DOI:
10.1039/C7FO01149K
(Paper)
Food Funct., 2018,
9, 171-178
Ginger fermented with Schizosaccharomyces pombe alleviates memory impairment via protecting hippocampal neuronal cells in amyloid beta1–42 plaque injected mice
Received
28th July 2017
, Accepted 12th November 2017
First published on 13th November 2017
Abstract
Ginger, which has been widely used for dietary condiment, has been reported to improve memory dysfunction in an animal model of Alzheimer's disease (AD). Recently, a few trials have been carried out to enhance the effects of ginger by improving the bioavailability of its relevant components via fermentation. Some reports have suggested that the fermented ginger has the ability to affect the AD in vitro systems; however, its anti-amnesic effects on an in vivo model still remain to be investigated. In the present study, we aimed to investigate the neuroprotective effects of ginger fermented with Schizosaccharomyces pombe (FG) in the in vivo models of AD. The neuroprotective effects were investigated by employing behavioral, western blotting, and immunohistochemical assays. The administration of FG improved recognition memory, impaired by scopolamine injection, than that of non-fermented ginger. In addition, FG ameliorated memory impairment in amyloid beta1–42 (Aβ1–42) plaque-injected mice via protecting neuronal cells in the CA3 area of the mouse hippocampus. Moreover, FG reinstated the pre- and postsynaptic protein levels decreased by Aβ1–42 plaque-toxicity. Overall, these data suggest that FG attenuates memory impairment in Aβ1–42 plaque-induced AD mice through inhibition of neuronal cell loss and synaptic disruption.
1. Introduction
Alzheimer's disease (AD) is one of the most common neurodegenerative disorders inducing cognitive dysfunction in old age.1 Multiple pathological conditions, such as extracellular senile plaques composed of amyloid beta (Aβ) deposits, intracellular neurofibrillary tangles, and cerebral atrophy, of AD trigger various upstream and downstream pathological pathways such as neuroinflammation, mitochondrial dysfunction, apoptosis, and oxidative stress. Thus, the development of strategies for the prevention and treatment of AD requires a multi-targeted approach.2 Recently, combination or complementary therapies have attracted significant scientific interest owing to their multimodal effects.3 Administration of functional foods, including natural products, is regarded as a complementary treatment. Recent evidence suggests that functional foods improve AD symptoms.2,4 For example, Ginkgo biloba and huperzine A, the most famous dietary supplements of AD, have been shown to improve cognitive function by inhibiting inflammation, oxidative stress, neuronal cell death, and cholinergic signal loss.5,6 Therefore, functional foods are highly potent candidates for the development of an effective treatment of AD.
Ginger (rhizome of Zingiber officinale Roscoe), one of the most popular functional foods, requires a hot and humid climate for cultivation and has been a popular cooking ingredient since ancient times because of its pungent taste. Ginger is well-known for its antioxidant, anti-inflammatory, anticancer, anti-hyperglycemic, and immunomodulatory properties.7 Recently, mounting evidence suggests that ginger positively affects memory function.8 Ginger has been shown to ameliorate scopolamine-induced memory deficits by up-regulating the expression of nerve growth factor in the mouse hippocampus9 and improve memory impairment, caused by focal cerebral ischemia or 3,4-methylenedioxymethamphetamine toxicity by virtue of its antioxidant and anti-apoptotic effects.10,11 Further studies conducted on rats reported that 5-week administration of ginger at a dose of 4 g kg−1 day−1 protected neuronal cells from Aβ-protein-induced damage due to the anti-inflammatory properties of ginger.12 Clinically, middle-aged healthy women treated with ginger for 2 months exhibited an enhanced working memory.13 In addition, strategies to increase the substance bioavailability of ginger using food-combination or processing techniques are currently under investigation.14
Fermentation is a food-processing technique that uses microbes, such as bacteria, yeast, and molds, to modify food and produce an eatable product with the desired properties.15,16 The major roles of fermentation include increasing substance bioavailability in functional foods and prolonging the shelf-life.17 Moreover, previous reports have suggested that fermentation enhances the effects of functional foods used in the treatment of AD. For example, black carrot fermented with Lactobacillus plantarum or Aspergillus oryzae contained more anthocyanin in its non-glycated form than unfermented black carrot and improved cognitive dysfunction in rat with type 2 diabetes and dementia by promoting hippocampal insulin signalling.18 Another study showed that Monascus purpureus-fermented products (monascin and ankaflavin) inhibited the accumulation of Aβ by suppressing AD risk factors in the hippocampus of Aβ protein-injected rats receiving a high-fat diet.19
Herein, we aimed to investigate the effects of ginger fermented with Schizosaccharomyces pombe (S. pombe) on AD. Several reports revealed that S. pombe, a type of the non-Saccharomyces wild yeasts, influenced on a compound by reducing biogenic amines and ethyl carbamate precursors and increasing anthocyanin in red wine.20–22 Previously, we established the condition for high stability of 6-paradol biotransformation using S. pombe and identified the possibility for the neuroprotective effect of the optimized fermented ginger with S. pombe on the AD in vitro systems.23 In the present study, we compared the anti-amnesic effects of non-fermented ginger (NFG) and optimized fermented ginger (FG) on scopolamine-injected mice. We then proceeded to investigate the protective effect of FG on Aβ1–42 plaque-injected mice. To investigate the effect of FG on memory impairment induced by Aβ1–42 plaques, we performed behavioral assays. To explore whether FG attenuated Aβ1–42 plaque-induced hippocampal cell damage and synaptic loss, we conducted Western blots and immunohistochemical assays.
2. Materials and methods
2.1. Materials
TEMED, a protein assay, Tween 20, ammonium persulfate, acrylamide, ECL reagent, and skim milk were purchased from Bio-Rad Lab. (Hercules, CA, USA). Mouse anti-β-actin antibody was purchased from Santa Cruz Biotechnology, Inc. (Santa Cruz, CA, USA). Mouse monoclonal anti-synaptophysin (SYN), L-glutamine, dimethyl sulfoxide (DMSO), paraformaldehyde (PFA), 3,3-diaminobenzidine (DAB), phosphate buffered saline (PBS), phosphate buffer (PB), tris-buffered saline (TBS), sodium dodecyl sulfate (SDS), glycine, trizma base, and donepezil hydrochloride (DN) were purchased from Sigma-Aldrich (St Louis, MO, USA). Rabbit polyclonal anti-post-synaptic density-95 (PSD95) antibody was purchased from Abcam (Cambridge, MA, USA). Anti-rabbit horseradish peroxidase (HRP) secondary antibody was purchased from Assay Designs (Ann Arbor, MI, USA). Biotinylated goat anti-mouse antibody, normal goat serum (NGS), and avidin–biotin complex were purchased from Vector Laboratories (Burlingame, CA, USA). The Aβ1–42 peptide was purchased from American Peptide (Sunnyvale, CA, USA).
2.2. Sample preparation
The extracts of NFG and FG were prepared according to our previous study.23 In brief, for preparing NFG, ginger was heated at 120 °C for 4 h to increase 6-shogaol contents, followed by an extraction with 70% ethanol (1/10, w/v).
For preparing the fermented ginger, S. pombe was inoculated onto YM agar slant and cultured at 26 °C for 3 days. After a pure culture was obtained, the colony was re-inoculated into a 100 mL YE broth in an Erlenmeyer flask and shaken (120 rpm) at 26 °C for 24 h. A pre-cultured medium (10 mL) was transferred into an Erlenmeyer flask containing 90 mL of the fresh medium followed by shaking (120 rpm) for an additional 24 h. Finally, 40 mL of the cultured medium was transferred into an Erlenmeyer flask, NFG (500 μg mL−1 of 6-shogaol) was added to the cultured medium that was then cultivated for an additional period of 72 hours, and fermented gingers were dried. After cultivation was finished, fermented ginger was freeze-dried. NFG and FG were standardized by analyzing the contents of 6-shogaol and 6-paradol, respectively, followed by a reference (NFG: 100 and 0%, FG: 12.8 and 87.2%). The quantification of relative constituents of FG has been provided previously.23 According to the reference, the contents of 6-paradol in FG is 7.5 mg g−1.
2.3. Animals
Male ICR mice at 7 weeks of age, weighing 25–30 g, were purchased from the Orient Co., Ltd, a branch of Charles River Laboratories (Seoul, Korea). The animals were allowed access to water and food ad libitum and maintained at a constant temperature (23 ± 1 °C) and humidity (60 ± 10%) under a 12 h light/dark cycle (light at 08:00–20:00 h). The animals were maintained under the laboratory conditions for an acclimatization period of 7 days before the experiments were performed. All experiments with mice were performed according to the protocols approved by the Institutional Animal Care and Use Committee of Kyung Hee University (KHP-2014-09-01).
2.4. Drug administration and surgery procedure
For optimizing the condition of fermentation, the treatments which were dissolved in 10 % DMSO were given 1 h before the acquisition trial in the animal behavior tests. Scopolamine dissolved in saline was injected at 1.1 mg kg−1 30 min before the acquisition trial in the animal behavior test. Mice were randomly divided into 5 groups (n = 10 per each group) as follows: (1) the control group (intraperitoneally saline injected plus intraorally 10% DMSO-treated group), (2) the scopolamine group (intraperitoneally scopolamine injected plus intraorally 10% DMSO-treated group), (3) the scopolamine + DN 2 mg kg−1 group (intraperitoneally scopolamine injected plus intraorally DN 2 mg kg−1-treated group), (4) the scopolamine + NFG 100 mg kg−1 group (intraperitoneally scopolamine injected plus intraorally NFG 100 mg kg−1-treated group), and (5) the scopolamine + FG 100 mg kg−1 group (intraperitoneally scopolamine injected plus intraorally FG 100 mg kg−1-treated group).
For investigating the effect of FG on the Aβ1–42 plaque-injected models, mice were immediately anesthetized and placed in a stereotaxic apparatus (myNeuroLab, St Louis, MO, USA). Each mouse was unilaterally injected (at a rate of 0.5 μl min−1) with 3 μl of Aβ1–42 plaque (1 mg ml−1) into the hippocampus (coordinates with respect to bregma in mm: AP -2.0, ML 1.5, DV 2.2) according to the stereotaxic atlas of the mouse brain.24 The sham-operated mice were injected with the same volume of distilled water alone. Treatments which were dissoveled in 19% DMSO were administered once per day for 14 consecutive days after stereotaxic injection. The mice were randomly arranged by 6 groups (n = 8 per each group): (1) the sham group (sham-operated plus intraorally 10% DMSO-treated group), (2) the Aβ1–42 plaque group (Aβ1–42 plaque-lesioned plus intraorally 10% DMSO-treated group), (3) the DN 2 mg kg−1 day−1 group (Aβ1–42 plaque-lesioned plus intraorally DN 2 mg kg−1 day−1-treated group), (4) the FG 50 mg kg−1 day−1 group (Aβ1–42 plaque-lesioned plus intraorally FG 50 mg kg−1 day−1-treated group), (5) the FG 100 mg kg−1 day−1 group (Aβ1–42 plaque-lesioned plus intraorally FG 100 mg kg−1 day−1-treated group), and (6) the FG 200 mg kg−1 day−1 group (Aβ1–42 plaque-lesioned plus intraorally FG 200 mg kg−1 day−1-treated group).
2.5. Novel object recognition test and Y-maze task
NORT was carried out in a black open-field box (45 cm × 45 cm × 50 cm) as previously described.25 All animals had an adaptation period of 5 min without objects. After adaptation, the animals were placed in the box with two identical objects and permitted to search for 3 min. The time spent by the mice exploring each object was determined (defined as the acquisition trial). After 24 hours, the animals were permitted to explore the objects, including the original object used in the training session and a novel object, for 3 min. The time spent by the mice exploring the novel and the familiar objects was measured (defined as the test trial). The results were expressed as the novel object recognition index
[% time percentage = tnovel/(tnovel + tfamiliar) × 100]. |
The Y-maze task apparatus was composed of three arms with equal angles from each other (40 cm long and 3 cm wide with 12 cm high walls), as previously described.25 Each arm has a sequence designated as A, B, and C, and mice were placed within any arm. The numbers of arm entries was determined manually for each mouse over an 8 min period. An actual alternation was defined as the entries into all three arms on consecutive choices such as ABC, CAB, or BCA. The result was expressed as the percentage of alternation identified by the following equation:
% Alternation = [(number of alternations)/(total arm entries − 2)] × 100. |
2.6. Brain tissue preparation
On the day after the last sample treatment, the mice were perfused transcardially with 0.05 M PBS and then fixed with a cold 4% PFA in a 0.1 M phosphate buffer. The brains were removed and post-fixed in 0.1 M PB containing 4% paraformaldehyde overnight at 4 °C and then immersed in a solution containing 30% sucrose in 0.05 M PBS for cryoprotection. Serial 30 μm-thick coronal sections were cut on a freezing microtome (Leica, Nussloch, Germany) and stored in cryoprotectant (25% ethylene glycol, 25% glycerol, and 0.05 M PB) at 4 °C until use. For Western blot analysis, the mice were decapitated, and the brains were isolated, washed with PBS, and stored at −80 °C until use.
2.7. Western blot analysis
The hippocampal tissues were lysed using a protein assay according to the manufacturer's instructions. The lysates were separated by 10% SDS-polyacrylamide gel electrophoresis and then transferred to a membrane. The membranes were incubated with 5% skim milk in TBST for 1 h and then with the primary antibody (1
:
500 dilution of PSD95 and 1
:
2000 β-actin) overnight at 4 °C. This was followed by incubation with an HRP-conjugated secondary antibody for 1 h. The immunoreactive bands were detected using an ECL detection kit, and an LAS-4000 mini system (Fujifilm, Tokyo, Japan) was used for visualization. The intensities of the bands were normalized to the β-actin intensity using the Multi Gauge software (Fujifilm).
2.8. Immunohistochemistry
For an immunohistochemical study, the brain sections were briefly rinsed in PBS and treated with 1% hydrogen peroxide for 15 min. The sections were incubated with a mouse anti-NeuN antibody (1
:
1000 dilution) and a mouse anti-SYN (1
:
200 dilution) overnight at 4 °C in the presence of 0.3% Triton X-100 and NGS. After rinsing in the PBS buffer, the sections were incubated with biotinylated anti-mouse IgG (1
:
200 dilution) for 90 min and with ABC (1
:
100 dilution) for 1 h at room temperature. Peroxidase activity was visualized by incubating the sections with DAB in 0.05 M tris-buffered saline (pH 7.6). After several rinses with PBS, the sections were mounted on gelatin-coated slides, dehydrated, and cover-slipped using a histomount medium. The optical density of SYN-immunoreactivities in the CA3 of hippocampus region was analyzed using the ImageJ software (Bethesda, MD, USA). The total region of interest was manually outlined, and the averaged optical densities were acquired from the images with converted eight-bit indexed color. The images were obtained at 200× magnification using an optical light microscope (Olympus Microscope System BX51, Olympus, Tokyo, Japan) equipped with a 20× objective lens. The data are presented as percentages of the sham group values.
2.9. Statistical analysis
All statistical parameters were calculated using GraphPad Prism 5.0 software (San Diego, CA). The values were expressed as the mean ± standard error of the mean (SEM). The results from this study were analyzed by one-way analysis of variance followed by Tukey's post-hoc test. The differences with a p-value less than 0.05 were considered statistically significant.
3. Results and discussion
3.1. Comparison between anti-dementia effects of NFG and FG in scopolamine-injected mice
The factors such as yeast strain, pH value, temperature, nutrients, humidity, and time are crucial for the fermentation of herbs.26 Among these parameters, fermentation time is a key to improve the functional effects by increasing the by-products of fermentation.27,28 It has been reported that ginger has an effect on various dementia models.9,10,13 Previously, we established the optimized time condition of fermented ginger to improve its memory enhancing effect. It has been reported that the contents of metabolites of 6-shogaol called 6-paradol in fermented ginger proportionally increased with an increase in the fermentation time. We optimized the fermentation condition with the contents of 6-paradol and found that 6-paradol-enriched ginger with fermentation showed an increase in the neuroprotective effect against Aβ1–42 plaque and oligomer toxicity in rat primary hippocampal cells as compared to 6-shogaol-enriched ginger without fermentation.23
In the present study, we utilized the optimal fermentation condition for ginger with S. pombe based on the previous study and compared its effect with those of NFG on memory function in scopolamine-injected mice. Memory function was evaluated using NORT and Y-maze tasks. In NORT, while scopolamine-treated mice spent a similar amount of time exploring the novel object and the familiar object (50.51 ± 2.58%), NFG- and FG-treated mice spent more time exploring the novel object than that for exploring the familiar object (57.63 ± 1.40 and 59.66 ± 3.01%, respectively). The anti-amnesic effect on the FG-treated mice was the exceeding effect of DN treatments (DN was used as a positive control, Fig. 1A). In the Y-maze task, the proportion of spontaneous alternations (%) significantly decreased in the scopolamine-treated mice (48.25 ± 3.14%) as compared to that in the vehicle-treated mice (63.09 ± 3.51%). By contrast, the proportion of spontaneous alternations significantly increased in the NFG and FG-treated mice (57.97 ± 3.38% and 58.93 ± 3.77%, respectively) as compared to that in the scopolamine-treated mice (Fig. 1B). The results of both NORT and Y-maze test showed that FG-treated mice slightly improved memory function more than the NFG-treated mice. On the basis of these data, we demonstrated that fermentation increased the anti-amnesic effect and proceeded to investigate the effects of FG on our Aβ1–42 plaque-injected model in a series of further experiments.
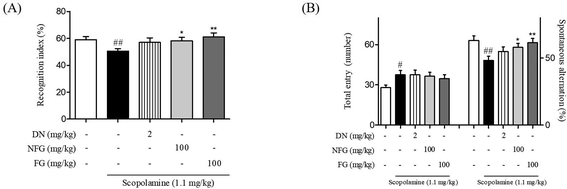 |
| Fig. 1 Effects of fermented gingers on scopolamine-induced cognitive deficits as determined by NORT and Y-maze test. Mice were treated with FG, NFG, and DN at the concentration of 100 mg kg−1 and 2 mg kg−1. Bar indicates the percentage of recognition index during a test trial (A). Bar indicates the percentage of spontaneous alternation (B). The values are the mean ± S.E.M. #p < 0.05 and ##p < 0.01 as compared to the vehicle-treated control group. *p < 0.05 and **p < 0.01 as compared to the scopolamine- and vehicle-treated group. DN: donepezil, NFG: non-fermented ginger, and FG: fermented ginger by S. pombe. | |
3.2. Effects of FG on memory impairment in an Aβ1–42 plaque-induced AD-like model
Aggregated Aβ, an important pathological factor in AD, induces neuronal cell damage, inhibits synaptic function, and ultimately results in memory impairment.29 Therefore, Aβ has been routinely used to provide in vivo models of AD. Among the Aβ plaques, Aβ1–42 is the initial and predominant constituent of amyloid plaques and exhibits a higher toxicity and aggregation propensity than other types of Aβ plaques.30,31 To explore the neuroprotective effects of FG, we developed an AD model by stereotaxic injection of the Aβ1–42 plaque into the mouse hippocampus.
At first, we performed two kinds of behavioral tests after the FG treatment to investigate whether FG alleviated memory impairment induced by the Aβ1–42 plaque toxicity. In NORT, the Aβ1–42 plaque-injected mice spent a smaller percentage of time exploring the novel object than that for the familiar object (46.77 ± 2.91%) as compared to the sham-operated mice (66.75 ± 2.75%). By contrast, mice treated with FG at a dose of 200 mg kg−1 day−1 for 14 days significantly increased the percentage of time spent on exploring the novel object; this indicated the improved memory functions (66.02 ± 3.59%, Fig. 2A). While NORT is commonly used to evaluate the onset of the hippocampus-dependent memory formation, particularly for recognition memory,32 the Y-maze test is often used to evaluate the role of the hippocampus in spatial memory.33 In the Y-maze test, the spontaneous alternation (%) was significantly reduced in the Aβ1–42 plaque-injected mice (61.91 ± 1.51%) as compared to that in the sham-operated mice (71.55 ± 2.14%). However, the treatment with FG at the doses of 100 and 200 mg kg−1 day−1 for 14 days significantly attenuated the effects of Aβ1–42 plaque-injection (68.40 ± 1.85 and 67.04 ± 1.18%, respectively, Fig. 2B). Overall, our behavioral assays revealed that the FG treatment attenuated Aβ1–42 plaque-induced memory impairment by promoting recognition and spatial memory. Next, we investigated whether FG protected hippocampal neurons against Aβ1–42 plaque neurotoxicity.
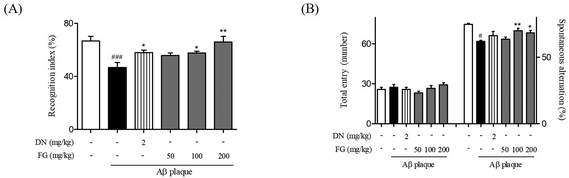 |
| Fig. 2 Protective effect of FG against Aβ1–42 plaque-induced cognitive dysfunction in the AD-like model. The time of exploring the novel object (A) and spontaneous alternation index (B) were recorded. The values are indicated as the mean ± SEM. #p < 0.05 and ###p < 0.001 compared with the sham group. *p < 0.05 and **p < 0.01 compared with the Aβ-only treated group. | |
3.3. Protective effect of FG on hippocampal neuronal cell damage induced by Aβ1–42 plaque
Neural atrophy, the representative characteristic of AD brain tissue, is thought to be crucially related to memory impairment under AD conditions. Moreover, Aβ plaque-injection into rodent brains has been shown to result in neuronal cell loss.34 The hippocampus plays an important role in mediating declarative memory. In particular, the hippocampal CA3 region plays an important role in encoding new spatial information in short-term memory.35 To estimate the degree of the neuronal damage in Aβ1–42 plaque-injected mice, we measured the immunoreactivity of NeuN neuronal marker in the hippocampal CA3 region. Neuronal cell loss in the CA3 region of the Aβ1–42 plaque-injected mice was significantly larger than that in the sham-operated mice (Fig. 3). Neuronal cell loss was reduced when FG was concomitantly administrated at the dose of 50, 100, and 200 mg kg−1 day−1 for 14 days. In summary, our experiments revealed that the FG treatment alleviated memory dysfunction induced by the Aβ1–42 plaque toxicity. Improved memory function was mediated by FG neuroprotective properties inhibiting Aβ1–42 plaque-induced neuronal cell loss in the CA3 region of the hippocampus.
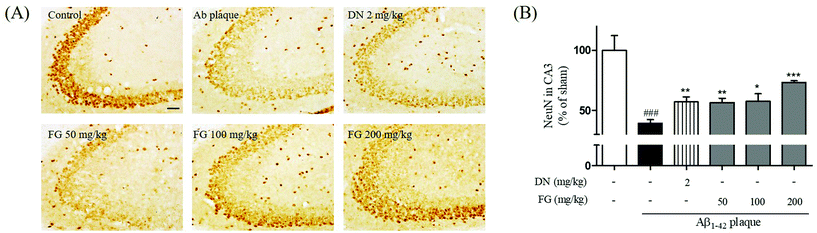 |
| Fig. 3 Protective effects of FG on neuronal cells damages by Aβ1–42 plaque in the hippocampal CA3 region. The NeuN immunoreactivity was detected in the ipsilateral region (A) in the CA3 of the hippocampus and the representative images of experiments are shown in sequence (B). The values are expressed as the mean ± S.E.M. Scale bar = 50 μm. ###p < 0.001 as compared to the control group. *p < 0.05, **p < 0.01 and ***p < 0.001 as compared with the Aβ-injected group. | |
3.4. Inhibitory effect of FG on synaptic disruption induced by Aβ1–42 plaque
Synaptic plasticity is the representative feature of hippocampal synapses and underlies hippocampus-dependent memory.36 The Aβ1–42 plaques have been shown to disrupt the synaptic function in AD brain tissue and result in memory impairment.37 SYN is located in the membrane of the pre-synaptic vesicles, and PSD95 is located in the postsynaptic density of asymmetric synapses. Both have been used as markers for the synaptic density.38 To explore the improvement of the synaptic function by FG, we performed Western blot analysis and immunohistochemistry to assess the expression levels of the SYN and PSD95 proteins in the hippocampus. The treatment of mice with FG at the doses of 50, 100, and 200 mg kg−1 day−1 for 14 days significantly increased the expression levels of SYN in the CA3 region as compared to the case of Aβ1–42 plaque-injected mice that did not receive FG (Fig. 4). The FG treatment at 200 mg kg−1 day−1 for 14 days also up-regulated the expression of PSD95 as compared to the case of Aβ1–42 plaque-injected mice that did not receive FG (Fig. 5).
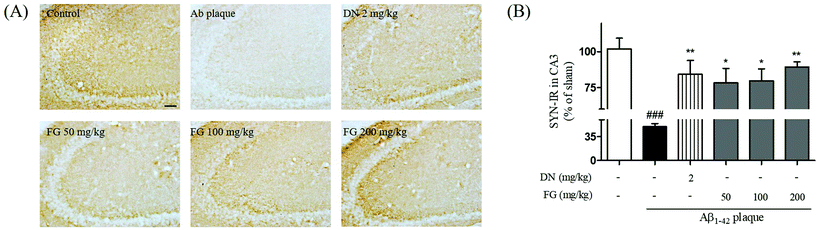 |
| Fig. 4 Protective effect of FG against Aβ1–42 plaque-induced decrease of pre-synaptic activity in the CA3 of the mouse hippocampus. The ipsilateral optical density of SYN-immunoreactivity in the CA3 of the hippocampus was measured (A) and the representative images of experiments are shown (B). Scale bar = 50 μm. The values are indicated as the mean ± SEM. ###p < 0.001 compared with the sham group. *p < 0.05 and **p < 0.01 compared with the Aβ-only treated group. | |
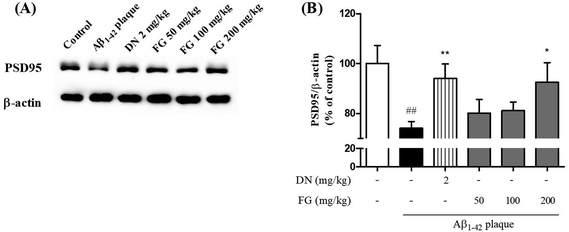 |
| Fig. 5 Protective effect of FG against Aβ1–42-induced the disruption of post-synaptic activity in the hippocampus in the AD-like model. (A) PSD95 expressions were assessed using Western blotting with antibodies against PSD95 in hippocampus. (B) The graph displays densitometric analyses of the expression ratio of the cytosolic PSD95/β-actin. The values are indicated as the mean ± SEM. ##p < 0.01 as compared to the control group. *p < 0.05 and **p < 0.01 as compared with the Aβ-injected group. | |
4. Conclusions
In the present study, we showed the anti-amnesic effects of the optimized fermented ginger in the scopolamine-injected mice and compared these effects with those of NFG. Then, we proceeded to show that FG attenuated the recognition and spatial memory dysfunction in the Aβ1–42 plaque-injected mice. Using immunohistochemistry, we demonstrated that FG inhibited neuronal cell loss and synapse disruption in the mouse hippocampus. We have speculated that the anti-amnesic effects of FG originate from the fermentation process because 6-paradol, a metabolite of the fermentation process, has previously been shown to possess neuroprotective properties in neurodegenerative diseases.39 Recently, due to the high bioactivity of 6-paradol, 6-paradol has attracted attention as a potential alternative candidate for the treatment of CNS disorders.40–42 Moreover, the fermented ginger extract, abundant in 6-paradol, showed the neuroprotective properties, counteracting the effects of the Aβ plaque toxicity in rat hippocampal cells.23,43 Overall, fermentation of ginger by S. pombe induced a change in the bioactive compounds of ginger, namely converting 6-shogaol to 6-paradol. The effects of FG reported in this study might be related to the by-product of the fermentation process. However, further study is needed to fully elucidate the mechanism of the action of FG in counteracting the effects of the Aβ1–42 plaque toxicity.
In summary, we demonstrated the anti-amnesic effects of FG against the Aβ1–42 plaque toxicity by inhibiting neuronal cell loss and synaptic disturbance. For further study, we speculated that effects of FG were derived from the metabolites of the fermentation process, 6-paradol, as a potential candidate mediating memory improvement. We suggest that fermented ginger by S. pombe alleviates AD-like memory dysfunction and neuronal degradation.
Abbreviations
Aβ | Amyloid beta |
AD | Alzheimer's disease |
DAB | 3,3-Diaminobenzidine |
DN | Donepezil hydrochloride |
DMSO | Dimethyl sulfoxide |
FG | Ginger fermented with Schizosaccharomyces pombe |
HPLC | High-performance liquid chromatography |
HRP | Horseradish peroxidase |
NFG | Non-fermented ginger |
NGS | Normal goat serum |
NORT | Novel object recognition test |
PB | Phosphate buffer |
PBS | Phosphate buffered saline |
PFA | Paraformaldehyde |
PSD95 | Post-synaptic density-95 |
SDS | Sodium dodecyl sulfate |
SEM | Standard error of the mean |
S. pombe
|
Schizosaccharomyces pombe
|
SYN | Synaptophysin |
TBS | Tris-buffered saline. |
Funding
This work was supported by the Bio-industry Technology Development Program, Ministry of Agriculture, Food and Rural Affairs (112136-4).
Conflicts of interest
The authors declare that there are no conflicts of interest.
References
- H. G. Kim, S. Lim, J. Hong, A. J. Kim and M. S. Oh, Phytother. Res., 2016, 30, 208–213 CrossRef CAS PubMed.
- H. G. Kim and M. S. Oh, Curr. Pharm. Des., 2012, 18, 57–75 CrossRef CAS PubMed.
- S. J. Zhang and X. Tian, J. Alzheimers Dis. Parkinsonism, 2016, 6, 216 Search PubMed.
- Y. Yang, Z. Zhang, S. Li, X. Ye, X. Li and K. He, Fitoterapia, 2014, 92, 133–147 CrossRef CAS PubMed.
- P. L. Le Bars, F. M. Velasco, J. M. Ferguson, E. C. Dessain, M. Kieser and R. Hoerr, Neuropsychobiology, 2002, 45, 19–26 CrossRef CAS PubMed.
- S. H. Xing, C. X. Zhu, R. Zhang and L. An, Evid. Based Complement Alternat. Med., 2014, 2014, 363985 Search PubMed.
- K. L. Nagendra chari, D. Manasa, P. Srinivas and H. B. Sowbhagya, Food Chem., 2013, 139, 509–514 CrossRef CAS PubMed.
- H. M. Hugel, Adv. Exp. Med. Biol., 2015, 863, 95–116 CrossRef CAS PubMed.
- S. Lim, M. Moon, H. Oh, H. G. Kim, S. Y. Kim and M. S. Oh, J. Nutr. Biochem., 2014, 25, 1058–1065 CrossRef CAS PubMed.
- J. Wattanathorn, J. Jittiwat, T. Tongun, S. Muchimapura and K. Ingkaninan, Evid. Based Complement Alternat. Med., 2011, 2011, 429505 Search PubMed.
- M. Mehdizadeh, F. Dabaghian, A. Nejhadi, H. Fallah-Huseini, S. Choopani, N. Shekarriz, N. Molavi, A. Basirat, F. Mohammadzadeh Kazorgah, A. Samzadeh-Kermani and S. Soleimani Asl, Cell J., 2012, 14, 177–184 Search PubMed.
- G. F. Zeng, Z. Y. Zhang, L. Lu, D. Q. Xiao, S. H. Zong and J. M. He, Rejuvenation Res., 2013, 16, 124–133 CrossRef PubMed.
- N. Saenghong, J. Wattanathorn, S. Muchimapura, T. Tongun, N. Piyavhatkul, C. Banchonglikitkul and T. Kajsongkram, Evid. Based Complement Alternat. Med., 2012, 2012, 383062 Search PubMed.
- S. Lim, J. G. Choi, M. Moon, H. G. Kim, W. Lee, H. R. Bak, H. Sung, C. H. Park, S. Y. Kim and M. S. Oh, J. Alzheimers Dis., 2016, 50, 189–200 CrossRef CAS PubMed.
- A. F. Ismail and S. M. El-Sonbaty, J. Photochem. Photobiol., B, 2016, 158, 154–163 CrossRef CAS PubMed.
- E. J. Yang, S. I. Kim, S. Y. Park, H. Y. Bang, J. H. Jeong, J. H. So, I. K. Rhee and K. S. Song, Food Chem. Toxicol., 2012, 50, 2042–2048 CrossRef CAS PubMed.
- E. C. Borresen, A. J. Henderson, A. Kumar, T. L. Weir and E. P. Ryan, Recent Pat. Food, Nutr. Agric., 2012, 4, 134–140 CrossRef CAS.
- S. K. S. Park, D. Y. Jeong, S. Y. Jeong and M. J. Kim, J. Funct. Foods, 2016, 25, 354–366 CrossRef CAS.
- C. L. Lee, P. Y. Lin, Y. W. Hsu and T. M. Pan, J. Funct. Foods, 2015, 18, 387–399 CrossRef CAS.
- A. Benito, F. Calderon, F. Palomero and S. Benito, Molecules, 2015, 20, 9510–9523 CrossRef CAS PubMed.
- A. Benito, D. Jeffares, F. Palomero, F. Calderon, F. Y. Bai, J. Bahler and S. Benito, PLoS One, 2016, 11, e0151102 Search PubMed.
- A. Benito, F. Calderon and S. Benito, Molecules, 2017, 22, 739 CrossRef PubMed.
- J. W. Choi, H. Y. Park, M. S. Oh, H. H. Yoo, S. H. Lee and S. K. Ha, J. Funct. Foods, 2017, 31, 304–310 CrossRef CAS.
-
G. Paxinos and K. Franklin, The mouse brain in stereotaxic coordinates, Gulf professional publishing, 2004 Search PubMed.
- E. Huh, H. G. Kim, H. Park, M. S. Kang, B. Lee and M. S. Oh, Biomol. Ther., 2014, 22, 176–183 CrossRef PubMed.
- C. L. Lee and T. M. Pan, Appl. Microbiol. Biotechnol., 2012, 94, 1449–1459 CrossRef CAS PubMed.
- C. T. Chang, C. K. Hsu, S. T. Chou, Y. C. Chen, F. S. Huang and Y. C. Chung, Int. J. Food Sci. Technol., 2009, 44, 799–806 CrossRef CAS.
- J. Rodriguez-Campos, H. B. Escalona-Buendia, S. M. Contreras-Ramos, I. Orozco-Avila, E. Jaramillo-Flores and E. Lugo-Cervantes, Food Chem., 2012, 132, 277–e0120203288 CrossRef CAS PubMed.
- H. G. Kim, J. Y. Kim, W. W. Whang and M. S. Oh, Can. J. Physiol. Pharmacol., 2014, 92, 429–437 CrossRef CAS PubMed.
- A. E. Roher, J. D. Lowenson, S. Clarke, A. S. Woods, R. J. Cotter, E. Gowing and M. J. Ball, Proc. Natl. Acad. Sci. U. S. A., 1993, 90, 10836–10840 CrossRef CAS.
- Y. Xiao, B. Ma, D. McElheny, S. Parthasarathy, F. Long, M. Hoshi, R. Nussinov and Y. Ishii, Nat. Struct. Mol. Biol., 2015, 22, 499–505 CAS.
- S. J. Cohen and R. W. Stackman Jr., Behav. Brain Res., 2015, 285, 105–117 CrossRef PubMed.
- T. J. Shors, G. Miesegaes, A. Beylin, M. Zhao, T. Rydel and E. Gould, Nature, 2001, 410, 372–376 CrossRef CAS PubMed.
- H. G. Kim, G. Park, S. Lim, H. Park, J. G. Choi, H. U. Jeong, M. S. Kang, M. K. Lee and M. S. Oh, J. Ethnopharmacol., 2015, 171, 196–204 CrossRef PubMed.
- R. P. Kesner, Learn. Mem., 2007, 14, 771–781 CrossRef PubMed.
- G. Neves, S. F. Cooke and T. V. Bliss, Nat. Rev. Neurosci., 2008, 9, 65–75 CrossRef CAS PubMed.
- D. J. Selkoe, Science, 2002, 298, 789–791 CrossRef CAS PubMed.
- L. A. Glantz, J. H. Gilmore, R. M. Hamer, J. A. Lieberman and L. F. Jarskog, Neuroscience, 2007, 149, 582–591 CrossRef CAS PubMed.
- B. P. Gaire, O. W. Kwon, S. H. Park, K. H. Chun, S. Y. Kim, D. Y. Shin and J. W. Choi, PLoS One, 2015, 10, e0120203 Search PubMed.
- D. S. Kim and J. Y. Kim, Bioorg. Med. Chem. Lett., 2004, 14, 1287–1289 CrossRef CAS PubMed.
- W. Y. Chung, Y. J. Jung, Y. J. Surh, S. S. Lee and K. K. Park, Mutat. Res., 2001, 496, 199–206 CAS.
- B. P. Gaire, O. W. Kwon, S. H. Park, K. H. Chun, S. Y. Kim, D. Y. Shin and J. W. Choi, PLoS One, 2015, 10, e0120203 Search PubMed.
- H. Y. Park, J. W. Choi, Y. Park, M. S. Oh and S. K. Ha, J. Funct. Foods, 2016, 21, 147–152 CrossRef CAS.
Footnote |
† These authors contributed equally to this study. |
|
This journal is © The Royal Society of Chemistry 2018 |