DOI:
10.1039/C7NJ03152A
(Paper)
New J. Chem., 2018,
42, 118-128
Fabrication of lithium ion imprinted hybrid membranes with antifouling performance for selective recovery of lithium
Received
23rd August 2017
, Accepted 15th November 2017
First published on 15th November 2017
Abstract
With the increasing demand for lithium resources, it is of great significance to develop a new technology for the selective recovery of lithium from complex systems. In the present work, antifouling lithium-imprinted hybrid membranes (LIHMs) were fabricated through a hydrolysis polymerization method using a PVDF/GO hybrid membrane as a basement membrane, and polydopamine as an adhesion layer to anchor imprinted sites. The antifouling experiments demonstrated that LIHMs had the underwater oil contact angle of 152 ± 0.8°. Furthermore, the adsorption isotherms, permeation and adsorption kinetics of the LIHMs were researched in detail. The results displayed that LIHMs showed a distinctive adsorption capacity (27.10 mg g−1) and permselectivity (βK/Li = 5.3780, βCa/Li = 21.9402, βMg/Li = 15.5620) for lithium. Also, the regeneration experiments confirmed the good durability of LIHMs, favoring long-term use. Therefore, the LIHMs provided a powerful tool for the effective separation and recovery of lithium in practical applications.
1. Introduction
Lithium ion batteries as efficient energy storage devices have attracted much attention due to their advantages, such as low self-discharge, lack of heavy metal pollution, lack of memory effect, high energy density, long cycle life and environmental friendliness.1–3 As an ideal alternative energy source, lithium ion batteries are widely used in electric vehicles and portable electronics. Nowadays, with the rapid development of electronic products in society, the demand for lithium has become increasingly urgent.4,5 However, the existing lithium resources are becoming sharply depleted as the market grows. Fortunately, saline lake brines provide a large quantity of lithium ions (Li+). Thus, it is significant to research into the selective separation and recovery of Li+ from complex systems.
The traditional separation and recovery processes of Li+, such as ion exchange, calcination leaching and solvent extraction, are usually considered to have high energy demands and to be complicated operations.6–8 Membrane separation technologies with low-energy demands, balanced properties and good applicability have been widely used in many fields such as gas separation, water treatment and drug purification.9,10 Meanwhile, the development of synthetic/artificial membranes with good antifouling properties, stability and selectivity is an important current goal in the recovery of specific metal ions. As is well known, carbon-based nanomaterials are used for the modification of polymeric membranes because of their superior chemical stability and robust mechanical properties. Among them, graphene oxide (GO) has sparked enormous interest as a high-efficiency additive to enhance membrane performance.11,12 For example, Zhang et al. demonstrated that GO could greatly improve the hydrophilicity of a polymer membrane.13 Therefore, GO can be used as an additive to fabricate organic–inorganic hybrid membrane materials, as an effective route to improving their antifouling properties.
Importantly, because of the absence of specific recognition sites in hybrid membranes, issues such as non-selectivity are still limiting their application for the selective separation of metal ions. However, ion imprinted membrane technology, offering specific recognition, is an efficient method for creating chemically complementary specific binding sites for a target ion.14–17 Ion imprinted membrane technology is one of the most promising ways for achieving selective adsorption of metals from mixed solutions. Chen et al. prepared ion imprinted membranes (polyvinyl alcohol/sodium alginate porous composite membranes) using glutaraldehyde as a cross-linking agent for the selective separation of Cr3+.18 Kai et al. prepared a magnetic imprinted chitosan/carbon nanotube nanocomposite for the selective adsorption of Gd3+ from a mixed solution.19 Nevertheless, it is difficult to fabricate Li+ imprinted membranes using common functional monomers because Li+ cannot be combined with the common functional monomers to form a stable framework.
As we know, crown ethers are thought to be important organics in analytical chemistry, organic chemistry and bionics, and they have high selectivity for alkali metal ions.20,21 12-Crown-4-ether (12C4E) has been demonstrated to be capable of coordination with Li+ due to the so called “macrocyclic effect”.22,23 The resultant cavities, of appropriate size after removal of Li+, can easily rebind Li+via coordination. The formation of tailor-made recognition sites on a hybrid membrane surface is a critical factor in obtaining Li+ imprinted membranes with effective separation capacity during a copolymerization procedure. Furthermore, it is well known that the chemical features of imprinted membranes influence the stability and durability of the imprinted layer. In order to address this issue, surface modifications allow control of the surface properties and confer new functionalities to membrane materials. Inspired by the bioadhesion property of marine mussels, polydopamine (pDA) can be easily deposited on almost all organic and inorganic substrates, and has emerged as a versatile secondary reagent for application in protein immobilization, nanoparticle stabilization, cell adhesion and biomineral formation.24,25 Indeed, we have found that a pDA layer coating is a powerful approach for improving chemical stability and providing a versatile surface for the further functionalization of robust and uniform ion imprinted layers.26
In this work, we present new lithium-imprinted hybrid membranes (LIHMs) via surface modification with pDA to anchor imprinted sites. The imprinted sites were synthesized via the binding of Li+–12C4E complexes using triethoxyvinylsilane (VTES) as a functional monomer and tetraethoxysilane (TEOS) as a cross-linker. The as-prepared LIHMs presented superior selective recognition ability for Li+, which mainly resulted from their imprinted sites. Furthermore, the LIHMs exhibited outstanding antifouling performance and regeneration ability. The selective separation mechanism of Li+ is discussed in depth. Importantly, this work has promising prospective applications in the selective separation of Li+ from mixed solutions.
2. Experimental section
2.1 Materials and chemicals
PVDF powder was purchased from Xiamen Tuo Fluoride Materials Co., Ltd. Sulphuric acid (H2SO4), hydrochloric acid (HCl), sodium nitrate (NaNO3), dopamine (98%), tris(hydroxymethyl) aminomethane (Tris–HCl, 99%), N-methyl pyrrolidone (NMP), ammonia solution (28–30%), polyvinyl pyrrolidone (PVP), lithium chloride (LiCl), magnesium chloride (MgCl2), potassium chloride (KCl), calcium chloride (CaCl2) and 12C4E were purchased from Aladdin Reagent Co., Ltd. Graphite, hydrogen peroxide (H2O2), tetraethyl orthosilicate (TEOS, 98%), potassium permanganate (KMnO4), triethoxyvinylsilane (VTES) and ethanol were obtained from Sinopharm Chemical Reagent Co., Ltd. Deionized water (DW) was used in all cleaning processes and aqueous solutions.
2.2 Characterizations
The morphologies of the membranes were observed by scanning electron microscopy (FE-SEM; S-4800II, Hitachi, Japan) and atomic force microscopy (AFM, MFP-3D, America). X-ray photoelectron spectroscopy (XPS, PHI5300, America) and Fourier transform infrared spectroscopy (FT-IR, Nicolet NEXUS-470) were conducted to analyze the chemical compositions of the membrane surfaces. Inductively coupled plasma-atomic emission spectrometry (ICP-AES) was used for detecting the concentrations of ions. Contact angles (CAs) were measured by a contact angle goniometer (KSV CAM 200, Finland). Mechanical tests were carried out on an Instron 3343 mechanical testing machine with a loading rate of 1 mm min−1.
2.3 Preparation of GO/PVDF hybrid membranes
GO was synthesized by the modified Hummers’ method.27,28 The GO/PVDF hybrid membranes were prepared by a facile phase inversion process. Firstly, to obtain casting solutions, NMP (30 g) and GO (for 0 wt%, 5 wt%, 10 wt%, 15 wt% and 20 wt% GO/PVDF) were treated by ultrasonication for about 1 h. Then PVDF was added into the solvent with mechanical stirring for 12 h at 50 °C, and allowed to stand for another 6 h. The casting solution was then cast onto a glass plate using a casting knife and immersed into a coagulant bath (DW) until the membrane detached from the glass plate.
2.4 Modification of GO/PVDF hybrid membranes via pDA
Briefly, a few pieces of GO/PVDF hybrid membrane were put into a 10 mM Tris–HCl (pH = 8.5) aqueous solution with dopamine (2 mg mL−1), and the reaction system was placed under mechanical oscillation at RT for 6 h to form pDA layers on the GO/PVDF hybrid membrane surfaces. After that, the produced pDA@GO/PVDF membranes were rinsed with DW to remove the redundant organic species and dried in a vacuum at 50 °C.
2.5 Synthesis of lithium-imprinted hybrid membranes (LIHMs)
Firstly, LiCl (0.02 mmol) and 12C4E (0.02 mmol) were dissolved in 30 mL ethanol and stirred for 5 min. Then VTES (0.2 mL), TEOS (0.5 mL) and a piece of pDA@GO/PVDF membrane were added to the solution with continuous stirring for 5 min. Then a mixture of ammonia solution (0.5 mL) and DW (5 mL) was added into the above-mentioned solution, and the polymerization was carried out under stirring for 16 h at 30 °C. After that, the resultant membranes were rinsed with methanol and DW to remove impurities. Finally, the target ion (Li+) was removed by rinsing with HCl (0.5 mol L−1) until Li+ could no longer be detected by ICP-AES. LIHMs were obtained by repeated washing with DW and were dried in a vacuum at 50 °C.
The non-imprinted hybrid membranes (NIHMs) were prepared using the same procedure as used for LIHMs except that LiCl was not added.
2.6 Adsorption experiments
The effect of pH on adsorption was tested at different pH values ranging from 1.0 to 9.0. The pH value of Li+ aqueous solution (50 mg L−1) was adjusted by 0.1 M HCl or 0.1 M NH4OH. The adsorption process was continued for 5 h and then the solutions were tested by ICP-AES.
Adsorption isotherms and adsorption kinetics of LIHMs and NIHMs were investigated by batch experiments. In adsorption isotherm experiments, to explore the adsorption capacity of Li+, a certain quantity of LIHMs or NIHMs was put into LiCl solutions of different concentrations (20, 50, 70, 100, 120, 150 and 200 mg L−1). The solutions were kept for 5 h at 25 °C and then tested by ICP-AES. The adsorption capacity Qe (mg g−1) was calculated by the following equation:
| 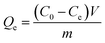 | (1) |
where
C0 and
Ce (mg L
−1) are the initial and equilibrium concentrations of Li
+, respectively.
V (mL) is volume of the solution, and
m (mg) is the mass of LIHMs or NIHMs.
The adsorption kinetics were investigated as follows: a certain quantity of LIHMs or NIHMs was put into LiCl solution (100 mg L−1) at 25 °C for predetermined time intervals (5, 10, 15, 30, 60, 120, 180 min). The residual concentration of Li+ was tested by ICP-AES and the amount of Li+ adsorbed at time t, Qt (mg g−1), was calculated as follows:
| 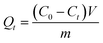 | (2) |
where
Ct is the concentration of Li
+ at different times.
The selectivity factor α could be calculated as follows:
|  | (3) |
where
QI and
QN are the amounts of Li
+ adsorbed by LIHMs and NIHMs, respectively.
2.7 Selective permeability experiments
The selectivity and permeability are critical for recognition of Li+. The permeation experiments were conducted using a U-shaped device. The LIHMs were fixed in the middle of the two tubular compartments with an inner diameter of 25 mm. The feeding solution containing Li+, K+, Ca2+ and Mg2+ (each at 50 mg L−1) was put into one chamber, and the same volume of DW (receiving solution) was put into the other chamber. The system was kept at 25 °C under conditions of homogeneous oscillation by a mechanical oscillator, and the receiving solution was measured at different times (5, 10, 15, 30, 60, 120, 180, 240, 360 min) by ICP-AES. The permeation flux, permeability coefficient and permselectivity factor are represented using J (mg cm−2 h−1), P (cm2 s−1) and β, respectively. The equations used for calculation are as follows: |  | (4) |
|  | (5) |
|  | (6) |
where V (mL) and A (cm2) are the volume of receiving solution and effective membrane area, respectively; ΔCi/Δt is the change of concentration in the receiving solution; d (cm) is the effective membrane thickness; and (CFi − CRi) (mg L−1) is the concentration difference between the feeding and receiving solutions.
3. Results and discussion
3.1 Preparation of LIHMs
The preparation of LIHMs is illustrated in Fig. 1. Firstly, the PVDF/GO hybrid membranes were prepared by a facile phase inversion method using NMP as the solvent and DW as the coagulant, which was the main cause of the formation of porous membranes. PVDF and GO could interact by hydrogen bonding to improve the mechanical properties of the membranes, and the existence of oxygen-containing functional groups on GO endowed the membranes with hydrophilicity, enhancing their antifouling performance. Secondly, inspired by the bioadhesion of mussels, pDA was adopted as the anchor for loading imprinted sites due to the nature of its surface functional groups such as catechol, amine, and imine.29–31 Lastly, the imprinting procedure was performed by hydrolysis polymerization methodology using 12C4E as a selective metal complexing agent for Li+, VTES as the functional monomer, and TEOS as the cross-linker. 12C4E was immobilized on the membrane surface by hydrogen bonding with the functional monomer, and π–π interactions with pDA. TEOS formed a network to reinforce the imprinted sites for effective rebinding of target ions (Li+). The resultant cavities, with appropriate size after the removal of Li+ ions, were capable of selective adsorption and separation of Li+.
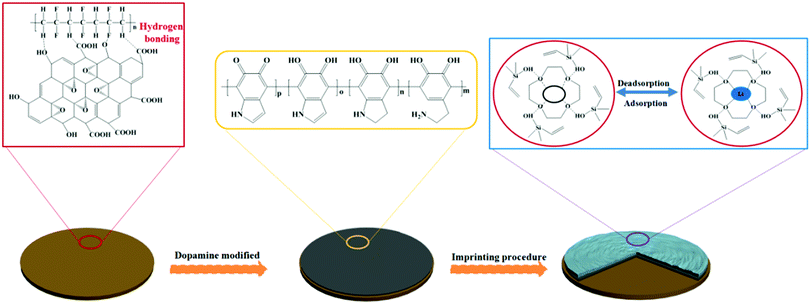 |
| Fig. 1 Schematic illustration of the procedure for the preparation of LIHMs for the selective adsorption of Li+. | |
3.2 Optimization of basement membrane
In order to obtain the optimized imprinting performance of the PVDF/GO hybrid membrane, the wettability of GO/PVDF (0 wt%, 5 wt%, 10 wt%, 15 wt%, 20 wt%) hybrid membranes were tested, as shown in Fig. 2. The water contact angles of 0 wt% and 5 wt% PVDF/GO were 103 ± 0.8° and 93 ± 0.8°, respectively. The water contact angles of 10 wt%, 15 wt%, and 20 wt% GO/PVDF hybrid membranes were about 80° (Fig. 2, upper panel). The underwater oil contact angles of 0 wt% and 5 wt% PVDF/GO hybrid membranes were 103 ± 0.8° and 130 ± 0.8°, respectively. The underwater oil contact angles of 10 wt%, 15 wt% and 20 wt% PVDF/GO hybrid membranes were all greater than 150° (Fig. 2, lower panel). It was observed that the hydrophilicity of the hybrid membranes increased with increasing content of graphene oxide and then tended toward stability. Therefore, the 10 wt% PVDF/GO hybrid membrane was used as the basement membrane for fabricating the antifouling LIHMs.
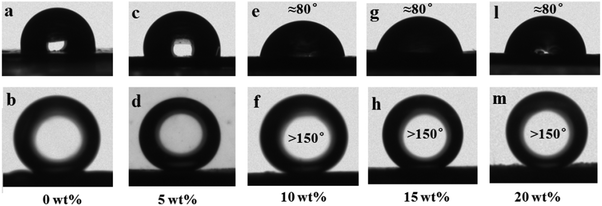 |
| Fig. 2 Wettability of GO/PVDF (0 wt%, 5 wt%, 10 wt%, 15 wt%, 20 wt%) hybrid membranes. | |
3.3 Characterization of membranes
The SEM images of various membranes are shown in Fig. 3. The PVDF/GO hybrid membrane (Fig. 3a and b) exhibited a porous structure with pore diameters of several micrometers. After modification by dopamine, the pDA@PVDF/GO membrane surface formed a hierarchical structure. A pDA layer was clearly observed on the pristine hybrid membrane surface (Fig. 3c and d). Fig. 3e, f show that LIHMs had smaller pore sizes in comparison to the pDA@PVDF/GO membrane, indicating the deposition of the polymer network on the pDA@PVDF/GO membrane surface. Furthermore, three dimensional AFM images of the as-prepared membranes are presented in Fig. 4. Fig. 4a and b show that the PVDF/GO hybrid membrane had a rough and porous structure with a root mean square (RMS) roughness of 547. Compared with the PVDF/GO hybrid membrane, the pDA@PVDF/GO membrane (Fig. 4c and d) was obviously smoother, and its RMS had declined to 339, confirming the presence of the pDA layer on the membrane surface. Additionally, from three dimensional AFM images (Fig. 4e and f), the roughness of the LIHMs was obviously decreased and the RMS was 214, indicating the presence of the polymer layer on the hybrid membrane.
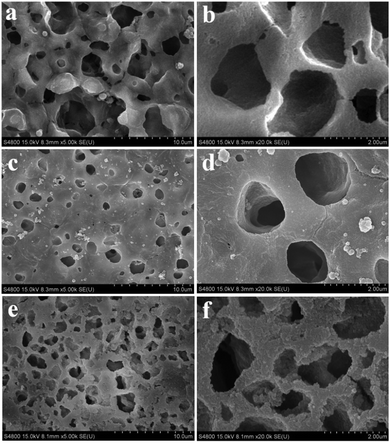 |
| Fig. 3 SEM images of GO/PVDF (a and b), pDA@GO/PVDF (c and d) and LIHMs (e and f). | |
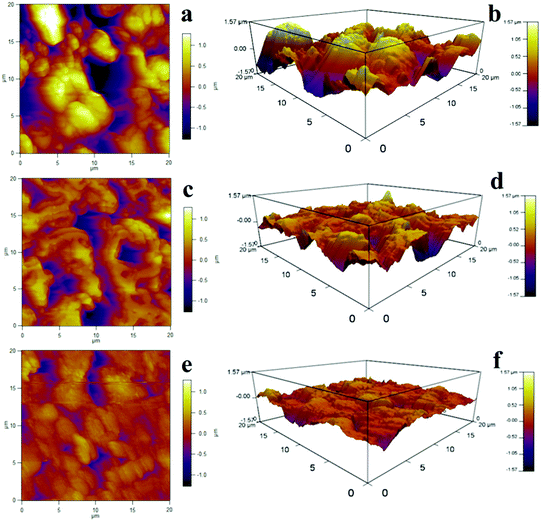 |
| Fig. 4 AFM images of GO/PVDF (a and b), pDA@GO/PVDF (c and d) and LIHMs (e and f). | |
The GO/PVDF and pDA@GO/PVDF hybrid membranes and LIHMs were characterized by FT-IR spectroscopy to determine their surface chemistry, as shown in Fig. 5. The strong characteristic peaks at 1180 cm−1, 880 cm−1 and 1403 cm−1 were ascribed to –CF2 stretching, C–C vibration, and CH2 in-plane bending, respectively, and originated from PVDF. The bands at 1668 cm−1 and 1073 cm−1 were attributed to C
C in the aromatic ring and C–O–C in the epoxy group of GO.32 These results indicated that GO/PVDF hybrid membranes had been successfully prepared. The spectrum of pDA@PVDF/GO showed a red shift due to the π–π interactions. The new peak at 1508 cm−1 was assigned to N–H bending vibrations, and the enhanced peak at 1654 cm−1 (C
C) indicated that pDA was coated on the GO/PVDF hybrid membrane. For the LIHMs, the characteristic absorption bands at 1734 cm−1, 1173 cm−1, 1276 cm−1, 3016 cm−1 and 1501 cm−1 were ascribed to C
O stretching, C–O stretching, C–O bending, C–H stretching and CH2 bending of 12C4E, and the band at 1073 cm−1 was due to Si–O–Si stretching vibrations of VTES and TEOS.21 These results demonstrated that LIHMs had been successfully prepared.
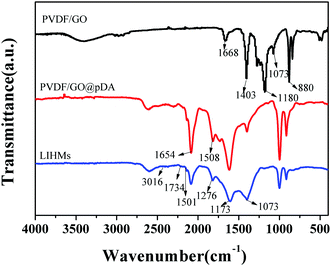 |
| Fig. 5 FT-IR spectra of PVDF/GO, pDA@PVDF/GO and LIHMs. | |
The surface compositions of the as-prepared membranes were further analyzed via XPS. From Fig. 6a, it can be seen that C, F and O elements were detected in the XPS survey spectrum of the PVDF/GO hybrid membrane. Moreover, the C1s spectrum of the PVDF/GO membrane is shown in Fig. 6b. Five different peaks at 284.8 eV, 287.7 eV, 286.4 eV, 288.3 eV and 290.7 eV were observed, corresponding to C–C, O–C–O, C–OH, C
O, and CF2, respectively.32 The results demonstrated that the PVDF/GO hybrid membrane had been synthesized. Additionally, a new N1s peak emerged in the XPS survey spectrum of the pDA@PVDF/GO membrane (Fig. 6b) and the N1s spectrum is shown in Fig. 6c. Three peaks at 395.7 eV, 398.6 eV, and 401.7 eV were ascribed to C–N, C–NH–C and –NH2 of pDA, respectively. These results indicated that pDA had been successfully coated on the PVDF/GO hybrid membrane surface. After imprinting, Si 1s and Si 2p peaks were clearly observed in the survey spectrum of LIHMs (Fig. 6a). Moreover, the Si 2p spectrum of LIHMs was deconvoluted into two peaks at 100.6 eV Si–C and 103.7 eV Si–O, which suggested the formation of an imprinted polymer layer on the pDA@PVDF/GO membrane surface.
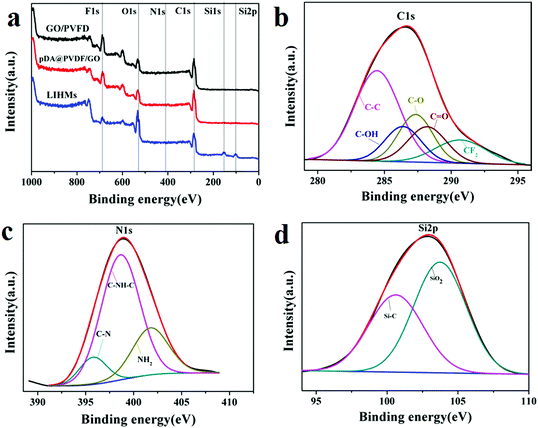 |
| Fig. 6 XPS spectra of the as-prepared membranes: wide survey scans (a), C1s spectrum of PVDF/GO (b), N1s spectrum of pDA@PVDF/GO (c) and Si 2p spectrum of LIHMs (d). | |
3.4 Effects of pH on adsorption
The pH of the aqueous solution was one of the most critical parameters for adsorption capacity. Thus, the effects of pH on the adsorption capacity of LIHMs and NIHMs were investigated. As shown in Fig. 7, it was observed that the adsorption capacity of the membranes increased remarkably with increasing pH values. The adsorption capacity reached a maximum at pH = 6. When the pH values were more than 6, the adsorption capacity was slightly decreased. The results might be due to the protonation of the oxygen groups of 12C4E within polymers at low pH values (pH < 3), which could diminish the number of available binding sites for Li+. Additionally, the protonation of active sites on the adsorbents would induce electrostatic repulsion toward Li+. At higher pH values (pH > 3), the deprotonated adsorbents would favor the accommodation of Li+ in the artificial receptor sites. Therefore, the optimal pH = 6 was used for further studies of the membrane adsorbents.
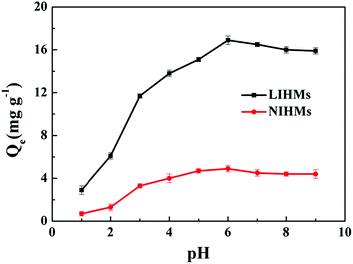 |
| Fig. 7 Effect of pH on adsorption capacity of LIHMs and NIHMs. | |
3.5 Adsorption isotherms
The adsorption isotherm experiments were conducted to evaluate the adsorption performance of LIHMs and NIHMs for Li+. As shown in Fig. 8, the equilibrium adsorption capacity of LIHMs and NIHMs for Li+ increased with increasing initial Li+ concentration from 20 to 200 mg L−1. It was observed that the adsorption capacity of LIHMs was much higher than that of NIHMs, indicating that LIHMs had specific recognition ability toward Li+, originating from the imprinting effect. Additionally, Langmuir and Freundlich isotherm models were used to study the adsorption behavior of LIHMs and NIHMs in detail, and the parameters are summarized in Table 1. The linear correlation coefficients showed that the experimental data were better fitted by the Langmuir isotherm model33 (R2 > 0.99) than by the Freundlich model (R2 < 0.99).34 The results indicated that Li+ led to monolayer adsorption. Furthermore, the Qm value of LIHMs is 27.10 mg g−1, while the Qm of NIHMs is 7.56 mg g−1,35 which showed that the adsorption capacity of LIHMs was much greater than that of NIHMs. Additionally, the selectivity factor α of LIHMs is 3.5, confirming that our LIHMs provided an outstanding performance for the selective adsorption of Li+. Moreover, as shown in Table 2, the adsorption capacity of LIHMs was higher than those reported in the literature.8,36–38
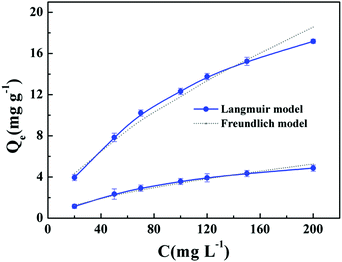 |
| Fig. 8 The nonlinear fitting of the Langmuir model and Freundlich model. | |
Table 1 Langmuir and Freundlich parameters for adsorption of Li+ on the LIHMs and NIHMs
Adsorbents |
Q
e,exp (mg g−1) |
Langmuir |
Freundlich |
K
L (L mg−1) |
Q
m (mg g−1) |
R
2
|
K
F (mg g−1) (L mg−1)1/n |
1/n |
R
2
|
LIHMs |
17.18 |
0.0090 |
27.10 |
0.9910 |
0.6936 |
0.6249 |
0.9899 |
NIHMs |
4.86 |
0.0091 |
7.56 |
0.9979 |
0.1980 |
0.6200 |
0.9879 |
Table 2 Comparison of LIHMs with other adsorbents applied for the adsorption of Li+
Adsorbents |
Adsorption capacity (mg g−1) |
Ref. |
Fe3O4@SiO2-IIP |
4.1 |
8
|
Mn2O3 |
1.1 |
36
|
(PAN)-MnO2 |
19.67 |
37
|
IIPs |
8.02 |
38
|
LIHMs |
27.10 |
This study |
3.6 Adsorption kinetics
The adsorption kinetics experiments were carried out to evaluate the rate-controlling mechanism. As shown in Table 3, the adsorption kinetics data fitted much better to the pseudo-second-order model (R2 > 0.99) than to the pseudo-first-order model (R2 < 0.94), indicating that the adsorption process between Li+ and LIHMs was mainly chemical adsorption.39,40Fig. 9 shows the nonlinear fitting of the pseudo-second-order model to the LIHM and NIHM adsorption data. The adsorption rate of LIHMs was fast in the first 80 min and then it tended to equilibrium as a result of the large numbers of effective recognition sites on the surface of LIHMs. The adsorption rate of NIHMs was slower than that of LIHMs because NIHMs did not possess recognition sites.
Table 3 Fitting parameters of the pseudo-first-order and pseudo-second-order models to the experimental data
Adsorbents |
Pseudo-first-order model |
Pseudo-second-order model |
Q
e,exp (mg g−1) |
Q
e,cal (mg g−1) |
K
1 (min−1) |
R
2
|
Q
e,cal (mg g−1) |
K
2 (g mg−1 min−1) |
R
2
|
LIHMs |
10.45 |
6.05 |
0.009 |
0.930 |
11.11 |
0.0913 |
0.996 |
NIHMs |
2.41 |
2.49 |
0.004 |
0.928 |
2.56 |
0.0556 |
0.992 |
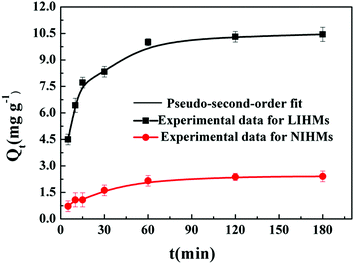 |
| Fig. 9 The nonlinear fitting of the pseudo-second-order model. | |
3.7 Selective permeation experiments
In order to evaluate the permselectivity of LIHMs, experiments were conducted using a mixed solution of Li+, K+, Mg2+ and Ca2+ each at a concentration of 50 mg L−1. As shown in Fig. 10a, the concentration of Li+ passing through the LIHMs was lower than those of other competing ions (K+, Mg2+, Ca2+), indicating a slower diffusion rate. Fig. 10b shows that Li+, K+, Mg2+ and Ca2+ could freely permeate the NIHMs due to the concentration gradients. These results showed that the LIHMs are a good candidate for practical application in the continuous separation of Li+.
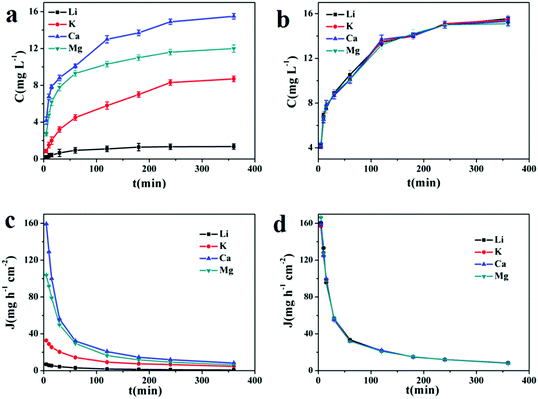 |
| Fig. 10 Permeation performances of LIHMs (a) and NIHMs (b); time-dependent permeation flux curves of LIHMs (c) and NIHMs (d). | |
The time-dependent permeation flux curves of LIHMs and NIHMs are presented in Fig. 10c and d. It can be seen that permeation flux declined with increasing time, which might be caused by the decreased concentration gradient or ion adsorption. Furthermore, from Fig. 10c, we can see that the permeation flux of Li+ across LIHMs was lower than that of the other coexisting ions (K+, Mg2+, Ca2+) during the initial 150 min. In contrast, Fig. 10d shows that there was no difference between the permeation flux of Li+ and the other coexisting ions across NIHMs. Additionally, as shown in Table 4, the β values of LIHMs for Li+ relative to K+, Ca2+ and Mg2+ are 5.3780, 21.9402 and 15.5620, respectively, while the NIHM β values are nearly 1. Clearly, this result revealed that the LIHMs could selectively separate Li+ due to the imprinted sites on the surface of the LIHMs.41,42
Table 4 Time-dependent permeation results across LIHMs and NIHMs for Li, K, Ca and Mg
Membranes |
Ions |
J (mg cm−2 h−1) |
P (cm2 h−1) |
β
mixture/Li
|
LIHMs |
Li |
0.0070 |
0.0068 |
— |
K |
0.2537 |
0.3669 |
5.3780 |
Ca |
7.5337 |
1.4967 |
21.9403 |
Mg |
1.7211 |
1.0616 |
15.5620 |
|
NIHMs |
Li |
6.7395 |
1.5156 |
— |
K |
6.9589 |
1.4905 |
0.9834 |
Ca |
7.0117 |
1.4890 |
0.9824 |
Mg |
6.8516 |
1.5110 |
0.9970 |
3.8 Proposed selective separation mechanism
Permeation mechanisms are generally divided into retarded permeation and facilitated permeation, according to different transfer modes.43,44 Based on the above results, a selective separation mechanism is proposed for Li+ using LIHMs. As shown in Fig. 11, the imprinted sites within LIHMs can rebind Li+ through coordination with 12C4E, which hinders the permeation of Li+. The other competing ions can freely diffuse to the other side of the LIHMs, due to the gradients in ion concentration. Additionally, the different transfer rates of the competing ions may be attributed to the different strengths of binding interactions. The results suggested that the LIHMs could selectively separate Li+ even with the coexistence of other metal ions. Therefore, the transport mechanism for permeation of the ions across LIHMs is in accordance with the retarded permeation mechanism.
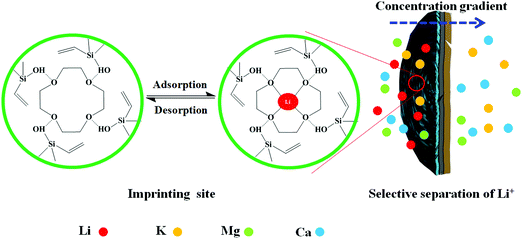 |
| Fig. 11 Schematic representations of the selective separation mechanism of LIHMs. | |
3.9 Regeneration
The regeneration experiments were conducted to evaluate the reusability and durability of the LIHMs by an adsorption–desorption procedure. The saturated LIHMs were washed using 1 M HCl solution to desorb Li+ from the complementary cavities. Fig. 12 shows that the rebinding capacity of Li+ did not significantly weaken after six cycles. Moreover, the adsorption capacity of LIHMs for Li+ after the sixth adsorption–desorption cycle was decreased by just 8.2%, which indicated that the imprinted sites within LIHMs were stable enough for long-term application.
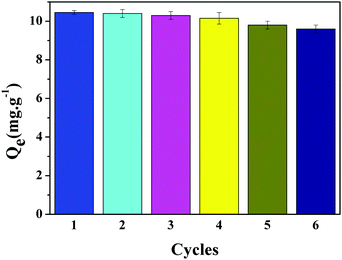 |
| Fig. 12 Adsorption stability and regeneration performances of LIHMs. | |
3.10 Antifouling experiments
Generally, the antifouling property of a membrane is evaluated by surface wettability. Fig. 13a shows that the water contact angle of PVDF is about 103 ± 0.8°, and that of the PVDF/GO hybrid membrane is about 80 ± 0.8° (Fig. 13b) which can be attributed to the modification of the PVDF with hydrophilic GO. The contact angle of LIHMs is about 82 ± 0.8° (Fig. 13c), which indicated that LIHMs still retain favorable hydrophilicity. The underwater oil contact angle of various membranes were also measured using 1,2-dichloroethane (about 5 μL). Pristine PVDF membrane has a contact angle of about 132 ± 0.8° (Fig. 13d), while the PVDF/GO hybrid membrane measures about 154 ± 0.8° (Fig. 13e) showing underwater superoleophobicity. The LIHMs also possess a high underwater oil contact angle of 152 ± 0.8 (Fig. 13f) displaying their outstanding antifouling property. The results indicated that the surface of LIHMs formed a water layer, which is beneficial for resisting organics.
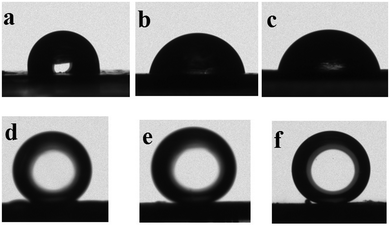 |
| Fig. 13 Water contact angle of PVDF (a), PVDF/GO (b) and LIHMs (c), underwater oil contact angle of PVDF (d), PVDF/GO (e) and LIHMs (f). | |
3.11 Mechanical strength of LIHMs
The mechanical strength is a vital factor for evaluating the usability of membranes in separation process. The typical stress–strain curve of LIHMs is shown in Fig. 14a. The tensile strength and Young's modulus of LIHMs were determined to be 0.286 MPa and 5.151 MPa, respectively. The test results indicated that the LIHMs have a good mechanical strength, which is sufficient for a typical microfiltration or ultrafiltration operation. Fig. 14b displays that a piece of LIHMs (about 20 mm × 15 mm) can hold a 100 g weight, which further demonstrated the good mechanical strength of LIHMs.
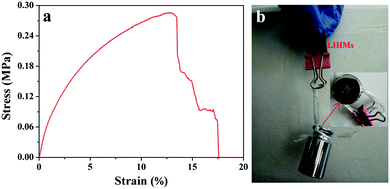 |
| Fig. 14 (a) The stress–strain curves of LIHMs, (b) the picture of LIHMs holding a 100 g weight. | |
4. Conclusions
In this study, a new type of LIHM has been successfully developed for the selective separation and recovery of Li+. The LIHMs presented superior antifouling with an underwater oil contact angle of 152 ± 0.8°. The regeneration experiments with LIHMs exhibited their excellent rebinding capacity for Li+ after six times reuse, indicating that LIHMs have favorable reusability and durability. The adsorption isotherm experiments, permeation and separation experiments confirmed that the LIHMs have outstanding adsorption capacity (Qm = 27.10 mg g−1) and permselectivity (βK/Li = 5.3780, βCa/Li = 21.9402, βMg/Li = 15.5620) for Li+, which can mainly be attributed to the effect of their specific imprinted sites. In summary, the LIHMs not only display selective separation performance but also show superior regeneration and antifouling resistance. Thus, these new LIHMs show promising potential applications for the specific and selective separation of Li+ from mixed solutions.
Conflicts of interest
There are no conflicts to declare.
Acknowledgements
This work was financially supported by the National Natural Science Foundation of China (No. U1507118, U1407123, 21406085), the Natural Science Foundation of Jiangsu Province (No. BK20151350, BK20140580, BK20161367), the China Postdoctoral Science Foundation (Project No. 2014M561588, 1501067C), and the fifteenth batch of undergraduate research projects of Jiangsu University (No. Y15A162).
References
- M. Pan and W. Lai, Cutting copper fiber/paraffin composite phase change material discharging experimental study based on heat dissipation capability of Li-ion battery, J. Renewable Energy, 2017, 114, 408–422 CrossRef CAS.
- Y. Y. Huang, Brief history of the lithium battery, J. Phys., 2007, 36, 643–651 CAS.
- S. Wang, Y. Li, Y. Z. Li, Y. Mao, Y. Zhang, W. Guo and M. Zhong, A forced gas cooling circle packaging with liquid cooling plate for the thermal management of Li-ion batteries under space environment, Appl. Therm. Eng., 2017, 929–939 CAS.
- D. Sun, Y. Zhu, M. Meng, Y. Qiao, Y. Yan and C. Li, Fabrication of highly selective ion imprinted macroporous membranes with crown ether for targeted separation of lithium ion, Sep. Purif. Technol., 2017, 175, 19–26 CrossRef CAS.
- Y. P. Deng, Z. W. Yin, Z. G. Wu, S. J. Zhang, F. Fu, T. Zhang, J. T. Li, L. Huang and S. G. Sun, Layered/spinel heterostructured and hierarchical micro/nanostructured Li-rich cathode materials with enhanced electrochemical properties for Li-ion batteries, ACS Appl. Mater. Interfaces, 2017, 9, 21065–21070 CAS.
- Q. Yu and K. Sasaki, Microwave-assisted hydrothermal synthesis of nanocrystalline lithium-ion sieve from biogenic manganese oxide, its characterization and lithium sorption studies, Hydrometallurgy, 2016, 165, 118–124 CrossRef CAS.
- A. Mizutani, K. Sugiura, H. Ohta and K. Koumoto, Exchange, I. Epitaxial film growth of LixCoO2 via topotactic ion exchange of Na0.8CoO2, Cryst. Growth Des., 2008, 8, 755–758 CAS.
- X. Luo, B. Guo, J. Luo, F. Deng, S. Zhang, S. Luo and J. Crittenden, Recovery of lithium from wastewater using development of Li ion-imprinted polymers, ACS Sustainable Chem. Eng., 2015, 3, 460–467 CrossRef CAS.
- Y. Wu, M. Yan, X. Liu, P. Lv, J. Cui, M. Meng, J. Dai, Y. Yan and C. Li, Accelerating the design of multi-component nanocomposite imprinted membrane by integrating a versatile metal-organic methodology with mussel-inspired secondary reaction platform, Green Chem., 2015, 17, 3338–3349 RSC.
- F. H. Akhtar, M. Kumar and K. V. Peinemann, Graphene oxide composite membranes for improved water vapor separation, J. Membr. Sci., 2017, 525, 187–194 CrossRef CAS.
- M. J. Allen, V. C. Tung and R. B. Kaner, Honeycomb carbon: A review of graphene, Chem. Rev., 2010, 110, 132–145 CrossRef CAS PubMed.
- N. F. D. Aba, J. Y. Chong, B. Wang, C. Mattevi and K. Li, Graphene oxide membranes on ceramic hollow fibers-microstructural stability and nanofiltration performance, J. Membr. Sci., 2015, 484, 87–94 CrossRef CAS.
- J. Zhang, X. Pan, Q. Xue, D. He, L. Zhu and Q. Guo, Antifouling hydrolyzed polyacrylonitrile/graphene oxide membrane with spindle-knotted structure for highly effective separation of oil–water emulsion, J. Membr. Sci., 2017, 532, 38–46 CrossRef CAS.
- M. Meng, Z. He, L. Yan, Y. Yan, F. Sun, Y. Liu and S. Liu, Fabrication of a novel cellulose acetate imprinted membrane assisted with chitosan-wrapped multi-walled carbon nanotubes for selective separation of salicylic acid from industrial wastewater, J. Appl. Polym. Sci., 2015, 132, 42654 CrossRef.
- A. Xie, J. Dai, X. Chen, T. Zou, J. He, Z. Chang, C. Li and Y. Yan, Hollow imprinted polymer nanorods with a tunable shell using halloysite nanotubes as a sacrificial template for selective recognition and separation of chloramphenicol, RSC Adv., 2016, 6, 51014–51023 RSC.
- Y. Wu, M. Yan, J. Cui, Y. Yan and C. Li, A multiple-functional Ag/SiO2/organic based biomimetic nanocomposite membrane for high-stability protein recognition and
cell adhesion/detachment, Adv. Funct. Mater., 2015, 25, 5823–5832 CrossRef CAS.
- J. Cui, Y. Wu, M. Meng, J. Lu, C. Wang, J. Zhao and Y. Yan, Bio-inspired synthesis of molecularly imprinted nanocomposite membrane for selective recognition and separation of artemisinin, J. Appl. Polym. Sci., 2016, 133, 43405 CrossRef.
- J. H. Chen, G. P. Li, Q. L. Liu, J. C. Ni, W. B. Wu and J. M. Lin, Cr(III) ionic imprinted polyvinyl alcohol/sodium alginate (PVA/SA) porous composite membranes for selective adsorption of Cr(III) ions, Chem. Eng. J., 2010, 165, 465–473 CrossRef CAS.
- L. Kai, G. Qiang, G. Yadavalli, S. Xiang, H. Lei, H. Bo, K. Xia and C. Zhou, Selective adsorption of Gd3+ on a magnetically retrievable imprinted chitosan/carbon nanotube composite with high capacity, ACS Appl. Mater. Interfaces, 2015, 7, 21047–21055 Search PubMed.
- G. M. Murray, A. L. Jenkins, A. Bzhelyansky and O. M. Uy, Molecularly imprinted polymers for the selective sequestering and sensing of ions, Johns Hopkins APL Tech. Dig., 1997, 464, 464–472 Search PubMed.
- B. Hashemi, M. Shamsipur and Z. Seyedzadeh, Synthesis of ion imprinted polymeric nanoparticles for selective pre-concentration and recognition of lithium ions, New J. Chem., 2016, 40, 4803–4809 RSC.
- D. K. Cabbiness and D. W. Margerum, Macrocyclic effect on the stability of copper(II) tetramine complexes, J. Am. Chem. Soc., 1969, 91, 6540–6541 CrossRef CAS.
- H. K. Frensdorff, Stability constants of cyclic polyether complexes with univalent cations, J. Am. Chem. Soc., 1971, 93, 600–606 CrossRef CAS.
- J. H. Waite and X. Qin, Polyphosphoprotein from the adhesive pads of mytilus edulis, J. Biochem., 2001, 40, 2887–2893 CrossRef CAS.
- J. H. Waite and M. L. Tanzer, Polyphenolic substance of mytilus edulis: novel adhesive containing L-dopa and hydroxyproline, Science, 1981, 212, 1038–1040 CAS.
- Y. Liu, K. Ai and L. Lu, Polydopamine and its derivative materials: synthesis and promising applications in energy, environmental, and biomedical fields, Chem. Rev., 2014, 114, 5057–5115 CrossRef CAS PubMed.
- C. Chen, W. Cai, M. Long, B. Zhou, Y. Wu, D. Wu and Y. Feng, Synthesis of visible-light responsive graphene oxide/TiO2 composites with p/n heterojunction, ACS Nano, 2014, 4, 6425–6432 CrossRef PubMed.
- W. S. Hummers and R. E. Offeman, Preparation of graphitic oxide, J. Am. Chem. Soc., 1958, 80, 1339 CrossRef CAS.
- C. Cheng, S. Li, W. Zhao, Q. Wei, S. Nie, S. Sun and C. Zhao, The hydrodynamic permeability and surface property of polyethersulfone ultrafiltration membranes with mussel-inspired polydopamine coatings, J. Membr. Sci., 2012, s417–s418, 228–236 CrossRef.
- C. Cheng, S. Li, J. Zhao, X. Li, Z. Liu, L. Ma, X. Zhang, S. Sun and C. Zhao, Biomimetic assembly of polydopamine-layer on graphene: mechanisms, versatile 2D and 3D architectures and pollutant disposal, Chem. Eng. J., 2013, 228, 468–481 CrossRef CAS.
- Y. He, J. Wang, H. Zhang, T. Zhang, B. Zhang, S. Cao and J. Liu, Polydopamine-modified graphene oxide nanocomposite membrane for proton exchange membrane fuel cell under anhydrous conditions, J. Mater. Chem. A, 2014, 2, 9548–9558 CAS.
- H. L. Ma, H. B. Zhang, Q. H. Hu, W. J. Li, Z. G. Jiang, Z. Z. Yu and A. Dasari, Functionalization and reduction of graphene oxide with p-phenylene diamine for electrically conductive and thermally stable polystyrene composites, ACS Appl. Mater. Interfaces, 2012, 4, 1948–1953 CAS.
- I. Langmuir, The adsorption of gases on plane surfaces of glass, mica and platinum, J. Chem. Phys., 1918, 40, 1361–1403 CAS.
- H. M. F. Freundlich, Uber die adsorption losungen, Z. Phys. Chem., 1906, 57, 385–470 CAS.
- A. Xie, J. Dai, X. Chen, P. Ma, J. He, C. Li, Z. Zhou and Y. Yan, Ultrahigh adsorption of typical antibiotics onto novel hierarchical porous carbons derived from renewable lignin via halloysite nanotubes-template and in situ activation, Chem. Eng. J., 2016, 304, 609–620 CrossRef CAS.
- Y. Han, H. Kim and J. Park, Millimeter-sized spherical ion-sieve foams with hierarchical pore structure for recovery of lithium from seawater, Chem. Eng. J., 2012, 210, 482–489 CrossRef CAS.
- J. L. Xiao, S. Y. Sun, X. Song, P. Li and J. G. Yu, Lithium ion recovery from brine using granulated polyacrylamide–MnO 2 ion-sieve, Chem. Eng. J., 2015, 279, 659–666 CrossRef CAS.
- X. Luo, W. Zhong, J. Luo, L. Yang, J. Long, B. Guo and S. Luo, Lithium ion-imprinted polymers with hydrophilic PHEMA polymer brushes: The role of grafting density in anti-interference and anti-blockage in wastewater, J. Colloid Interface Sci., 2016, 492, 146 CrossRef PubMed.
- A. Xie, J. D. Dai, J. Y. Cui, J. H. Lang, M. B. Wei, X. H. Dai, C. X. Li and Y. S. Yan, Novel graphene oxide-confined nanospace directed synthesis of glucose-based porous carbon nanosheets with enhanced adsorption performance, ACS Sustainable Chem. Eng., 2017 DOI:10.1021/acssuschemeng.7b02917.
- F. C. Wu, R. L. Tseng, S. C. Huang and R. S. Juang, Characteristics of pseudo-second-order kinetic model for liquid-phase adsorption: a mini-review, Chem. Eng. J., 2009, 151, 1–9 CrossRef CAS.
- M. Ulbricht, Advanced functional polymer membranes, Polymer, 2006, 47, 2217–2262 CrossRef CAS.
- C. Zhao, B. Yu, B. Qian, W. Qiang, K. Yang and A. Zhang, BPA transfer rate increase using molecular imprinted polyethersulfone hollow fiber membrane, J. Membr. Sci., 2008, 310, 38–43 CrossRef CAS.
- D. K. Roper and E. N. Lightfoot, Separation of Biomolecules Using Adsorptive Membranes, J. Chromatogr. A, 1995, 702, 3–26 CrossRef CAS.
- R. D. Noble, Generalized microscopic mechanism of facilitated transport in fixed site carrier membranes, J. Membr. Sci., 1992, 75, 121–129 CrossRef CAS.
|
This journal is © The Royal Society of Chemistry and the Centre National de la Recherche Scientifique 2018 |