Carbon Quantum Dots and Photocatalyst Design, Angew. Chem., Int. Ed., 2010, 49, 4430–4434 CrossRef CAS PubMed.
- S. Qu, X. Wang, Q. Lu, X. Liu and L. Wang, A Biocompatible Fluorescent Ink Based on Water-Soluble Luminescent Carbon Nanodots, Angew. Chem., Int. Ed., 2012, 51, 12215–12218 CrossRef CAS PubMed.
- W. Kwon and S.-W. Rhee, Facile Synthesis of Graphitic Carbon Quantum Dots with Size Tunability and Uniformity Using Reverse Micelles, Chem. Commun., 2012, 48, 5256–5258 RSC.
- T. Gokus, R. R. Nair, A. Bonetti, M. Böhmler, A. Lombardo, K. S. Novoselov, A. K. Geim, A. C. Ferrari and A. Hartschuh, Making Graphene Luminescent by Oxygen Plasma Treatment, ACS Nano, 2009, 3, 3963–3968 CrossRef CAS PubMed.
- D. Pan, L. Guo, J. Zhang, C. Xi, Q. Xue, H. Huang, J. Li, Z. Zhang, W. Yu, Z. Chen, Z. Li and M. Wu, Cutting sp2 Clusters in Graphene Sheets into Colloidal Graphene Quantum Dots with Strong Green Fluorescence, J. Mater. Chem., 2012, 22, 3314–3318 RSC.
- R. Liu, D. Wu, X. Feng and K. Müllen, Bottom-Up Fabrication of Photoluminescent Graphene Quantum Dots with Uniform Morphology, J. Am. Chem. Soc., 2011, 133, 15221–15223 CrossRef CAS PubMed.
- L. Li, G. Wu, G. Yang, J. Peng, J. Zhao and J.-J. Zhu, Focusing on Luminescent Graphene Quantum Dots: Current Status and Future Perspectives, Nanoscale, 2013, 5, 4015–4039 RSC.
- S. Zhu, Q. Meng, L. Wang, J. Zhang, Y. Song, H. Jin, K. Zhang, H. Sun, H. Wang and B. Yang, Highly Photoluminescent Carbon Dots for Multicolor Patterning, Sensors, and Bioimaging, Angew. Chem., Int. Ed., 2013, 52, 3953–3957 CrossRef CAS PubMed.
- Y.-M. Long, C.-H. Zhou, Z.-L. Zhang, Z.-Q. Tian, L. Bao, Y. Lin and D.-W. Pang, Shifting and Non-shifting Fluorescence Emitted by Carbon Nanodots, J. Mater. Chem., 2012, 22, 5917–5920 RSC.
- Y.-F. Hsu, Y.-H. Chen and C.-W. Chang, The Spectral Heterogeneity and Size Distribution of the Carbon Dots Derived from Time-resolved Fluorescence Studies, Phys. Chem. Chem. Phys., 2016, 18, 30086–30092 RSC.
- L. Liu, D. Ma, H. Zheng, X. Li, M. Cheng and X. Bao, Synthesis and Characterization of Microporous Carbon Nitride, Microporous Mesoporous Mater., 2008, 110, 216–222 CrossRef CAS.
- K. K. R. Datta, B. V. S. Reddy, K. Ariga and A. Vinu, Gold Nanoparticles Embedded in a Mesoporous Carbon Nitride Stabilizer for Highly Efficient Three-Component Coupling Reaction, Angew. Chem., Int. Ed., 2010, 49, 5961–5965 CrossRef CAS PubMed.
- Y. Qiu and L. Gao, Chemical Synthesis of Turbostratic Carbon Nitride, Containing C–N Crystallites, at Atmospheric Pressure, Chem. Commun., 2003, 2378–2379 RSC.
- A. Y. Liu and M. L. Cohen, Prediction of New Low Compressibility Solids, Science, 1989, 245, 841–842 CrossRef CAS PubMed.
- Y.-J. Bai, B. Lu, Z.-G. Liu, L. Li, D.-L. Cui, X.-G. Xu and Q.-L. Wang, Solvothermal Preparation of Ggraphite-like C3N4 Nanocrystals, J. Cryst. Growth, 2003, 247, 505–508 CrossRef CAS.
- L. Yang, P. W. May, Y. Huang and L. Yin, Hierarchical Architecture of Self-assembled Carbon Nitride Nanocrystals, J. Mater. Chem., 2007, 17, 1255–1257 RSC.
- K. Ghosh, M. Kumar, H. Wang, T. Maruyama and Y. Ando, Facile Decoration of Platinum Nanoparticles on Carbon-Nitride Nanotubes via Microwave-Assisted Chemical Reduction and Their Optimization for Field-Emission Application, J. Phys. Chem. C, 2010, 114, 5107–5112 CrossRef CAS.
- Z. Qian, J. Ma, X. Shan, H. Feng, L. Shao and J. Chen, Highly Luminescent N-Doped Carbon Quantum Dots as an Effective Multifunctional Fluorescence Sensing Platform, Chem. – Eur. J., 2014, 20, 2254–2263 CrossRef CAS PubMed.
- Y. Xu, M. Wu, Y. Liu, X.-Z. Feng, X.-B. Yin, X.-W. He and Y.-K. Zhang, Nitrogen-Doped Carbon Dots: A Facile and General Preparation Method, Photoluminescence Investigation, and Imaging Applications, Chem. – Eur. J., 2013, 19, 2276–2283 CrossRef CAS PubMed.
- Z. Jiang, A. Nolan, J. G. A. Walton, A. Lilienkampf, R. Zhang and M. Bradley, Photoluminescent Carbon Dots from 1,4-Addition Polymers, Chem. – Eur. J., 2014, 20, 2276–2283 CrossRef PubMed.
- V. Strauss, J. T. Margraf, C. Dolle, B. Butz, T. J. Nacken, J. Walter, W. Bauer, W. Peukert, E. Spiecker, T. Clark and D. M. Guldi, Carbon Nanodots: Toward a Comprehensive Understanding of Their Photoluminescence, J. Am. Chem. Soc., 2014, 136, 17308–17316 CrossRef CAS PubMed.
- K. Suda, M. Terazima, H. Sato and Y. Kimura, Excitation Wavelength Dependence of Excited State Intramolecular Proton Transfer Reaction of 4′-N,N-Diethylamino-3-hydroxyflavone in Room Temperature Ionic Liquids Studied by Optical Kerr Gate Fluorescence Measurement, J. Phys. Chem. B, 2013, 117, 12567–12582 CrossRef CAS PubMed.
- A. S. R. Koti and N. Periasamy, Application of Time Resolved Area Normalized Emission Spectroscopy to Multicomponent Systems, J. Chem. Phys., 2001, 115, 7094–7099 CrossRef CAS.
Footnote |
† Electronic supplementary information (ESI) available. See DOI: 10.1039/c8pp00077h |
|
This journal is © The Royal Society of Chemistry and Owner Societies 2019 |
Click here to see how this site uses Cookies. View our privacy policy here.