DOI:
10.1039/C7NH00147A
(Communication)
Nanoscale Horiz., 2018,
3, 35-44
Sliced graphene foam films for dual-functional wearable strain sensors and switches†
Received
14th September 2017
, Accepted 30th October 2017
First published on 30th October 2017
Abstract
The demand for wearable sensors is growing in many emerging fields, such as health monitoring, human-machine interfaces, robotics and personalized medicine. Here, we report the integration of skin-mountable, flexible, stretchable, dual-functional sensors and switches together with silicon-based electronics to create a novel healthcare system. We employ a facile approach to design highly stretchable graphene foam (GF)/PDMS composite films with tunable sensitivities and switching capabilities by simply controlling the thickness of GF. The GF/PDMS composite films deliver a high gauge factor of 24 at a 10% strain, tunable stretchability up to 70% and an excellent on/off switching ratio on the order of 1000. The highly reversible switching capability of the composite films is realized by identifying abnormal resistance changes at strains beyond a threshold value. To bridge the gap between signal transmission, wireless communication and post-processing in wearable devices, the sensors are combined with electronics, allowing data transmission to a smartphone using a custom-developed application consisting of a user-friendly interface. The novel approaches reported here offer a wide range of practical applications, including medical diagnosis, health monitoring and patient healthcare.
Conceptual insights
A simple approach is developed for innovative design and assembly of new wearable electronics. Dual-functional wearable strain sensors and switches with tunable sensitivities and switching capabilities are prepared by simply tailoring the thickness of graphene foam. The sensors and switches are integrated with a smartphone using a custom-developed app consisting of a user-friendly interface. The integrated system is capable of monitoring, processing, transmitting and recognizing human physiological signals. The unique combination of highly stretchable and ultrasensitive strain sensors and switches based on sliced graphene foam–elastomer composites and information technology is a reliable, accurate and convenient approach to realize wearable wireless sensing systems for real-time health monitoring. The technology developed here has potential for application in emerging biomedical fields, such as home-care and telemedicine.
|
Introduction
Wearable electronics have received much attention due to their broad potential applications in human motion detection,1,2 human-machine interfaces,3 personalized health monitoring,4 electronic skins,5–9 and soft robotics.10 As an important component of wearable electronics, wearable strain sensors/switches should be mechanically flexible to allow conformal attachment to a curved surface and highly sensitive to reflect the level of deformation.11 Piezoresistive sensors and switches which transform mechanical strains to electrical signals12 have been widely used benefiting from their simple read-out mechanism, superior sensitivity, simplicity in device design and cost-effective fabrication.13–15 Although traditional piezoresistive strain sensors based on semiconductors and metal foils can be cost-effective, the majority of them could not serve as wearable strain sensors due to their low sensitivities or narrow sensing ranges below 5%.16 To develop stretchable and sensitive strain sensors, various nanoscale materials, including metal nanowires17,18 nanoparticles,19 silicon nanoribbons,20 carbon black,21 carbon nanotubes (CNTs),1,22–24 and graphene,25–31 have been explored as alternative materials coupled with elastomeric polymers as a supporting substrate. Among them, graphene is considered an ideal material for developing strain sensors due to its excellent electrical conductivity, unique optical properties and high flexibility.13,14,32
The sensitivity of a strain sensor is evaluated using the gauge factor (G-F), which is defined as (ΔR/R0)/ε, where ΔR/R0 is the relative resistance change and ε is the applied strain.33 The hexagonal atomic structure of graphene would be partially destroyed under an applied tensile strain, altering its electronic band structure and the electrical resistance.34–36 However, the sensitivity and stretchability of pristine graphene are relatively low because the sensing mechanism relies mainly on the opening of bandgaps. For example, sensors made from graphene grown by chemical vapor deposition (CVD) had G-Fs less than 14 at a maximum strain of ∼7%.37,38 The interconnected network of graphene has been altered using several different synthesis methods, including nanographene,29,39 graphene woven fabrics,40–42 graphene/polymer composites27,28 and graphene foam,33,43,44 to achieve enhanced sensitivities and stretchability. In light of the finding that small size graphene can offer more tunneling pathways, nanographene strain sensors presented G-Fs exceeding 500.29 However, these sensors had a very limited applicable strain range of 0–2%, greatly restricting their practical application. Graphene woven fabrics assembled by interweaving graphene ribbons showed a high sensitivity with a G-F of ∼35 at a 0.2% strain.41 Reduced graphene oxide (rGO) embedded in a polymer has also been explored in search of highly stretchable strain sensors. A rGO/nanocellulose composite exhibited a G-F of 7.1 at 100% strain.28 rGO with natural rubber has shown an ability to work at strains exceeding 800% with G-Fs up to 35.27 It should be noted that the aforementioned graphene structures are hard to be integrated with electronics using standard manufacturing processes.45 Three-dimensional (3D) graphene foam (GF) with unique interconnected networks has attracted great interest due to its excellent electrical and mechanical properties.33,46 However, the composites made from 3D GFs are unsuitable for strain sensors because of their stable electrical properties under various loading conditions with poor sensitivities.46 For example, GF/polydimethylsiloxane (PDMS) composites exhibited a resistance increase of 30% under 50% stretching, giving rise to a G-F of less than 1.46 In order to improve the sensitivity of GF-based composite strain sensors, a thin layer of polyethylene teraphthalate (PET) was introduced as a substrate.47 Although the change in electrical resistance increased by six times, the PET layer greatly limited the stretchability of the sensors. The PDMS composite strain sensors assembled from fragmentized GFs (FGFs) of 200–300 μm in size improved the G-F to 15–29 due to the increased contact area.33 It should be noted that the difficulties of controlling the size of FGFs hinder the accurate control of G-Fs of the sensors. Thus, it is necessary to find facile approaches to fabricate GF-based composites for highly sensitive and stretchable dual-functional strain sensors and switches.
The next generation of wearable devices is expected to be multifunctional, faster and compact without interrupting the users’ motions.48 For example, uninterrupted streaming of information is one of the most attractive features because it will allow continuous interaction with a nearby electronic device, such as most widely used smartphones. The electronic devices can automatically classify certain events, such as important medical events or an emergency situation, and provide timely intervention by alerting the users or inform the remote caregivers.49 In some cases, real-time intervention is not required, but the data need to be logged locally or uploaded to a server. As an example, the long-term monitoring of biomedical signals can enable early-stage diagnosis of health issues.50 Thus, one of the key functional requirements of wearable devices is wireless communication for fast and steady interactions and monitoring so as to make timely warning and/or recording.51 However, integrating the wearable sensors with wireless communication devices is a challenging task.
In this study, we employed an effective and facile approach to prepare highly stretchable, sliced GF/PDMS composites with tunable sensitivity and switching capabilities by simply controlling the thickness of GF. The composite thin films present unprecedented features, including high sensitivities, excellent stretchabilities and outstanding on/off switching ratios. Being able to detect both tiny and large deformations, various wearable devices, including acoustic sensors, pulse sensors, finger motion sensors, respiration monitor belts, neck posture correctors, pedometers and fall detectors, were demonstrated by integrating wearable sensors, a battery-powered measurement system and wireless communication with an internet-enabled smartphone. The integrated system can not only measure, display and analyze the collected signals, but also upload the collected data to the cloud to share information on health conditions, facilitating remote access to the results. These unique functional features and advantages have rarely been reported previously, thus this work offers a practical approach to greatly broaden and advance the applications of wearable electronics.
Results and discussion
Preparation and characterization
Fig. 1 presents the fabrication process of the proposed GF/PDMS composite thin films. The scanning electron microscopy (SEM) images (Fig. 2a and b) show that the 3D GF possessed well-defined interconnected graphene networks with a pore size of 100–300 μm. The rippled morphology of graphene with grain boundaries (Fig. 2c and d) was caused by the different thermal expansion between the Ni template and graphene46 and also a reflection of polycrystalline structure of the Ni template. The high-resolution TEM images shown in Fig. 2e indicate that the GF consisted of a few to ∼20 graphene layers, which is consistent with the Raman spectra (black line in Fig. 2f). To confirm the successful infiltration of PDMS into the porous GF, Raman spectra were also taken for pristine PDMS (red line in Fig. 2f) and the GF/PDMS composites (blue line in Fig. 2f). The very weak disordered D-band at ∼1330 cm−1 confirmed the high quality GFs with few defects synthesized in this study. The 2D band marginally upshifted from 2680 cm−1 to 2685 cm−1 after the infiltration of PDMS, caused probably by morphological changes of the graphene surface. The difference in PDMS distribution between the inner and outer graphene tubes generated local strains in the graphene lattice with increased interatomic spacing between the surrounding carbons.52
 |
| Fig. 1 Flow chart for preparation of GF/PDMS composites. | |
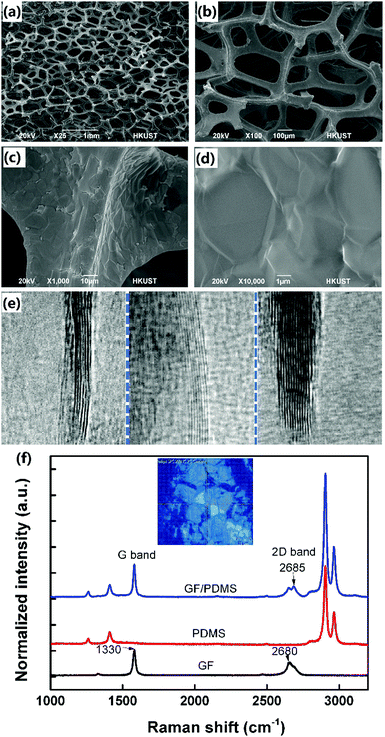 |
| Fig. 2 Characterization of GF. (a–d) Low to high-magnification SEM images of GF; (e) high-resolution TEM images show that the GF consists of a few to ∼20 graphene layers; (f) typical Raman spectra of GF, PDMS and the GF/PDMS composite. | |
GF/PDMS as strain sensors
We fabricated composite films having different thicknesses of ∼200, 400, 800 and 1600 μm sliced from identical GFs. The PDMS residue was removed from the GF surface using a scalpel. The GF/PDMS composites with an initial thickness of 1600 μm were sliced into pieces with different thicknesses. By reducing the thickness of the composite films, the resistance changed by over two orders of magnitude, from 149 Ω to 19.3 kΩ. To compare the sensitivities of composite film strain sensors with different piezoresistivities, the change in resistance (ΔR) was normalized to the initial resistance (R). Fig. 3a–c show the relative resistance change and G-Fs as a function of applied strain. The thinner the composite film, the higher the resistance change, offering a facile and effective means to tune the sensitivity by simply controlling the composite film thickness. Traditional piezoresistive sensors made of homogeneous metallic materials usually possess constant G-Fs, which greatly limits their broad applications. The composite strain sensors demonstrated here have several unique advantages, such as ease of tuning the G-Fs and stretchability so as to meet different requirements for different applications.26 As shown in Fig. 3d and e, the composite strain sensors can be used for both high-strain applications with low G-Fs and low-strain applications with high G-Fs. In other words, the thin composite sensors with a high initial resistance are particularly suited for applications requiring high G-Fs at low-strain service environments, whereas the thick composite sensors with a low initial resistance are appropriate for applications requiring moderate G-Fs at very high-strain service conditions. For example, we can fabricate strain sensors with a G-F of ∼5 and stretchability of 70%, or alternatively sensors with a G-F of ∼20 and stretchability of 25%. In comparison with conventional metal-based strain sensors (having G-Fs of 1–5 with a stretchability of 5%),13,53 Ag NW/polymer composites (having a G-F of ∼5 with a stretchability of 5%),17 ZnO NW/polymer composites (having a G-F of ∼116 with a stretchability of 50%),54 CNT/polymer composites (having a G-F of ∼0.82 with a stretchability of 40%),1 nanographene (having G-Fs of 300–600 with a stretchability of 1.6%),29,39 graphene woven fabrics (having a G-F of ∼500 with a stretchability of 8%),40–42 and graphene/polymer composites (having G-Fs of 1.6–7.1 with a stretchability of 100%),28 the current GF/PDMS strain sensors simultaneously deliver an excellent linearity, sensitivity and stretchability. In addition, the response of the GF/PDMS composite under repeated stretch–release cycles from 0% to 10% strain is recorded (Fig. S3, ESI†). It is seen that the peaks and valleys of resistance change remained almost identical even after 1000 cycles, indicating superior repeatability and durability.
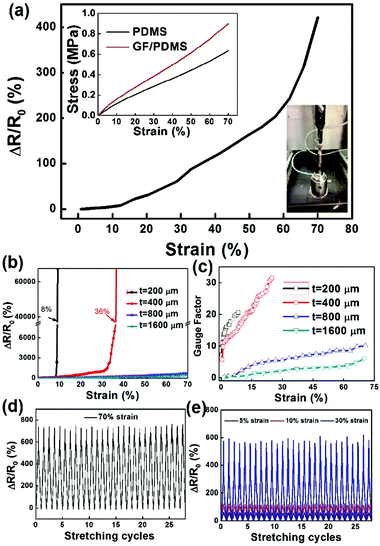 |
| Fig. 3 Piezoresistive properties of GF/PDMS composite films with different thicknesses (∼200, 400, 800 and 1600 μm) and initial resistances (19.3, 4.3, 1.3 and 0.149 kΩ respectively). (a) Resistance change of the composite film (1.6 mm) as a function of tensile strain; the corresponding stress–strain is shown in the inset. (b and c) Resistance change (b) and G-F (c) of composites with different thicknesses (200–1600 μm). (d and e) Durability test results for 800 μm (d) and 400 μm thick (e) composite films. | |
GF/PDMS as electromechanical switches
It is worth noting that the ultrathin composite films can serve not only as strain sensors, but also as electromechanical switches. The switching capability was realized by identifying unusual resistance changes vs. applied strain. Fig. 4a shows the piezoresistive response of the composite films with a thickness of 400 μm in tension. The resistance change initially increased almost linearly at strains up to 36%, followed by a sudden surge in resistance change by almost three orders of magnitude as the strain increased beyond the switching strain (εs) of 36%. Once the strain was released, the resistance was fully recovered to the initial value. These unique piezoresistive characteristics can make the composite films ideal electromechanical switches. For example, when the composite film is used as a power switch, the switch circuit is closed at a low strain level due to its conductive characteristic and the switch shows the “on” state. When the strain increases beyond εs, the switch circuit is open due its nonconductive characteristic and the switch shows the “off” state. One attractive feature of the composite switch is that εs can be rationally controlled to satisfy the desirable operating strains by adjusting the number of conduction paths. As shown in Fig. 3b, the εs values of composite films could be tailored between 8% and 36% by controlling the film thickness from 200 to 400 μm. εs can be further tuned over a wide range of strains for various applications by choosing different polymer matrices or optimizing chemical vapor deposition (CVD) growth conditions of GFs.
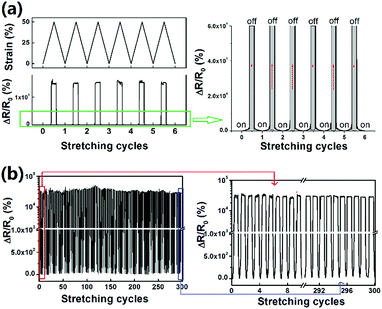 |
| Fig. 4 Performance of the electromechanical switch made of GF/PDMS composites. (a) Electromechanical switch (400 μm) with “on” and “off” states. (b) Multi-cycle (300 cycles) periodic switching of the 200 μm thick GF/PDMS composite between 0 and 30% maximum strain. | |
It should be noted that the strain-induced switching of the composite films was highly reversible and repeatable. Fig. 4b shows the relative resistance change of the composite film upon cyclic loading. The switching between the stretched and released states of the composite film was robust and maintained an on/off switching ratio in excess of 3 orders of magnitude after 300 cycles, confirming the excellent cyclic stability of the switch. The high durability of the switch is attributed to the repeated opening and closure of the microcracks formed on the graphene tubes during cycles. Fig. 5a–d indicate that both intergranular and transgranular cracks initiated and propagated, especially at high strains. Upon loading, the microcracks gradually opened up and eventually destroyed the conducting pathways, leading to an “off” state. As observed in Fig. 5e and f, although the cracks are opened up under load, they are closed upon release of the load because of the surrounding highly flexible PDMS matrix which holds the whole GF structure, allowing repeatable opening and closing of microcracks. With the microcracks being gradually closed upon unloading, the separated graphene tubes were reinstated, presenting the “on” state. The opening and closure of microcracks were highly repeatable, making the switch capable of numerous connections/disconnections without damage.55,56 As Table 1 may suggest, the main objective of this work is to construct graphene structures with a wide range of stretchabilities with low resistivities, easily tunable sensitivities and new switching behaviours, rather than achieving extremely high sensitivities with high gauge factors.
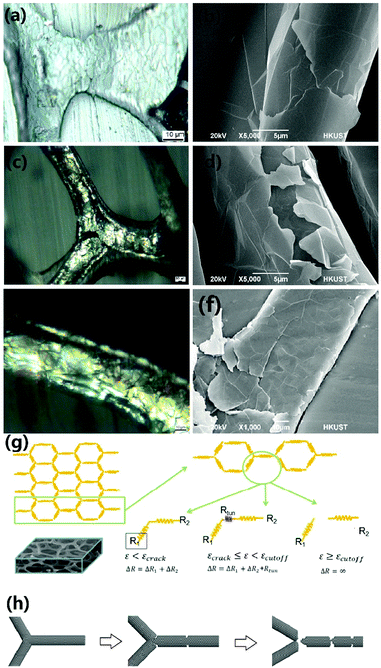 |
| Fig. 5 Strain sensing and switching mechanisms. Optical (a, c and d) and SEM (b, d and e) images of the initial undamaged graphene (a and b), zip-like crack junctions (c and d) after applying 30% strain, and the closure of microcracks (e and f) after the release of strain. (g) Change in electrical interconnection and resistance at different tensile strains: (i) complete Ohmic connection, (ii) tunneling between neighboring graphene tubes and (iii) complete disconnection due to microcracks. (h) A molecular model showing crack initiation and propagation at the junction and on the surface of graphene tubes at different tensile strains. | |
Table 1 Comparison of sensing/switching performance with other representative graphene-based structures
Graphene structure |
Material |
Initial resistance |
G-F |
Stretchability (%) |
Strain switch |
Ref. |
Sliced GF |
Sliced GF/PDMS composite |
∼0.15 kΩ |
3.3–24.1 |
70 |
Yes |
Current work |
Graphene film |
Graphene ripples on PDMS |
3.6 kΩ |
∼2 |
20 |
No |
25
|
Patterned graphene on PDMS |
∼500 Ω sq−1 |
2.4–14 |
7.1 |
No |
37
|
Graphene woven fabric |
Graphene woven fabric/PDMS composite |
∼0.1–1 kΩ |
223 |
3 |
No |
42
|
Graphene woven fabric on PDMS |
— |
500 |
2 |
No |
40
|
Graphene yarn |
Graphene on stretchable yarns |
— |
∼0.1–1.4 |
50–150 |
No |
66
|
Graphene on double-covered yarn |
— |
3.7–10 |
50 |
No |
30
|
GF |
GF/PDMS composite |
— |
2.6–8.5 |
45% |
No |
67
|
GF/PDMS composite for detecting frequency signals |
∼30 Ω |
— |
— |
No |
68
|
GF/PDMS composite |
— |
6.24 |
15 |
No |
47
|
Fragmentized GF/PDMS composite |
0.47–1.8 kΩ |
15–29 |
70 |
No |
33
|
GF made from graphene oxide via chemical reduction |
∼3.5 kΩ |
98.66 |
30 |
No |
44
|
Graphene aerogel |
Graphene aerogel made from graphene oxide via chemical reduction – induced self-assembly |
— |
Up to 61.3 |
19 |
No |
69
|
Reduced graphene oxide aerogel/polyimide composite |
— |
∼0.5 |
5 |
No |
70
|
The cross-section SEM images and the schematics of the corresponding sliced GFs with different thicknesses of 200 μm (a), 400 μm (b), 800 μm (c), and 1600 μm (d) are shown in Fig. S1 (ESI†). To help understand the difference in the conductive network, they are shown and drawn at different magnifications. It is seen that the thicker GFs possessed more extensive percolating networks because the relative size of the graphene tubes which provide conductive pathways became smaller with increasing sample thickness. Upon external loading, cracks are inevitably generated and gradually propagate on the strained graphene tubes, giving rise to increase in resistance. It is expected that the thicker GFs would have a higher chance of maintaining a larger number of conductive networks even after extensive damage to the graphene tubes. In particular, there was a large difference in graphene tube density between the films of 400 and 800 μm thickness (Fig. S1, ESI†). This may explain the significant difference in change of resistance between these two films.
Sensing and switching mechanisms
The electrical resistance of the composite films was analyzed in reference to the schematics in Fig. 5g and h. The initial resistance, R, consists of two major components, namely:57where RI is the inherent resistance of graphene tubes and RT is the electron tunneling resistance due to the cracked graphene. The change in RI caused by tension is relatively small compared to the dominant tunneling resistance, thus the resistance change under strain can be approximated:
According to the Simmons function,29,58 the rate of resistance change caused by tunneling can be described as:
| 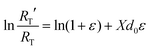 | (3) |
where
RT′ is the tunneling resistance under strain,
ε,
X describes the tunneling barrier's height dependence and
d0 is the original tunneling distance. It follows then that G-F is given by:
| 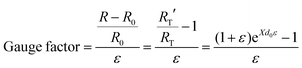 | (4) |
It is seen that G-F is affected by the original tunneling gap, d0, and a high G-F can be achieved by reducing the thickness of the sensors to increase d0.39 This explains the observed phenomenon that the thinner composite films possessed higher sensitivities. However, if the tunneling gap becomes too large by complete cracking with a fully disconnected network (see final stage of Fig. 5h), it is difficult for the electron charges to transport from one side of the cracked graphene tube to another, leading to a surge in electrical resistance.
Demonstration of GF/PDMS composite films as wearable sensors
Integration of the wearable monitoring device and android.
For ultimate user convenience and widespread acceptance by the general public, the next-generation wearable devices should be able to communicate with personal electronic devices, especially commercially available smartphones. By seamlessly interconnecting the composite sensors, a Bluetooth module and an external device like a smartphone, we developed a fully integrated wearable human motion monitoring system. Fig. 6 presents a schematic diagram of the wearable system composed of the composite sensors, a signal analyzer, a Bluetooth transmitter and a smartphone. The on-body measurements were facilitated by signal transduction, conditioning, processing and wireless transmission. Bluno Beetle with computational and serial communication capabilities was used to analyze the resistance change of the sensors. The piezoresistive signals generated from Bluno Beetle were transmitted over the wireless Bluetooth communication link, which has been extensively used to connect with external devices. As shown in ESI,† Movie 1, the smartphone displayed in real time the signals acquired from the measured resistance changes. The custom-built Android application containing a user-friendly interface with a minimal complex design saved the collected results in a text file, which could be shared, via short message service (SMS) or email, or uploaded to cloud servers. This feature is particularly useful for people who monitor their health conditions at home and have to share the information with their remote caregivers. The real-time display and uploading of the data using the wearable human motion monitoring system are shown in ESI,† Movie 2.
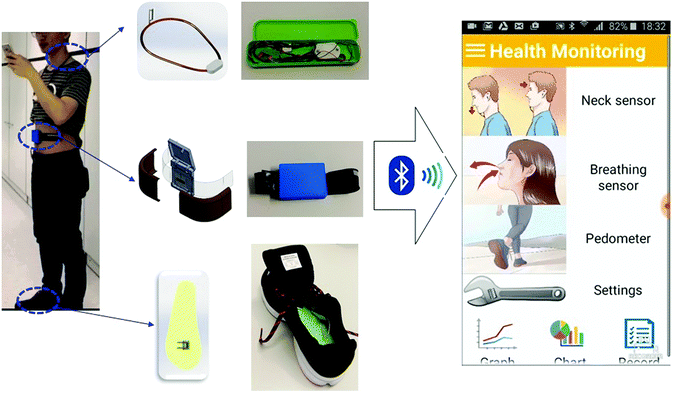 |
| Fig. 6 Custom-developed mobile application for data display and aggregation: photograph of wireless and real-time sensing using GF/PDMS sensors integrated with a Bluetooth system. | |
Acoustic sensors.
Voice actions are an important part of wearable experiences, thus we first demonstrated the potential application of the composite film as a wearable acoustic sensor. When saying different words, the throat muscle has different degrees of stretching and shrinking in various directions.59 To capture physiological waves generated in the throat, the composite sensor was affixed onto the throat to collect the voice signals. The flexible composite sensor was stretched or compressed along with the throat skin and ESI,† Movie 3, Fig. S6 and S7 show examples of signal waveforms generated when English letters and phrases were voiced. It is shown that the amplitudes, durations and resistance changes for different words were highly discernable, while the characteristic peaks and valleys of the waves were similar for the identical words. It is envisaged that the novel acoustic sensor with excellent voice recognition should be able to handle more complex acoustic systems and can be used as a wearable voice controller if wireless transmission and/or artificial intelligence is incorporated into the system. It may also be possible to help patients who have voice troubles collect and reproduce their voice.15,60
Pulse sensors.
Any changes to the mental or physical state of a person may cause a change in pulse because the heart rate or pulse alters according to the body's need.61 Therefore, pulse is one of the most important signs to assess one's health status. The radial pulse (measured at the wrist) and the brachial pulse (measured at the upper arm near the elbow) are the key indicators of heart rate, and can indicate health condition for non-invasive medical diagnosis.60 As shown in Fig. 7a and b, the flexibility of composite films was sufficient to make a conformal contact to detect minute strains from both the radial pulse and brachial pulse. The measured pulse rate was ∼60 times per min, which is within the normal value of a healthy adult.
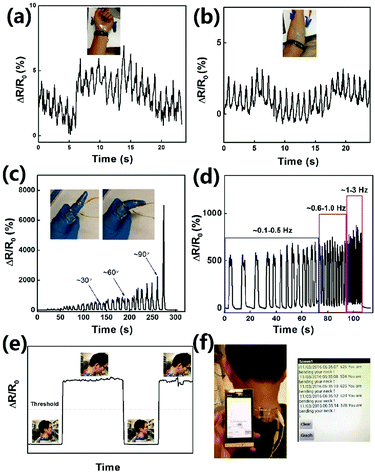 |
| Fig. 7 (a and b) Relative resistance changes in the strain sensors due to radial (a) and brachial pulses (b). (c and d) Relative resistance changes in the strain sensors attached on a glove due to bending of the index finger to different angles (c) and at different speeds (d). (e and f) A neck posture corrector that can mitigate neck pains or prevent myopia arising from texting. (a) Relative resistance change in the strain switch due to neck bending. (b) A warning system of neck bending. | |
Finger motion sensors.
It is demonstrated that the composite strain sensor was able to detect both weak physical stimuli in healthcare and large human motions. The sensor was attached on a gloved forefinger to monitor the finger motions with different bending states and speeds. Since GF would be compressed and stretched under bending, an approximately linear response was observed between the resistance change and the degree of bending (Fig. 7c). Upon returning to its original position, the resistance change was fully recovered, confirming the sensitivity and flexibility of the sensor. Fig. 7d shows the resistance variation corresponding to finger-bending motion under both slow and rapid bending speeds. The sensor was also able to distinguish the different bending speeds by showing increased frequencies for higher speed bending and vice versa. The capabilities of detecting both the bending degree and speed make the composite strain sensor an excellent candidate for human-machine interface applications.
Respiration monitoring belts.
The respiratory rate, which gives meaningful information on respiratory system performance, is another important physiological parameter that needs to be monitored over extended periods of time to estimate the basic health-status of patients.61 The rate of respiration is commonly counted visually by observing the rising and falling of the chest in most clinical settings where the information regarding the depth of respiration is missing.62 Here, a respiration monitoring belt based on thoracic expansion measurement was fabricated by integrating the strain sensor into a conventional belt to measure human respiration rates. The composite strain sensor was repeatedly stretched and released due to the expansion of thoracic or abdominal part during inhalation and exhalation, causing resistance changes. ESI,† Video 4 shows the results obtained from real-time respiration testing, confirming that the respiration monitoring belt was able to record breathing patterns for different situations, such as normal breathing and deep breathing.
Neck posture correctors.
Excessive texting and overuse of portable electronic devices, such as smartphones, tablets and laptops, have created “text-neck” which has become a new medical term that impacts a vast majority of people.63 The repeated stress arising from bending the neck while looking down at the screens of mobile devices or long periods of texting is the main reason for “text-neck”.64 The strain switches with ultrahigh switching ratios are suitable for integration with working systems as a neck posture corrector. As shown in Fig. 7e and f, the neck posture corrector was attached to the back of the neck to measure the degree of bending, giving instantaneous warnings by sensing the neck bending. Once the sensor receives sensing signals, the signals will be wirelessly forwarded to the smartphone which responds with warning action, like phone vibration or voice reminder (see ESI,† Video 5). Apart from protecting the neck, the neck posture corrector has a variety of potential applications, such as the prevention of drowsy driving. It is found that the percentage of correct prediction of drowsiness by the bending angle of the neck was 85%.65 Thus, the warning system can also be used to monitor drivers’ arousal level and warn drivers of a risk of falling asleep to ensure safe driving.
Pedometers.
A pedometer was designed by mounting the strain sensor on the insole and embedding the signal collection and transmission module into the shoe. The pedometer mechanism of our sensor lies in bending and relaxation of the sensor on the insole, leading to a sharp increase and reduction of electrical resistance. As shown in SI Video 6, the pedometer can accurately record the number of steps during walking. Gait or walking pattern is another general indicator of an individual's health condition.71,72 The gaits of persons with illnesses are quite different from those of healthy individuals, making it possible to give a preliminary diagnosis of various elderly diseases, such as the early onset of Alzheimer's.73 Once the correlations between the gait signals and various diseases are identified, the developed pedometer may be able to quantify, classify and interpret the information.
Fall detectors.
With sharp increases in the elderly population especially in developed countries, falls become critical events that need particular attention from the healthcare viewpoint. For example, two thirds of injury-related hospitalizations among elderly people are caused by falls.74 A fall event may not only lead to physical damage, like bone fractures and skin abrasions, but also influence the psychological health, which drastically reduces the self-confidence and independence.75 Although there are several approaches for detecting falls using accelerometers,76 they are either too complicated or uncomfortable to wear. We designed a smartphone-based fall detection system capable of recognizing a fall and informing the caregivers. As shown in Fig. S9 (ESI†), a pressure sensor was developed to distinguish between the falling and walking motions. The graphene foam sensor was suspended on cavities to enhance the sensitivity. Simple fall detection can be realized by observing the sudden resistance change of the pressure sensor resulting in impulsive signals above a predefined threshold. Once a fall is detected, the designed Android app will request immediate help via text message, voice calls, or transmitting an alert messages to a central server for immediate assistance (see ESI,† Video 7). The fall detection system is also valuable for individuals working in high-risk environments where constant safety monitoring is needed.
Conclusions
We have developed novel flexible and stretchable piezoresistive sensors and switches based on interconnected networks of graphene for a wide range of applications (see Table S1, ESI†). The dual-functional composite sensors and switches possessed excellent mechanical flexibility and durability. The cracking-induced resistance change enabled the GF/PDMS composites to serve as wearable strain sensors and switches with excellent sensitivities, stretchability and on/off switching capabilities. The skin-conforming flexible composite sensors were integrated with circuits to realize real-time monitoring, processing and wireless transmission of human physiological parameters, including acoustics, pulse, finger motion and respiration. The integrated monitoring system was capable of collecting and transmitting the sensed data to a smartphone through Bluetooth communication. The custom-built Android app was developed to share the data with caregivers by uploading the information to the cloud. A wireless neck posture corrector was developed to monitor the neck bending and give warnings through a voice function of smartphones, making it potentially suited for other applications, e.g. for prevention of drowsy driving. A fully embedded pedometer and a wearable fall detector were also designed and fabricated to monitor the walking patterns and possible falls, both of which are crucial to mobile health monitoring for the elderly. Our sensor and switch systems may expand their application to medical fields for wireless remote diagnosis and treatment of patients in the near future.
Experimental
Fabrication of GFs
GFs were synthesized by CVD using a horizontal tube furnace system (OTF-1200X-II-80-SL, Hefei Kejing Materials) following our previously reported procedure.77 Ni foams (Heze Tianyu Technology Development Co. with a density of 320 g m−2, 1.6 mm thick) were cleaned by ultrasonication in acetone followed by rinsing in deionized (DI) water. The Ni template with a rectangular shape (50 mm × 100 mm) was put into a quartz tube with an initial base pressure of 5 mTorr. The temperature was raised to 1000 °C with Ar (500 ml min−1) and H2 (200 ml min−1) flow followed by annealing for 10 min. Methane (CH4) precursor at a concentration of 2.8 vol% was introduced for 20 min followed by rapid cooling to grow graphene on the template surface. Freestanding GFs were obtained by immersing the graphene-Ni foam in a 0.5 M FeCl3/1 M HCl mixture at 80 °C for 2 h to remove the Ni template. The residual chemicals within the GFs were removed by rinsing using hot DI water, followed by drying.
Fabrication of GF/PDMS dual-functional strain sensors and switches
PDMS (Sylgard 184, Dow Corning) prepolymer was prepared by mixing base silicone gel and a curing agent at a 10
:
1 weight ratio, followed by degassing in a vacuum oven for 30 min. The as-prepared GF was completely immersed into the PDMS mixture, which was placed in a vacuum oven for 2 h at 80 °C to allow infiltration of PDMS into the GF and curing. After removing excessive PDMS adhered on the GF surface, the 3D GF/PDMS composite (1.6 mm) was cut into thin films with different thicknesses of 800, 400 and 200 μm using a scalpel. The thickness was precisely controlled by measuring the cutting position using a Vernier caliper. Finally, copper tapes were glued to the two ends of a composite film using silver conductive paste to form a strain sensor.
Device integration
The composite sensors were connected to a resistance analyzer (DFR0339-Bluno beetle and HC06-Bluetooth Module), which was wirelessly controlled using an Android application. The data measured by the wearable sensors were transmitted to an Android smartphone through Bluetooth communication. The Android app was designed and implemented in the MIT App Inventor development environment, which had three main functions. (i) The raw data received from the sensors were displayed and stored automatically. (ii) The app could upload the collected results to the cloud for caregiver's access to data. (iii) The app was automatically directed to a warning action or an emergency call once abnormal conditions were detected.
Characterization
A scanning electron microscope (SEM, JEOL JSM-6390F), transmission electron microscope (TEM, JEOL 2010) and optical microscope (Olympus BX51M) were used to characterize the microstructures and morphologies of the freestanding GFs and GF/PDMS composites. Raman spectra were obtained using a Micro-Raman spectrometer (Renishaw Micro-Raman /Photoluminescence System) with a He–Ne laser source at an excitation wavelength of 633 nm. The electromechanical characterization was performed by loading the strain sensors in tension on a universal testing machine (MTS Alliane RT-5) at a cross-head speed of 1 mm min−1. The electrical resistance was continuously monitored using a digital multimeter (34970A Data Acquisition/Data Logger Switch Unit, Agilent) while the sensors were under load.
Author contributions
The manuscript was written through contributions of all authors. All authors have given approval to the final version of the manuscript. Q. B. Z., X. L., and J. K. K. conceived the idea. Q. B. Z. and X. L. prepared the materials. Q. B. Z., X. L., X. S., Z. Y. W. and Y. W. conducted the mechanical and electrical tests of the materials. Q. B. Z., X. L. and H. R. X. conducted the material characterization. Q. B. Z. built the models and analyzed the data. M. S. C., Y. W. C., H. C. H., and H. Y. L. designed the devices, packaged the hardware and wrote the programs for the smartphone App. S. Y. K. provided suggestions in the smartphone App part. Q. B. Z. and X. L. wrote the manuscript instructed by J. K. K. with input from all the authors.
Conflicts of interest
There are no conflicts to declare.
Acknowledgements
This project was financially supported by the Research Grants Council of Hong Kong SAR (GRF projects: 16229216, 16209917 and 16205517), Initiation Grant for New Faculty (IGN15EG06) and Postgraduate Scholarships from the Centre for 1D/2D Quantum Materials at HKUST. Technical assistance from the Advanced Engineering Materials facilities (AEMF) and the Materials Characterization and Preparation Facilities (MCPF) of HKUST is appreciated. The project “Wearable Graphene Foam/Rubber Composite Sensors for Remote Health Monitoring of Patients” was awarded “The Disney-HKUST Grant for Technology and Wellbeing”, “The Hong Kong X FYP Supporting Scheme” and The Best Project Award of “IMechE Best Project Award Competition 2016–2017”.
References
- T. Yamada, Y. Hayamizu, Y. Yamamoto, Y. Yomogida, A. Izadi-Najafabadi, D. N. Futaba and K. Hata, Nat. Nanotechnol., 2011, 6, 296–301 CrossRef CAS PubMed.
- T. T. Yang, D. Xie, Z. H. Li and H. W. Zhu, Mater. Sci. Eng., R., 2017, 115, 1–37 CrossRef.
- S. Lim, D. Son, J. Kim, Y. B. Lee, J. K. Song, S. Choi, D. J. Lee, J. H. Kim, M. Lee, T. Hyeon and D. H. Kim, Adv. Funct. Mater., 2015, 25, 375–383 CrossRef CAS.
- S. Lee, A. Reuveny, J. Reeder, S. Lee, H. Jin, Q. H. Liu, T. Yokota, T. Sekitani, T. Isoyama, Y. Abe, Z. G. Suo and T. Someya, Nat. Nanotechnol., 2016, 11, 472–478 CrossRef CAS PubMed.
- Z. Lou, S. Chen, L. L. Wang, K. Jiang and G. Z. Shen, Nano Energy, 2016, 23, 7–14 CrossRef CAS.
- D. H. Ho, Q. Sun, S. Y. Kim, J. T. Han, D. H. Kim and J. H. Cho, Adv. Mater., 2016, 28, 2601–2608 CrossRef CAS PubMed.
- J. Ge, L. Sun, F. R. Zhang, Y. Zhang, L. A. Shi, H. Y. Zhao, H. W. Zhu, H. L. Jiang and S. H. Yu, Adv. Mater., 2016, 28, 722–728 CrossRef CAS PubMed.
- S. Y. Hong, Y. H. Lee, H. Park, S. W. Jin, Y. R. Jeong, J. Yun, I. You, G. Zi and J. S. Ha, Adv. Mater., 2016, 28, 930–935 CrossRef CAS PubMed.
- M. Park, Y. J. Park, X. Chen, Y. K. Park, M. S. Kim and J. H. Ahn, Adv. Mater., 2016, 28, 2556–2562 CrossRef CAS PubMed.
- S. Bauer, S. Bauer-Gogonea, I. Graz, M. Kaltenbrunner, C. Keplinger and R. Schwodiauer, Adv. Mater., 2014, 26, 149–162 CrossRef CAS PubMed.
- S. F. Zhao, G. Y. Zhang, Y. J. Gao, L. B. Deng, J. H. Li, R. Sun and C. P. Wong, ACS Appl. Mater. Interfaces, 2014, 6, 22823–22829 CAS.
- C. Pang, G. Y. Lee, T. I. Kim, S. M. Kim, H. N. Kim, S. H. Ahn and K. Y. Suh, Nat. Mater., 2012, 11, 795–801 CrossRef CAS PubMed.
- M. Amjadi, K. U. Kyung, I. Park and M. Sitti, Adv. Funct. Mater., 2016, 26, 1678–1698 CrossRef CAS.
- T. Q. Trung and N. E. Lee, Adv. Mater., 2016, 28, 4338–4372 CrossRef CAS PubMed.
- Y. C. Lai, B. W. Ye, C. F. Lu, C. T. Chen, M. H. Jao, W. F. Su, W. Y. Hung, T. Y. Lin and Y. F. Chen, Adv. Funct. Mater., 2016, 26, 1286–1295 CrossRef CAS.
- C. Y. Wang, X. Li, E. L. Gao, M. Q. Jian, K. L. Xia, Q. Wang, Z. P. Xu, T. L. Ren and Y. Y. Zhang, Adv. Mater., 2016, 28, 6640–6648 CrossRef CAS PubMed.
- M. Amjadi, A. Pichitpajongkit, S. Lee, S. Ryu and I. Park, ACS Nano, 2014, 8, 5154–5163 CrossRef CAS PubMed.
- B. U. Hwang, J. H. Lee, T. Q. Trung, E. Roh, D. I. Kim, S. W. Kim and N. E. Lee, ACS Nano, 2015, 9, 8801–8810 CrossRef CAS PubMed.
- M. Segev-Bar and H. Haick, ACS Nano, 2013, 7, 8366–8378 CrossRef CAS PubMed.
- J. Kim, M. Lee, H. J. Shim, R. Ghaffari, H. R. Cho, D. Son, Y. H. Jung, M. Soh, C. Choi, S. Jung, K. Chu, D. Jeon, S. T. Lee, J. H. Kim, S. H. Choi, T. Hyeon and D. H. Kim, Nat. Commun., 2014, 5, 5747 CrossRef CAS PubMed.
- N. S. Lu, C. Lu, S. X. Yang and J. Rogers, Adv. Funct. Mater., 2012, 22, 4044–4050 CrossRef CAS.
- J. Park, Y. Lee, J. Hong, Y. Lee, M. Ha, Y. Jung, H. Lim, S. Y. Kim and H. Ko, ACS Nano, 2014, 8, 12020–12029 CrossRef CAS PubMed.
- S. Ryu, P. Lee, J. B. Chou, R. Z. Xu, R. Zhao, A. J. Hart and S. G. Kim, ACS Nano, 2015, 9, 5929–5936 CrossRef CAS PubMed.
- Z. Y. Liu, D. P. Qi, P. Z. Guo, Y. Liu, B. W. Zhu, H. Yang, Y. Q. Liu, B. Li, C. G. Zhang, J. C. Yu, B. Liedberg and X. D. Chen, Adv. Mater., 2015, 27, 6230–6237 CrossRef CAS PubMed.
- Y. Wang, R. Yang, Z. W. Shi, L. C. Zhang, D. X. Shi, E. Wang and G. Y. Zhang, ACS Nano, 2011, 5, 3645–3650 CrossRef CAS PubMed.
- M. Hempel, D. Nezich, J. Kong and M. Hofmann, Nano Lett., 2012, 12, 5714–5718 CrossRef CAS PubMed.
- C. S. Boland, U. Khan, C. Backes, A. O'Neill, J. McCauley, S. Duane, R. Shanker, Y. Liu, I. Jurewicz, A. B. Dalton and J. N. Coleman, ACS Nano, 2014, 8, 8819–8830 CrossRef CAS PubMed.
- C. Y. Yan, J. X. Wang, W. B. Kang, M. Q. Cui, X. Wang, C. Y. Foo, K. J. Chee and P. S. Lee, Adv. Mater., 2014, 26, 2022–2027 CrossRef CAS PubMed.
- J. Zhao, G. L. Wang, R. Yang, X. B. Lu, M. Cheng, C. L. He, G. B. Xie, J. L. Meng, D. X. Shi and G. Y. Zhang, ACS Nano, 2015, 9, 1622–1629 CrossRef CAS PubMed.
- Y. Cheng, R. R. Wang, J. Sun and L. Gao, Adv. Mater., 2015, 27, 7365–7371 CrossRef CAS PubMed.
- Y. Lin, S. Q. Liu, S. Chen, Y. Wei, X. C. Dong and L. Liu, J. Mater. Chem. C, 2016, 4, 6345–6352 RSC.
- H. Jang, Y. J. Park, X. Chen, T. Das, M. S. Kim and J. H. Ahn, Adv. Mater., 2016, 28, 4184–4202 CrossRef CAS PubMed.
- Y. R. Jeong, H. Park, S. W. Jin, S. Y. Hong, S.
S. Lee and J. S. Ha, Adv. Funct. Mater., 2015, 25, 4228–4236 CrossRef CAS.
- V. M. Pereira and A. H. C. Neto, Phys. Rev. Lett., 2009, 103, 046801 CrossRef PubMed.
- M. Y. Huang, T. A. Pascal, H. Kim, W. A. Goddard and J. R. Greer, Nano Lett., 2011, 11, 1241–1246 CrossRef CAS PubMed.
- J. F. Zang, S. Ryu, N. Pugno, Q. M. Wang, Q. Tu, M. J. Buehler and X. H. Zhao, Nat. Mater., 2013, 12, 321–325 CrossRef CAS PubMed.
- S. H. Bae, Y. Lee, B. K. Sharma, H. J. Lee, J. H. Kim and J. H. Ahn, Carbon, 2013, 51, 236–242 CrossRef CAS.
- Y. Lee, S. Bae, H. Jang, S. Jang, S. E. Zhu, S. H. Sim, Y. I. Song, B. H. Hong and J. H. Ahn, Nano Lett., 2010, 10, 490–493 CrossRef CAS PubMed.
- J. Zhao, C. L. He, R. Yang, Z. W. Shi, M. Cheng, W. Yang, G. B. Xie, D. M. Wang, D. X. Shi and G. Y. Zhang, Appl. Phys. Lett., 2012, 101, 063112 CrossRef.
- T. T. Yang, W. Wang, H. Z. Zhang, X. M. Li, J. D. Shi, Y. J. He, Q. S. Zheng, Z. H. Li and H. W. Zhu, ACS Nano, 2015, 9, 10867–10875 CrossRef CAS PubMed.
- Y. Wang, L. Wang, T. T. Yang, X. Li, X. B. Zang, M. Zhu, K. L. Wang, D. H. Wu and H. W. Zhu, Adv. Funct. Mater., 2014, 24, 4666–4670 CrossRef CAS.
- X. Liu, C. Tang, X. H. Du, S. Xiong, S. Y. Xi, Y. F. Liu, X. Shen, Q. B. Zheng, Z. Y. Wang, Y. Wu, A. Horner and J. K. Kim, Mater. Horiz., 2017, 4, 477–486 RSC.
- J. Kuang, L. Q. Liu, Y. Gao, D. Zhou, Z. Chen, B. H. Han and Z. Zhang, Nanoscale, 2013, 5, 12171–12177 RSC.
- J. H. Li, S. F. Zhao, X. L. Zeng, W. P. Huang, Z. Y. Gong, G. P. Zhang, R. Sun and C. P. Wong, ACS Appl. Mater. Interfaces, 2016, 8, 18954–18961 CAS.
- X. M. Li, T. T. Yang, Y. Yang, J. Zhu, L. Li, F. E. Alam, X. Li, K. L. Wang, H. Y. Cheng, C. T. Lin, Y. Fang and H. W. Zhu, Adv. Funct. Mater., 2016, 26, 1322–1329 CrossRef CAS.
- Z. P. Chen, W. C. Ren, L. B. Gao, B. L. Liu, S. F. Pei and H. M. Cheng, Nat. Mater., 2011, 10, 424–428 CrossRef CAS PubMed.
- R. Q. Xu, Y. Q. Lu, C. H. Jiang, J. Chen, P. Mao, G. H. Gao, L. B. Zhang and S. Wu, ACS Appl. Mater. Interfaces, 2014, 6, 13455–13460 CAS.
- W. Gao, S. Emaminejad, H. Y. Y. Nyein, S. Challa, K. V. Chen, A. Peck, H. M. Fahad, H. Ota, H. Shiraki, D. Kiriya, D. H. Lien, G. A. Brooks, R. W. Davis and A. Javey, Nature, 2016, 529, 509–514 CrossRef CAS PubMed.
- A. J. Bandodkar, I. Jeerapan and J. Wang, ACS Sens., 2016, 1, 464–482 CrossRef CAS.
- S. M. Lee, H. J. Byeon, J. H. Lee, D. H. Baek, K. H. Lee, J. S. Hong and S. H. Lee, Sci. Rep., 2014, 4, 6074 CrossRef CAS PubMed.
- J. Park, J. Kim, K. Kim, S. Y. Kim, W. H. Cheong, K. Park, J. H. Song, G. Namgoong, J. J. Kim, J. Heo, F. Bien and J. U. Park, Nanoscale, 2016, 8, 10591–10597 RSC.
- M. A. Bissett, M. Tsuji and H. Ago, J. Phys. Chem. C, 2013, 117, 3152–3159 CAS.
- R. R. He and P. D. Yang, Nat. Nanotechnol., 2006, 1, 42–46 CrossRef CAS PubMed.
- X. Xiao, L. Y. Yuan, J. W. Zhong, T. P. Ding, Y. Liu, Z. X. Cai, Y. G. Rong, H. W. Han, J. Zhou and Z. L. Wang, Adv. Mater., 2011, 23, 5440–5444 CrossRef CAS PubMed.
- S. L. Gao, R. C. Zhuang, J. Zhang, J. W. Liu and E. Mader, Adv. Funct. Mater., 2010, 20, 1885–1893 CrossRef CAS.
- J. Zhang, J. W. Liu, R. C. Zhuang, E. Mader, G. Heinrich and S. L. Gao, Adv. Mater., 2011, 23, 3392–3397 CrossRef CAS PubMed.
- Y. Yu, G. Song and L. Sun, J. Appl. Phys., 2010, 108, 084319 CrossRef.
- T. T. Yang, X. M. Li, X. Jiang, S. Y. Lin, J. C. Lao, J. D. Shi, Z. Zhen, Z. H. Li and H. W. Zhu, Mater. Horiz., 2016, 3, 248–255 RSC.
- Y. Wang, T. T. Yang, J. C. Lao, R. J. Zhang, Y. Y. Zhang, M. Zhu, X. Li, X. B. Zang, K. L. Wang, W. J. Yu, H. Jin, L. Wang and H. W. Zhu, Nano Res., 2015, 8, 1627–1636 CrossRef CAS.
- X. W. Wang, Y. Gu, Z. P. Xiong, Z. Cui and T. Zhang, Adv. Mater., 2014, 26, 1336–1342 CrossRef CAS PubMed.
- Y. Khan, A. E. Ostfeld, C. M. Lochner, A. Pierre and A. C. Arias, Adv. Mater., 2016, 28, 4373–4395 CrossRef CAS PubMed.
- W. Karlen, H. Gan, M. Chiu, D. Dunsmuir, G. H. Zhou, G. A. Dumont and J. M. Ansermino, PLoS One, 2014, 9, e99266 Search PubMed.
- J. McAviney, D. Schulz, R. Bock, D. E. Harrison and B. Holland, J. Manip. Physiol. Ther., 2005, 28, 187–193 CrossRef PubMed.
- T. Toda, M. Nakai and X. X. Liu, IEEE Ind. Elec., 2015, 3347–3352 Search PubMed.
-
A. Murata, T. Koriyama and T. Hayami, 2012 Proceedings of Sice Annual Conference (Sice), 2012, 274–279.
- J. J. Park, W. J. Hyun, S. C. Mun, Y. T. Park and O. O. Park, ACS Appl. Mater. Interfaces, 2015, 7, 6317–6324 CAS.
- Y. Pang, H. Tian, L. Q. Tao, Y. X. Li, X. F. Wang, N. Q. Deng, Y. Yang and T. L. Ren, ACS Appl. Mater. Interfaces, 2016, 8, 26458–26462 CAS.
- R. Xu, H. Zhang, Y. Cai, J. Ruan, K. Qu, E. Liu, X. Ni, M. Lu and X. Dong, Appl. Phys. Lett., 2017, 111, 103501 CrossRef.
- S. Y. Wu, R. B. Ladani, J. Zhang, K. Ghorbani, X. H. Zhang, A. P. Mouritz, A. J. Kinloch and C. H. Wang, ACS Appl. Mater. Interfaces, 2016, 8, 24853–24861 CAS.
- Y. Y. Qin, Q. Y. Peng, Y. J. Ding, Z. S. Lin, C. H. Wang, Y. Li, J. J. Li, Y. Yuan, X. D. He and Y. B. Li, ACS Nano, 2015, 9, 8933–8941 CrossRef CAS PubMed.
- S. Mulroy, J. Gronley, W. Weiss, C. Newsam and J. Perry, Gait Posture, 2003, 18, 114–125 CrossRef PubMed.
-
M. Chen, J. Y. Yan and Y. S. Xu, 2009 Ieee-Rsj International Conference on Intelligent Robots and Systems, 2009, 833–839, DOI: 10.1109/Iros.2009.5354111.
- M. J. Deen, Pers Ubiquit Comput., 2015, 19, 573–599 CrossRef.
- P. Pierleoni, A. Belli, L. Palma, M. Pellegrini, L. Pernini and S. Valenti, IEEE Sens. J., 2015, 15, 4544–4553 CrossRef CAS.
- S. Abbate, M. Avvenuti, F. Bonatesta, G. Cola, P. Corsini and A. Vecchio, Pervasive Mob. Comput., 2012, 8, 883–899 CrossRef.
- A. Hakim, M. S. Huq, S. Shanta and B. S. K. K. Ibrahim, Procedia Comput. Sci., 2017, 105, 46–51 CrossRef.
- J. J. Jia, X. Y. Sun, X. Y. Lin, X. Shen, Y. W. Mai and J. K. Kim, ACS Nano, 2014, 8, 5774–5783 CrossRef CAS PubMed.
Footnotes |
† Electronic supplementary information (ESI) available. See DOI: 10.1039/c7nh00147a |
‡ These authors contributed equally to this work. |
|
This journal is © The Royal Society of Chemistry 2018 |