DOI:
10.1039/C7NJ03522E
(Paper)
New J. Chem., 2018,
42, 395-401
New porphyrin-based dendrimers with alkene linked fluorenyl antennae for optics†
Received
18th September 2017
, Accepted 16th November 2017
First published on 16th November 2017
Abstract
The design of porphyrin-based dendrimers featuring conjugated dendrons via alkene spacers herein demonstrates their important role in determining linear optical properties, as well as two-photon absorption (PA) and singlet oxygen photosensitising properties. Improvement in these properties compared to those observed for their analogous alkyne spacers makes these molecules appealing for combined 2-photon-based photodynamic therapy (PDT) and imaging.
Introduction
The capacity of porphyrin-based molecular and supramolecular systems to exhibit special photophysical properties makes the continued development of design principles important in order to promote their potential applications in materials science and anti-cancer therapy.1 In particular the generation of singlet oxygen (1O2) upon photo excitation has been studied for applications in photodynamic therapy (PDT),2 leading eventually to the clinical use of selected porphyrin derivatives such as photofrin® or visudyne®.3 Further advances in this field now focus on the development of photosensitizers possessing larger two-photon (2PA) absorption cross-sections, likely to be excited with NIR lasers,3,4 thereby allowing for (i) enhanced penetrability in tissues of the exciting beam, (ii) better spatial control of the 1O2 generation and overall (iii) lower photo damage to tissue compared to usual visible excitation.5 Accordingly, various porphyrin arrays with giant 2PA cross-sections and impressive photosensitizing activities have been reported.6 However, for several of them,6a,c,d the 2PA enhancement results from quasi-resonance of the exciting beam with one-photon absorbing (1PA) states at low energy, potentially limiting the achievement of an optimal 3D-spatial resolution.
To avoid any interference with 1PA states at low energies, we have focussed our efforts on more classical meso-tetra-arylporphyrin (TAP) cores, functionalized with fluorenyl-containing conjugated dendrons at their periphery, such as 1a or 2a (Scheme 1).7 In these semi-disconnected π-structures,8 the peripheral dendrons are only partly conjugated with the lower central porphyrin core due to tilting of the meso-aryl rings for sterical reasons.7a Thus, while roughly presenting the linear optical signature and properties of an unsubstituted TAP core, this kind of structure has usually a larger transparency window than fully conjugated structures and the porphyrin-centered excited state active for oxygen sensitization can still be populated by resonant energy transfer following mono- or bi-photonic excitation of the peripheral dendrons.9 In addition, this kind of structure also permits an overall faster radiative decay, preserving (or even improving) the fluorescence of the porphyrin core over that of the parent tetraphenylporphyrin (TPP) molecule (11%).7 By comparison, apart from rare exceptions,6e most of the related porphyrin-based systems with higher 2PA cross-sections reported so far6a–d,10 present modest to negligible fluorescence or interfering residual one-photon absorption near 800 nm.
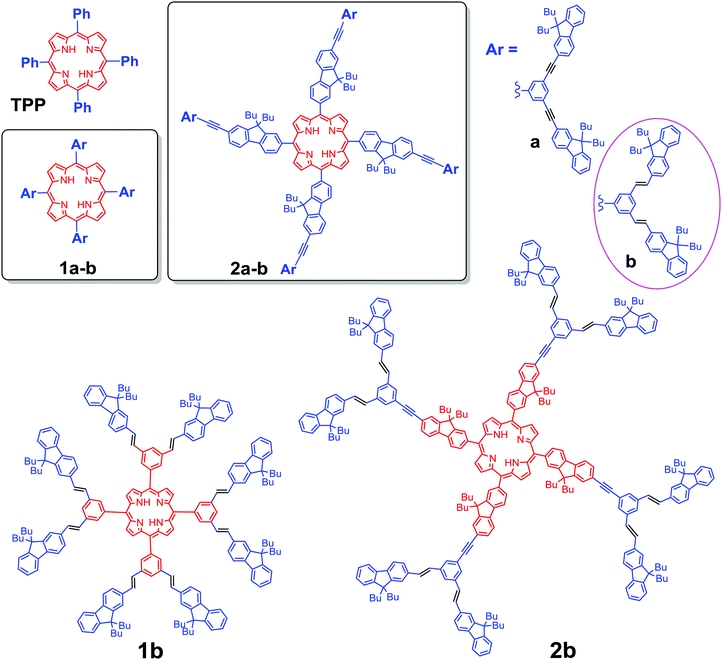 |
| Scheme 1 Known (1a and 2a) and targeted meso-tetraarylporphyrin-based dendrimers (1b and 2b). | |
Molecules such as 1a or 2a would therefore present appealing potential for achieving combined two-photon-based PDT and imaging, i.e. for theranostics,11 provided that additional key features such as water-solubility and photostability can be imparted to these remarkable molecular architectures without detrimentally affecting their photophysical properties. In order to gain additional insights into how a definite structural change of the peripheral light-harvesting antenna will affect their photophysical properties we have examined the impact of several kinds of structural modifications.12 Lately, given that alkene spacers usually allow a better electronic communication between the terminal moieties than alkynes,13 we have explored the impact of replacing some of the alkynes by alkenes.
Results and discussion
Dendron formation
Aldehyde D-PhCHO was obtained according to the classic procedures.7,14a Then by taking this dendron D-PhCHO as a starting material, synthesis of new aldehyde dendron D-FlCHO is described in Scheme 2a. D-FlCHO is obtained by successive reactions: (1) the aldehyde group of D-PhCHO will react to first create an intermediate dibromovinyl compound and then an alkynyl-intermediate after two steps of the Corey-Fuchs reaction14b–d (74% yield for the first step, 93% yield for the second one); (2) then bromo-fluorene aldehyde reacts with this alkynyl-intermediate through Sonogashira coupling14e,f (59% yield) to give desired conjugated aldehyde dendron D-FlCHO (see Scheme 2a).
 |
| Scheme 2 (a) Synthesis of dendron D-FlCHO and (b) new vinyl-bridged porphyrin dendrimers (D series) based on TPP-cored and TFP-cored porphyrins (1b and 2b). | |
Porphyrin synthesis
Nowadays, two methods are the most used to synthesize a porphyrin core: Adler–Longo14g and Lindsey,14h both of which are efficient for synthesizing porphyrins substituted at the meso positions. However, we noticed that our target porphyrins could not be obtained under Lindsey conditions, only black polymers being obtained instead. In contrast, Adler–Longo conditions14g could be successfully utilized to synthesize the targeted porphyrins but the yields were still low (see Scheme 2b). Consequently, the new compounds 1b and 2b were obtained in 8% and 1% yield, respectively, in one step from the corresponding functional aldehydes (D-PhCHO or D-FlCHO) and pyrrole in propionic acid under typical Adler–Longo conditions.14g The resulting porphyrins were completely characterized using HRMS, 1H and 13C NMR (see the ESI†).
Optical properties
After having synthesized and studied the new porphyrin-based dendrimers 1b and 2b (Scheme 2), the significant improvement in their linear and nonlinear optical properties compared to those previously reported for 1a and 2a7 prompts us to communicate our results here.
Absorption properties
Their linear optical properties were subsequently studied (Fig. 1). In line with data previously gathered for related structures,7 these molecules display typical features of the TAP core such as an intense Soret band near 430 nm and four weaker Q-bands in the 518–652 nm range along with a strong structured band near 350 nm corresponding to the first fluorene-based π* ← π transition(s). As expected, the intensity of the latter remains fairly similar between 1a–b and 2a–b (which contain the same number of fluorenyl groups), but undergoes a slight bathochromic shift when progressing from triple to double bond-containing dendrons, in line with a better π-conjugation in the latter case. Transitions more localized on the porphyrin ring such as the Soret band or Q bands shift also to the red, but to a smaller extent in 1a–b.
 |
| Fig. 1 Absorption and normalized emission spectra of 1b–2bvs.1a–2a and TPP in CH2Cl2. | |
Emission properties
When excited at 350 nm, both 1b and 2b fluoresce in the red, corresponding to an emission from the Q-states which are the first singlet excited states. The absence of any fluorescence from the fluorenyl-based π* ← π state points to a nearly quantitative intramolecular energy transfer to the Q-states, recalling the process already evidenced for 1a and 2a.
The fluorescence quantum yields derived for 1b and 2b were shown to be always larger than for TPP (Table 1). While that of 1b is slightly superior to that of 1a, that found for 2b is lower than that of 2a.
Table 1 Photophysical data in dichloromethane of selected porphyrin derivativesa
Cpnd |
Fluorenes/porphyrin |
λ
maxabs (nm)
UV band
|
λ
maxabs (nm)
Soret
|
λ
maxabs (nm)
Q-bands
|
λ
em (nm) |
Φ
F
|
Φ
Δ
|
1 − ΦF − ΦΔc |
λ
max2PA (nm) |
σ
max2 d (GM) |
Enhancement factore |
Fluorescence quantum yield determined relative to TPP in toluene (ΦF = 11%), upon excitation at the Soret band.
1O2 formation quantum yield determined relative to TPP in CH2Cl2 (ΦΔ[TPP] = 0.60).
Upper bound yield of non radiative decay processes other than intersystem crossing.
Intrinsic 2PA cross-sections measured by 2PEF in the fs regime; a fully quadratic dependence of the fluorescence intensity on the excitation power is observed and 2PA responses are fully non-resonant.
Two-photon excited singlet oxygen generation ΦΔ·σmax2 figure of merit relative to that for TPP.
Data from ref. 15.
Data from ref. 7.
|
TPP
|
0 |
— |
418 |
514, 549, 590, 647 |
650, 716 |
0.11 |
0.60 |
0.29 |
790 |
12f |
1 |
1a
|
8 |
326 |
426 |
517, 552, 589, 646 |
650, 718 |
0.12 |
0.59 |
0.29 |
790 |
200 |
16 |
2a
|
12 |
340 |
431 |
521, 559, 594, 652 |
660, 726 |
0.23 |
0.61 |
0.16 |
790 |
730 |
62 |
1b
|
8
|
349
|
434
|
518, 552, 593, 649
|
652, 719
|
0.13
|
0.64
|
0.23
|
790
|
280
|
25
|
2b
|
12
|
351
|
431
|
520, 557, 597, 652
|
659, 725
|
0.17
|
0.62
|
0.21
|
790
|
1010
|
87
|
Two-photon absorption
The 2PA cross-sections were then measured for 1b and 2b by two-photon excitation fluorescence (2PEF). These are one or two orders of magnitude larger than that of TPP (Fig. 2). In line with our expectations, the presence of the double bonds leads to an improvement of about 40% of the effective 2PA cross-sections at 790 nm compared to 1a–2a (Table 1), while keeping a strict quadratic dependence on the power of the incoming laser beam, in line with a “pure” 2PA phenomenon.
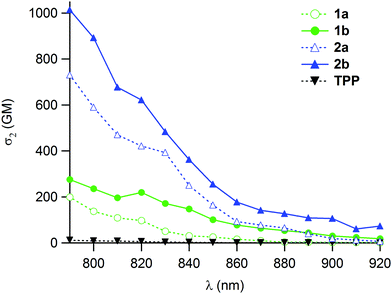 |
| Fig. 2 Two-photon absorption spectra of the new porphyrin dendrimers 1b–2b (solid lines) vs. those of 1a–2a and reference TPP (dotted lines) in CH2Cl2. | |
Comparison with 1a–2a further reveals that in spite of the slight decrease in fluorescence quantum yield stated for 2b relative to 2a, the two-photon brightness (ΦF·σmax2) is significantly improved for both 1b and 2b.
Singlet oxygen generation
Finally, the quantum yields for singlet oxygen generation were determined for 1b and 2b (ESI†) and each of them are better than those of their analogues containing triple bonds instead of alkene spacers (1a–2a). For 1b and 2b, this leads to a remarkable 25- and 87-fold enhancement, respectively, of the ΦΔ·σmax2 factor taken as a figure of merit for two-photon singlet oxygen generation over TPP, while 16- to 62-fold enhancements had been previously obtained for 1a and 2a (Table 1), outlining the interest of these compounds as biphotonic sensitizers for photodynamic therapy.
These enhancements evidence the positive role of double over triple bonds when used as internal spacers within fluorene-containing dendrons for significantly enhancing the 2PA cross-sections while marginally affecting the fluorescence yields and singlet-oxygen formation of the compounds. This positive effect likely originates from the improved π-conjugation between the peripheral fluorenyl units and the central TPP core in 1b–2b.16
Conclusions
We report here the photochemical study of two new TAP-based dendritic chromophores (1b–2b) possessing 8 to 12 fluorenyl groups at their periphery. These are analogues of related dendrimers (1a–2a) presenting the so-called “semi-disconnected” peripheral arms, in which we have now replaced the alkyne linkages by E-alkene ones at their periphery (Scheme 1). As for the T series (1a–2a), the optical properties of the new D series (1b–2b) exhibit an obvious dependence on the central TAP core, TFP cores being clearly better than TPP ones for enhancing the properties of interest (luminescence, 2PA cross-section and sensitization yields).
In comparison to 1a–2a, the new D derivatives possess a much improved 1O2 generation capability after two-photon excitation and better or comparable two-photon brightness. Thus, the new double-bonded dendrimers 1b–2b present intrinsic two-photon absorption enhanced by one to two orders of magnitude in comparison with TPP, and also significantly enlarged compared to their triple-bonded analogues. As a result, their 2PA cross-sections at 790 nm and their specific red fluorescence supersede those of many TPP-based photosensitisers developed so far. Furthermore, a strict quadratic dependence of the two-photon excited fluorescence (2PEF) intensity on the fluence of the exciting laser beam was established in all cases, allowing optimal spatial resolution to be expected during imaging or therapy. Presently, the best trade-off between two-photon brightness and sensitization is achieved for 2b. When considering its performances, such a kind of structure appears to be quite appealing for obtaining photosensitisers. This work also discloses a new and valuable design criterion with which the photosensitizing performances of porphyrin-based conjugated dendrimers containing related numbers of peripheral fluorene units can be readily improved.
Experimental section
General
Unless otherwise stated, all solvents used in reactions were distilled using common purification protocols,17 except DMF and iPr2NH which were dried on molecular sieves (3 Å). 1H and 13C NMR spectra were recorded on a BRUKER Ascend 400 and 500 MHz at 298 K. The chemical shifts are referenced to internal tetramethylsilane. High-resolution mass spectra were recorded on a Bruker MicrOTOF-Q II in the ESI positive mode in a dried solvent at CRMPO in Rennes. Reagents were purchased from commercial suppliers and used as received. 7-Bromo-9,9-dibutyl-fluorene-2-carbaldehyde was synthesized as described earlier.7b Aldehyde D-PhCHO was obtained according to the classic procedures.7,14a
Synthesis of D-FlCHO (Scheme 2a)
2,2′-(5-(2,2-Dibromovinyl)-1,3-phenylene)bis(ethene-2,1-diyl)bis(9,9-dibutyl-9H-fluorene).
In a Schlenk tube, to a mixture of PPh3 (221 mg, 0.84 mmol, 2 eq.) and Zn powder (55 mg, 0.84 mmol, 2 eq.) dry CH2Cl2 (4 mL) was added. After cooling to 0 °C with an ice-water bath, CBr4 (280 mg, 0.84 mmol, 2 eq.) was added under argon protection and kept at low temperature for 2 min. Then the bath was removed and the mixture was stirred overnight at room temperature. The mixture was cooled again to 0 °C, and a solution of D-PhCHO7,14a (300 mg, 0.42 mmol, 1 eq.) in dry CH2Cl2 (4 mL) was injected into the Schlenk tube still under argon protection. We kept the low temperature (0 °C) for 10 min and stirred it for 4 h in dark at room temperature. Then we filtered the solution and evaporated the CH2Cl2. This dry residue was absorbed in silica and further purified using chromatography (heptane
:
CH2Cl2 = 100
:
5), finally we collected a white powder (270 mg, 74% yield). 1H NMR (400 MHz, CDCl3, ppm): δ = 7.71 (d, J = 7.6 Hz, 5H), 7.60 (s, 2H), 7.56 (s, 1H), 7.54 (d, J = 7.6 Hz, 2H), 7.51 (s, 2H), 7.37–7.30 (m, 7H), 7.27 (s, 1H), 7.22–7.18 (m, 2H), 2.02 (t, J = 8.0 Hz, 8H), 1.15–1.06 (m, 8H), 0.71–0.54 (m, 20H).
2,2′-(1E,1′E)-2,2′-(5-Ethynyl-1,3-phenylene)bis(ethene-2,1-diyl)bis(9,9-dibutyl-9H-fluorene).
N-BuLi (0.58 mL, 0.93 mmol, 3 eq.) was added to a cold (−78 °C) solution of iPr2NH (0.13 mL, 0.93 mmol, 3 eq.) in dry THF (4 mL). After warming up to room temperature, the mixture was injected dropwise into the solution of 2,2′-(5-(2,2-dibromovinyl)-1,3-phenylene)bis(ethene-2,1-diyl)bis(9,9-dibutyl-9H-fluorene) (270 mg, 0.31 mmol, 1 eq.) in dry THF (8 mL) at −78 °C (liquid nitrogen–acetone bath). The low temperature was kept for 1 h, and then the reaction was quenched with saturated NH4Cl (2 mL). We removed the bath; it was stirred until the temperature decreased to room temperature. We extracted it with ethyl acetate and evaporated solvents. Then the dry residue was absorbed on silica and further purified using chromatography (heptane
:
CH2Cl2 = 100
:
5), we obtained a white powder (205 mg, 93% yield). 1H NMR (400 MHz, CDCl3, ppm): δ = 7.71 (d, J = 7.6 Hz, 5H), 7.60 (s, 2H), 7.53 (d, J = 7.6 Hz, 2H), 7.50 (s, 2H), 7.37–7.29 (m, 7H), 7.27 (s, 1H), 7.17–7.13 (m, 2H), 3.12 (s, 1H), 2.01 (t, J = 8.0 Hz, 8H), 1.15–1.06 (m, 8H), 0.71–0.58 (m, 20H).
Dendron D-FlCHO.
In a Schlenk tube, to a mixture of 7-bromo-9,9-dibutyl-fluorene-2carbaldehyde (101 mg, 0.26 mmol, 1 eq.), 2,2′-(1E,1′E)-2,2′-(5-ethynyl-1,3-phenylene)bis(ethene-2,1-diyl)bis(9,9-dibutyl-9H-fluorene) (205 mg, 0.29 mmol, 1.1 eq.), Pd(PPh3)2Cl2 (12 mg, 0.017 mmol, 6% eq.) and CuI (1.6 mg, 0.009 mmol, 3% eq.), DMF (10 mL) and iPr2NH (10 mL) were added under argon. Then the system was degassed by the freeze–pump–thaw cycle twice and heated for 90 h at 95 °C. After being evaporated, the residue was absorbed on silica and further purified using chromatography (petroleum ether
:
CH2Cl2 = 4
:
1), showing white powder (170 mg, 65% yield). 1H NMR (400 MHz, CDCl3, ppm): δ = 10.08 (s, 1H), 7.90–7.85 (m, 3H), 7.80 (d, J = 8.0 Hz, 1H), 7.73–7.69 (m, 6H), 7.63–7.60 (m, 2H), 7.55 (d, J = 7.6 Hz, 2H), 7.52 (s, 2H), 7.37–7.30 (m, 9H), 7.22–7.18 (m, 2H), 2.09–2.00 (m, 12H), 1.16–1.07 (m, 12H), 0.72–0.51 (m, 30H).
Synthesis of porphyrins (Scheme 2b)
Porphyrin 1b.
A mixture of D-PhCHO (230 mg, 0.32 mmol, 1 eq.) and propionic acid (2 mL) was heated to 120 °C. Pyrrole (0.02 mL, 0.32 mmol, 1 eq.) in propionic acid (1 mL) was then added dropwise to the mixture and the reaction was kept under reflux for 5.5 h. After cooling to room temperature, MeOH was added to the reaction mixture and the precipitate was filtered. The residue was purified by chromatography using petroleum ether/CH2Cl2 (5
:
1) as an eluent. After evacuation of the volatiles under vacuum, a crude red powder was obtained. The title porphyrin was obtained as a dark red powder (19 mg, 8% yield) after recrystallisation of the crude residue from refluxing CHCl3/MeOH (1
:
1) mixtures. 1H NMR (400 MHz, CDCl3, ppm): δ = 9.06 (s, 8H), 8.38 (s, 8H), 8.19 (s, 4H), 7.69–7.67 (m, 16H), 7.57 (s, 4H), 7.55 (s, 12H), 7.50 (s, 16H), 7.31–7.28 (m, 24H), 1.97 (t, J = 8.0 Hz, 32H), 1.08–1.02 (m, 32H), 0.65–0.54 (m, 80H), −2.57 (s, 2H). 13C NMR (125 MHz, CDCl3, ppm): δ = 151.3, 151.0, 143.0, 141.2, 140.8, 136.3, 136.1, 132.0, 130.7, 127.6, 127.1, 126.8, 125.8, 124.0, 122.8, 120.8, 119.9, 119.7, 54.9, 40.3, 25.9, 23.1, 13.8. HRMS-ESI: m/z = 3032.8723 [M + H]+ (calcd: 3032.8819).
Porphyrin 2b.
A mixture of D-FlCHO (170 mg, 0.19 mmol, 1 eq.) and propionic acid (5 mL) was heated to 120 °C. Pyrrole (0.01 mL, 0.19 mmol, 1 eq.) in propionic acid (0.5 mL) was then added dropwise to the mixture and the reaction was kept under reflux for 5 h. After cooling to room temperature, MeOH was added to the reaction mixture and the precipitate was filtered. The residue was purified by chromatography using petroleum ether/CH2Cl2 (5
:
1) as an eluent. After evacuation of the volatiles under vacuum, a crude red powder was obtained. The title porphyrin was obtained as a red powder (2 mg, 1% yield). 1H NMR (400 MHz, CDCl3, ppm): δ = 8.95 (s, 8H), 8.27–8.24 (m, 8H), 8.12 (d, J = 7.2 Hz, 4H), 7.99 (d, J = 8.0 Hz, 4H), 7.74–7.72 (m, 32H), 7.57 (d, J = 8.0 Hz, 8H), 7.54 (s, 8H), 7.38–7.30 (m, 36H), 7.24–7.20 (m, 8H), 2.20 (broad, 16H), 2.02 (t, J = 8.0 Hz, 32H), 1.26–1.22 (m, 16H), 1.16–1.07 (m, 32H), 1.03–0.95 (m, broad, 16H), 0.84–0.78 (m, 24H), 0.70 (t, J = 7.2 Hz, 48H), 0.67–0.58 (m, 32H), −2.55 (s, 2H). 13C NMR (125 MHz, CDCl3, ppm): δ = 151.3, 151.0, 141.5, 141.3, 140.8, 138.2, 136.0, 133.9, 130.5, 128.4, 127.2, 126.8, 125.7, 124.2, 124.0, 123.1, 122.9, 121.0, 120.0, 119.8, 92.1, 55.0, 40.3, 26.0, 23.1, 14.1, 14.0, 13.8. HRMS-ESI: m/z = 4233.710 [M + H]+ (calcd: 4233.6331).
Spectroscopic measurements
General data
All the photophysical properties have been performed with freshly-prepared air-equilibrated solutions at room temperature (298 K). UV/Vis absorption spectra were recorded on a Jasco V-570 spectrophotometer or a BIO-TEK instrument UVIKON XL spectrometer at room temperature. Fluorescence measurements were performed on dilute solutions (ca. 10−6 M, optical density < 0.1) contained in standard 1 cm quartz cuvettes using an Edinburgh Instruments FLS920 spectrometer (Xe900) in the photon-counting mode. Fully corrected emission spectra were obtained, for each compound, under excitation at a wavelength of the absorption maximum, with Aλex < 0.1 to minimize internal absorption.
Two-photon absorption experiments
To span the 790–920 nm range, a Nd:YLF-pumped Ti:sapphire oscillator (Chameleon Ultra, Coherent) was used generating 140 fs pulses at a 80 MHz rate. The excitation power is controlled using neutral density filters of varying optical density mounted in a computer-controlled filter wheel. After five-fold expansion through two achromatic doublets, the laser beam is focused by a microscope objective (10×, NA 0.25, Olympus, Japan) into a standard 1 cm absorption cuvette containing the sample. The applied average laser power arriving at the sample is typically between 0.5 and 40 mW, leading to a time-averaged light flux in the focal volume on the order of 0.1–10 mW mm−2. The fluorescence from the sample is collected in the epifluorescence mode, through the microscope objective, and reflected by a dichroic mirror (Chroma Technology Corporation, USA; ‘‘blue’’ filter set: 675dcxru; ‘‘red’’ filter set: 780dxcrr). This makes it possible to avoid the inner filter effects related to the high dye concentrations used (10−4 M) by focusing the laser near the cuvette window. Residual excitation light is removed using a barrier filter (Chroma Technology; ‘‘blue’’: e650-2p, ‘‘red’’: e750sp-2p). The fluorescence is coupled into a 600 μm multimode fiber by an achromatic doublet. The fiber is connected to a compact CCD-based spectrometer (BTC112-E, B&WTek, USA), which measures the two-photon excited emission spectrum. The emission spectra are corrected for the wavelength-dependence of the detection efficiency using correction factors established through the measurement of reference compounds having known fluorescence emission spectra. Briefly, the set-up allows for the recording of corrected fluorescence emission spectra under multiphoton excitation at variable excitation power and wavelength. 2PA cross sections (σ2) were determined from the two-photon excited fluorescence (2PEF) cross sections (σ2·ΦF) and the fluorescence emission quantum yield (ΦF). 2PEF cross sections of 10−4 M dichloromethane solutions were measured relative to fluorescein in 0.01 M aqueous NaOH using the well-established method described by Xu and Webb18 and the appropriate solvent-related refractive index corrections.19 The quadratic dependence of the fluorescence intensity on the excitation power was checked for each sample and all wavelengths.
Measurements of singlet oxygen quantum yield (ΦΔ)
Measurements were performed on a Fluorolog-3 (Horiba Jobin Yvon), using a 450 W Xenon lamp. The emission at 1272 nm was detected using a liquid nitrogen-cooled Ge-detector model (EO-817L, North Coast Scientific Co). Singlet oxygen quantum yields ΦΔ were determined in dichloromethane solutions, using tetraphenylporphyrin (TPP) in dichloromethane as the reference solution (ΦΔ[TPP] = 0.60) and were estimated from 1O2 luminescence at 1272 nm.
Conflicts of interest
There are no conflicts to declare.
Acknowledgements
DY, XZ and LS thank the China Scholarship Council (CSC) for a PhD grant, SA thanks the “Ministère de l’Enseignement Supérieur et de la Recherche Scientifique de Tunisie” for PhD funding. This project was supported by the departmental committees CD35 and CD85 of the “Ligue contre le Cancer du Grand-Ouest”.
References
- K. Szaciłowski, W. Macyk, A. Drzewiecka-Matuszek, M. Brindell and G. Stochel, Chem. Rev., 2005, 105, 2647 CrossRef PubMed.
- J. F. Lovell, T. W. B. Liu, J. Chen and G. Zheng, Chem. Rev., 2010, 110, 2839 CrossRef CAS PubMed.
-
(a) A. Karotki, M. Khurana, J. R. Lepock and B. C. Wilson, Photochem. Photobiol., 2006, 82, 443 CrossRef CAS PubMed;
(b) M. Khurana, H. A. Collins, A. Karotki, H. L. Anderson, D. T. Cramb and B. C. Wilson, Photochem. Photobiol., 2007, 83, 1441 CrossRef CAS PubMed.
-
(a) J. D. Bhawalkar, N. D. Kumar, C. F. Zhao and P. N. Prasad, J. Clin. Laser Med. Surg., 1997, 15, 201 CAS;
(b) C.-Y. Chen, Y. Tang, Y.-J. Cheng, A. C. Young, J.-W. Ka and A. K.-Y. Jen, J. Am. Chem. Soc., 2007, 129, 7220 CrossRef CAS PubMed;
(c) J. R. Starkey, A. K. Rebane, M. A. Drobizhev, F. Meng, A. Gong, A. Elliott, K. McInnerney and C. W. Spangler, Clin. Cancer Res., 2008, 14, 6564 CrossRef CAS PubMed.
- M. Pawlicki, H. A. Collins, R. G. Denning and H. L. Anderson, Angew. Chem., Int. Ed., 2009, 48, 3244 CrossRef CAS PubMed.
-
(a) M. Drobizhev, Y. Stepanenko, Y. Dzenis, A. Karotki, A. Rebane, P. N. Taylor and H. L. Anderson, J. Am. Chem. Soc., 2004, 126, 15352 CrossRef CAS PubMed;
(b) D. Y. Kim, T. K. Ahn, J. H. Kwon, D. Kim, T. Ikeue, N. Aratani, A. Osuka, M. Shigeiwa and S. Maeda, J. Phys. Chem. A, 2005, 109, 2996 CrossRef CAS PubMed;
(c) K. Ogawa, H. Hasegawa, Y. Inaba, Y. Kobuke, H. Inouye, Y. Kanemitsu, E. Kohno, T. Hirano, S.-I. Ogura and I. Okura, J. Med. Chem., 2006, 49, 2276 CrossRef CAS PubMed;
(d) S. Achelle, P. Couleaud, P. Baldeck, M.-P. Teulade-Fichou and P. Maillard, Eur. J. Org. Chem., 2011, 1271 CrossRef CAS;
(e) J. Schmitt, V. Heitz, A. Sour, F. Bolze, H. Ftouni, J.-F. Nicoud, L. Flamigni and B. Ventura, Angew. Chem., Int. Ed., 2015, 54, 169 CrossRef CAS PubMed.
-
(a) D. Yao, X. Zhang, O. Mongin, F. Paul and C. O. Paul-Roth, Chem. – Eur. J., 2016, 22, 5583 CrossRef CAS PubMed;
(b) D. Yao, X. Zhang, A. Triadon, N. Richy, O. Mongin, M. Blanchard-Desce, F. Paul and C. O. Paul-Roth, Chem. – Eur. J., 2017, 23, 2635 CrossRef CAS PubMed.
- O. Mongin, M. Sankar, M. Charlot, Y. Mir and M. Blanchard-Desce, Tetrahedron Lett., 2013, 54, 6474 CrossRef CAS.
-
(a) M. A. Oar, W. R. Dichtel, J. M. Serin, J. M. J. Frechet, J. E. Roger, J. E. Slagla, P. A. Fleiz, L. S. Tan, T. Y. Ohulchanskyy and P. N. Prasad, Chem. Mater., 2006, 18, 3682 CrossRef CAS;
(b) S.-H. Lee, J.-R. Park, M.-Y. Jeong, H. M. Kim, S. Li, J. Song, S. Ham, S.-J. Jeon and B. R. Cho, ChemPhysChem, 2006, 7, 206 CrossRef CAS PubMed.
-
(a) M. Drobizhev, Y. Stepanenko, Y. Dzenis, A. Karotki, A. Rebane, P. N. Taylor and H. L. Anderson, J. Phys. Chem. B, 2005, 109, 7223 CrossRef CAS PubMed;
(b) T. K. Ahn, K. S. Kim, D. Y. Kim, S. B. Noh, N. Aratani, C. Ikeda, A. Osuka and D. Kim, J. Am. Chem. Soc., 2006, 128, 1700 CrossRef CAS PubMed;
(c) F. Hammerer, S. Achelle, P. Baldeck, P. Maillard and M.-P. Teulade-Fichou, J. Phys. Chem. A, 2011, 115, 6503 CrossRef CAS PubMed;
(d) M. Pawlicki, M. Morisue, N. K. S. Davis, D. G. McLean, J. E. Haley, E. Beuerman, M. Drobizhev, A. Rebane, A. L. Thompson, S. I. Pascu, G. Accorsi, N. Armaroli and H. L. Anderson, Chem. Sci., 2012, 3, 1541 RSC.
- L. B. Josefsen and R. W. Boyle, Theranostics, 2012, 2, 916 CrossRef CAS PubMed.
- O. Mongin, V. Hugues, M. Blanchard-Desce, A. Merhi, S. Drouet, D. Yao and C. Paul-Roth, Chem. Phys. Lett., 2015, 625, 151 CrossRef CAS.
- F. Paul and C. Lapinte, Coord. Chem. Rev., 1998, 178/180, 431 CrossRef.
-
(a) To be published;
(b) M. L. G. Borst, R. E. Bulo, D. J. Gibney, Y. Alem, F. J. J. de Kanter, A. W. Ehlers, M. Schakel, M. Lutz, A. L. Spek and K. Lammertsma, J. Am. Chem. Soc., 2005, 127, 16985 CrossRef CAS PubMed;
(c) B. Sahu, R. Muruganantham and I. N. N. Namboothiri, Eur. J. Org. Chem., 2007, 2477 CrossRef CAS;
(d) D. E. Polyansky, E. O. Danilov, S. V. Voskresensky, M. A. J. Rodgers and D. C. Neckers, J. Am. Chem. Soc., 2005, 127, 13452 CrossRef CAS PubMed;
(e) Z. Fang and B. Liu, Tetrahedron Lett., 2008, 49, 2311 CrossRef CAS;
(f) C. Richardson and C. A. Reed, J. Org. Chem., 2007, 72, 4750 CrossRef CAS PubMed;
(g) A. D. Adler, F. R. Longo, J. D. Finarelli, J. Goldmacher, J. Assour and L. Korsakoff, J. Org. Chem., 1967, 32, 476 CrossRef CAS;
(h) J. S. Lindsey, I. C. Schreiman, H. C. Hsu, P. C. Kearney and A. M. Marguerettaz, J. Org. Chem., 1987, 52, 827 CrossRef CAS.
- N. S. Makarov, M. Drobizhev and A. Rebane, Opt. Express, 2008, 16, 4029 CrossRef CAS PubMed.
-
(a) B. Li, J. Li, Y. Fu and Z. Bo, J. Am. Chem. Soc., 2004, 126, 3430 CrossRef CAS PubMed;
(b) B. Li, K. Xu, M. Sun, Y. Fu, G. Yu, Y. Liu and Z. Bo, Macromolecules, 2006, 39, 456 CrossRef CAS.
-
D. D. Perrin and W. L. F. Armarego, Purification of Laboratory Chemicals, Pergamon Press, Oxford, 3rd edn, 1988 Search PubMed.
- C. Xu and W. W. Webb, J. Opt. Soc. Am. B, 1996, 13, 481 CrossRef CAS.
- M. H. V. Werts, N. Nerambourg, D. Pélégry, Y. Le Grand and M. Blanchard-Desce, Photochem. Photobiol. Sci., 2005, 4, 531 CAS.
Footnote |
† Electronic supplementary information (ESI) available. See DOI: 10.1039/c7nj03522e |
|
This journal is © The Royal Society of Chemistry and the Centre National de la Recherche Scientifique 2018 |