DOI:
10.1039/C7EN01139C
(Perspective)
Environ. Sci.: Nano, 2018,
5, 2030-2046
Proposal for a tiered dietary bioaccumulation testing strategy for engineered nanomaterials using fish
Received
29th November 2017
, Accepted 20th June 2018
First published on 2nd August 2018
Abstract
The scientific community has invested effort into standardising methodologies for the regulatory ecotoxicity testing of engineered nanomaterials (ENMs), but the practical requirements for bioaccumulation testing of ENMs have been given less attention. A strategy for a tiered approach to bioaccumulation testing of ENMs using fish is proposed, with recommendations for its implementation by regulatory agencies. The strategy recognises that testing the many shapes, sizes and chemistries of ENMs as new substances in vivo would be an unrealistic workload. The approach therefore includes grouping/read-across methods and tools to screen out ENMs of negligible/low bioaccumulation potential. The strategy proposes reductions of animal use for in vivo testing and with greater consideration of in vitro methods. The first tier uses dissolution in water or lipids and particle settling rates as environmental chemistry triggers for ‘ENMs of concern’. The first tier also involves a weight of evidence from these tests, plus using existing data sets from selected literature that meet data quality criteria for ENMs. Tier 2 involves new data generation using in silico models now being validated for ENMs, including QSARs and systems biology tools. Tier 2 also includes using existing experimental data, and an option to collect new data. These data can be on soils/sediments, microbial degradation, and bioaccumulation studies on invertebrates or fish cell lines. In tier 3, an in chemico digestibility assay simulating the gut lumen of fish is proposed to identify the bioaccessible fractions from an oral exposure to ENMs. If the digestibility assay is positive, then in vitro gut sacs from rainbow trout can be used to confirm accumulation by the gut mucosa. Only if both these tests in tier 3 are positive would the work proceed to the final in vivo test (tier 4) which is essentially the OECD TG 305 method for dietary bioaccumulation testing using fish, with some caveats and recommendations for ENMs. These include considerations of terminology, how to prepare contaminated food for dietary exposures, the additional controls and endpoints for ENMs, measuring ENMs in food and tissues to confirm the exposure, and the limitations of any subsequent calculation of the bioaccumulation potential.
Environmental significance
There is an urgent need to clarify the approaches and methodology for measuring the bioaccumulation potential of engineered nanomaterials (ENMs). A strategy for the bioaccumulation testing of ENMs using fish is proposed with recommendations for its implementation by regulatory agencies. The strategy involved a tiered approach that rationalises the workload from the many possible shapes, sizes and chemistries of ENMs. The strategy involves four tiers, starting from existing data and using grouping and read-across methods, then considers in silico, in chemico and in vitro methods to screen out ENMs so that only the hazardous materials go forward to in vivo testing.
|
Introduction
The environmental risk assessment of chemicals has traditionally provided information on three main areas of concern: the persistence of a substance in the environment, its potential for bioaccumulation, and toxicity to wildlife (or PBT,1). For engineered nanomaterials (ENMs), their behaviour, persistence and transformations in the environment,2–4 as well as ecotoxicity,5–8 have been studied. However, the bioaccumulation potential of ENMs has been given much less attention. The process of bioaccumulation for any substance in a eukaryote organism will involve a number of fundamental steps.9 These include: (i) the initial presentation of the substance to the organism and any binding (adsorption) to the exterior surfaces such as the gills/respiratory surface, skin, or gut; (ii) absorption (net uptake) from the exterior through the epithelial barriers into the blood circulation or extracellular fluid; (iii) some storage and/or retention by the internal organs; and (iv) excretion from the body. These fundamental processes also apply to ENMs (reviews, ref. 5 and 10). For example, the surface adsorption of ENMs onto epithelia (TiO2 nanoparticles onto the trout intestine,11) has been observed. The apparent net uptake of metal from exposure to metal-containing ENMs has also been documented in different organisms (e.g., Cu ENMs in trout,12 Ag nanoparticles in invertebrates,13 CdTe in algae,14 CeO2 in zebrafish embryos15). Although, how much of the metal is internalised as the nanoform is often unclear. The use of radiolabelled materials has shown also the uptake of carbon nanotubes, albeit to a limited extent.16 The excretion mechanisms for ENMs remains less well known, but excretion via the kidney is unlikely because of the small pore size of the glomerular filter, with excretion via the liver the most likely elimination route.5
The apparent bioaccumulation of a substance in an organism is a single moment in time (a ‘snap shot’) of all the above processes, and can be considered the sum of absorption, distribution, metabolism and excretion (ADME). However, while such detailed knowledge may inform on the target organs and aetiology of toxicity, a much simpler approach is needed for the routine determination of bioaccumulation potential. The original approach to measuring the bioaccumulation potential of chemicals involved exposing small fish (e.g., trout fingerlings) to the test substance via the water for about thirty days until the chemical concentrations in the external media and the fish tissues were more or less in steady state.17 This approach enabled the calculation of a bioconcentration factor (BCF) which equalled the concentration in the fish or specified tissues thereof (Cf as mg kg−1) divided by the concentration of the chemical in the surrounding water (Cw as mg L−1). The BCF is usually expressed in L kg−1.
Engineered nanomaterials might be considered ‘difficult to handle substances’ in water,18 with the problems of maintaining ENM dispersions for waterborne exposures, particle settling and dissolution. Similar to the challenges of very hydrophobic chemicals, the additions of solvents/dispersing agents along with various approaches to mixing and shaking have been considered for aquatic tests (reviewed by Handy et al.,19). However, a better approach may be to use a dietary bioaccumulation test for ENMs. Protocols for dietary bioaccumulation tests for substances that are difficult to handle in water are already established,20–22 and a standardised dietary protocol was developed by the Organisation for Economic Cooperation and Development (OECD) as an addition to the Technical Guideline (TG) for fish bioaccumulation (TG 305 (ref. 23)). Arguably, the dietary bioaccumulation method is a pragmatic solution to the problem of maintaining waterborne exposure in a long-term test with ENMs.
The proposal here is a call to action for the scientific community, and especially those involved with regulation; to develop, agree, and then validate where necessary, a bioaccumulation testing strategy for ENMs. Our proposal firmly indicates how this should be done with descriptions of methodology, and we challenge the status quo of regulatory testing to go far beyond simply modifying TG 305 for ENMs. Instead, we offer a fresh look at the overall testing strategy. Here, we recommend a new tiered approach with new initial triggers for testing that are specifically relevant to the behaviour of ENMs. We also recommend a strong emphasis on in silico through to in vitro screening tools to reduce the burden of work with so many sizes, shapes and chemical compositions of ENMs to consider. This approach also places much greater emphasis on animal welfare (3Rs), so that only selected ENMs are taken to the final tier of in vivo testing. In this latter tier we recommend modifications of TG 305 for ENMs.
Applying the bioaccumulation concept to engineered nanomaterials
The bioaccumulation concept was originally devised with solutes of ‘conventional’ chemicals in mind, not ENMs.19 Briefly, for solutes, the underlying theory for an aqueous exposure relies on achieving steady-state concentrations of the test substance between the external media and the tissues of the animal. For example, the diffuse entry of a non-polar substance would be driven by the inward concentration gradient, that inevitably dissipates, giving rise to the apparent steady-state. A simple two compartment model (i.e., the external environment, and the whole organism) can describe this outcome; where the net flux of the substance into the organism (i.e., accumulation) is the sum of the unidirectional fluxes going into (uptake) or out of (excretion) the organism. It can also be calculated from the ratio of uptake (k1) and elimination (k2) rate constants. The calculations normally require knowledge of the thermodynamic stability constants of the substance, free ion activity (actual concentration gradient) and applies the Fick equation and/or Michaelis–Menten kinetics to calculate the fluxes (see ref. 19, 23 and 24). This approach will also work for solutes added to the food. In this case, the solutes are released from the food by digestion, and the gut luminal fluid is the external compartment in the model. In contrast, particles will diffuse by Brownian motion in liquids; but the particle number concentration, the collision frequency and the energy in the dispersion, will determine if agglomerates form or not (according to DLVO theory, after Derjaguin, Landau, Verwey and Overbeek).25 Crucially particles form dynamic, not steady-state equilibria like solutes;25 and the uptake and excretion of ENMs are driven by various endocytosis-related pathways, and not by solute transporters.26 Nevertheless, despite these differences in processes, it has been shown that data from studies with ENMs can be fitted to Michaelis–Menten kinetics (e.g., uptake curves for TiO2 in Caco-2 cells,27) for uptake calculations. Thus, pragmatically, it is possible to conduct bioaccumulation-like experiments with ENMs and fit the data to uptake/elimination curves, but the reasons for the curve fits have an entirely different basis to that of solutes. For regulatory purpose, we also propose distinct terminology for bioconcentration factors from ENMs, so the user is reminded of the scientific origin and assumptions in the data (see below).
There are also some ecological justifications for a dietary approach. For example, an estuarine mesocosm study with gold nanorods showed the transfer of gold to the sediments and biofilm, then the primary producers and finally the gastro-intestinal tract of minnows; with a concentration factor of at least 100 for the latter step.28 Skjolding et al.29 showed that Daphnia magna could accumulate total zinc from ZnO nanoparticles exposures via the water, and that subsequently, zebrafish fed on the contaminated daphnids showed Zn accumulation. Crouteau et al.30 used isotopically modified ZnO particles to trace the trophic transfer from a diatom biofilm to freshwater snails grazing on the surface, and showed assimilation efficiencies that were broadly consistent with those known for trace metals. Together, these studies indicate potential for transfer of ENMs within aquatic food chains.
From a practical perspective, there is decades of research using in vivo dietary exposure studies with fish with metal-contaminated food pellets (reviews, ref. 31 and 32), and with organic chemicals (e.g., ref. 20, 21 and 33). Then more lately, the option to use a dietary exposure method for bioaccumulation potential was added in the OECD TG 305.23 There are also reports in the scientific literature of nutrition trial-like studies with ENMs on fish. These demonstrate that fish will eat food contaminated with ENMs and achieve a steady ration with a gain in body weight over several weeks (TiO2 with trout,34 CNTs and C60 with trout35); demonstrating the potential to use the dietary route for a long-term exposure. For dietary TiO2, this also included measurement of the total Ti concentrations in the internal organs.34 Clearly, from these years of experience with metals, organic chemicals and now ENMs, there is a good prospect of now deriving a robust protocol for an in vivo dietary bioaccumulation test for ENMs in fish.
Definitions and terminology for a bioaccumulation test with nanomaterials
The OECD TG 305 intends to help the reader choose either the aqueous or dietary test, depending on the practical problems of handling the test substance. The terms used to describe the accumulation factors are also different; with bioconcentration factor (BCF) and biomagnification factor (BMF) intended for the aqueous and dietary tests respectively, to further stress the differences between the two tests. ENMs are not solutes and do not move through biological membranes in the same way as metals or many organic compounds, and so for regulatory purposes there may need to be a distinction between the traditional bioaccumulation test and the terms calculated from it, as opposed to a test with ENMs. One suggestion is to prefix existing terms and definitions with “nano” (e.g., nanoBCF for an aqueous study, nanoBMF for a dietary exposure, or nBCF and nBMF respectively), so that the reader is reminded of their different origins and limitations. It would be impractical and confusing to create an entirely new nomenclature just for ENMs, but it is worth considering which of the existing terminologies in the OECD TG 305 would work for/be relevant to nanomaterials – and also if any of the definitions could be improved. For example in TG 305, bioaccumulation is defined as follows: bioaccumulation is generally referred to as a process in which the chemical concentration in an organism achieves a level that exceeds that in the respiratory medium (e.g., water for a fish or air for a mammal), the diet, or both. This definition is necessarily broad to capture all the possible ways the exposure might be conducted in one phrase, and to be accessible to many potential users from the scientific community.
The OECD TG 305 defines bioconcentration as follows: bioconcentration is the increase in concentration of the test substance in or on an organism (or specified tissues thereof) relative to the concentration of test substance in the surrounding medium. In principle, this definition can also work for an ENM test conducted via the water, with a nano prefix (e.g., nBCF). For the dietary test method, the definition of biomagnification used by TG 305 for regulatory purposes is: biomagnification is the increase in concentration of the test substance in or on an organism (or specified tissues thereof) relative to the concentration of test substance in the food. This definition could also be applied to ENMs (e.g., nBMF) in the regulatory context. Risk assessors may also find BCF or BMF values for soluble chemicals in the scientific literature. However, the regulatory use of BMF to infer dietary bioaccumulation in a single species of fish is not consistent with that used by other scientific communities. In ecology, academics refer to ‘biomagnification’ as the increase in concentration of a substance across trophic levels in a food web, whereas ‘bioconcentration’ relates the increase in an individual organism relative to the external environment (water or food). We should be mindful that the BMF definitions used in academic research are not necessarily the same as that used in TG 305.
Sensibly, for solutes, the OECD TG 305 recognises that the test durations are long and that the organisms may not come into steady state so easily with some chemicals. It therefore offers an approach to calculate the bioconcentration factor from the uptake (k1) and elimination (k2) rate constants, with the logic being that bioconcentration will occur if k2 is less than k1. TG 305 terms this the kinetic bioconcentration factor (BCFK), i.e., the ratio of k1/k2. At the present time, any kinetic calculations or curve fitting with data from ENMs must be regarded as empirical observations; as a good fit to the expected first order kinetics typical of a solute transport process may be entirely fortuitous. Any BCFK should have a nano prefix to remind of the caveats in the theoretical foundations.
A tiered approach to dietary bioaccumulation testing
The regulation of an entirely new group of materials such as ENMs offers the opportunity to take a step back and consider the overall testing strategy for bioaccumulation testing, and whether or not it can be made more effective, efficient and responsive to ethical concerns (the 3Rs). The numerous potential combinations of size, shape, surface coating and chemical cores of ENMs also present a logistical challenge regarding the volume of work to test each material, or group of ENMs as a new substance (e.g., ref. 36–40). Consequently, we advocate a tiered approach to the testing strategy (Fig. 1), which is described below.
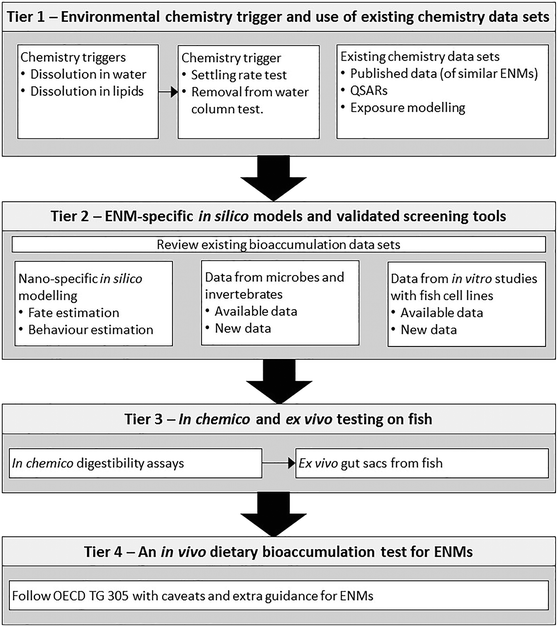 |
| Fig. 1 A tiered approach to the dietary bioaccumulation testing strategy. The first tier uses dissolution in water or in lipids as an environmental chemistry trigger(s) for concern, but where these triggers do not work for a particular ENM, then an alternative settling rate test is proposed or similar measures that quantifies removal from the water column. This tier uses a weight of evidence from these tests plus existing data sets from the literature. A positive result of the trigger test and/or evidence of concern from literature will move investigations to the next tier. In tier 2 new data generation occurs using in silico models and supported by evidence from soils, microbial or invertebrate tests that might suggest a bioaccumulation potential. A likely risk from this tier will move work to tier 3 where in chemico studies are done to determine the bioaccessible fractions of ENMs in the gut lumen environment of fish. If the digestibility assay is positive, then gut sacs can be used to confirm accumulation by the gut mucosa of fish tissue. Only if both these tests are positive would the work proceed to the final in vivo test (tier 4). The latter test is essentially the OECD TG 305 dietary bioaccumulation method, with some additional caveats and guidance for ENMs. | |
Tier 1: environmental chemistry trigger and use of existing chemistry data sets
This tier is intended as the initial chemistry ‘trigger for concern’ that a bioaccumulation-style test may be needed with an ENM. The trigger for a bioaccumulation concern for traditional organic chemicals has been the use of the octanol–water partition coefficient, which determines the relative solubility of a substance in aqueous and lipid phases. The assumption is that a substance may be more likely to bioaccumulate if it is more soluble in lipid than it is in water.17 Although there are exceptions, the octanol–water partition coefficient has been used as a ‘trigger for concern’ for organic chemicals for many years. However, results from octanol–water partition studies show varied results with ENMs, depending on their surface charge and other properties; with some ENMs becoming trapped at the liquid interphase due to surface tension, invalidating the approach.41 Consequently, the regulatory use of the octanol–water partition coefficient is generally discouraged for ENMs (e.g.ref. 42) and an alternative chemical trigger is desirable.
The thinking here is to select a factor(s) from the intrinsic properties of ENMs and/or from the extrinsic environmental chemistry that might serve as a trigger for further testing. From the intrinsic properties, these could be selected from shape, size, surface charge, chemical composition and/or particle dissolution. For the extrinsic properties, the environmental chemistry driving the transformation(s) and settling behaviours of ENMs should be considered. Extrinsic factors such as water pH, ionic strength, the presence of divalent ions and organic matter will influence surface charge and the ability of particles to form agglomerates or aggregates, either with themselves, or with other natural particles.2,25,43,44 Thus, the challenge for the testing strategy is to select the most useful ‘trigger’ for these choices in the physicochemistry.
A chemistry trigger for ENMs could include the dispersion stability (e.g., based on the recently published new OECD Test Guideline 318 (ref. 45)), and ideally accompanied by a test addressing heteroagglomeration; but could also include removal rates or other measures of the residence time of the ENM in the water column. Fast removal from the water column would indicate that exposure, and thus uptake, is primarily through food webs via the sediment, biofilms and plants. The advantage of using settling rates is that it is the sum effect of several particle properties: surface chemistry/surface charge, as well as homo- and hetero-aggregation in the environmental media; and as such, it could be used to indicate the most likely route of exposure. Fig. 2 illustrates an example for TiO2. Settling can be inferred from measurements of the disappearance of Ti from the water column over time. Both in a fish tank of continuously aerated freshwater (Fig. 2A), as well as in an unstirred physiological saline relevant to the gut (Fig. 2B), the ENMs show settling. This would raise a concern for food chain exposure for TiO2. In Fig. 2C the dissolution of the TiO2 in the gut lumen is also measured by dialysis of intact particles in a fish gut saline. Only a small fraction of the TiO2 dissolved to release Ti metal. Thus, one would infer that the bioaccumulation concern is mainly from the particulate form of the TiO2 material, rather than dissolved metal. Thus we recommend a settling rate style of measurement, in combination with dissolution rates as the key chemistry triggers of concern for bioaccumulation potential. For ENMs that also dissolve in water and/or lipids, the dissolution rate(s) could be determined and bioaccumulation risk estimated for the dissolved fractions(s) from the octanol–water partition coefficient in the normal way.46
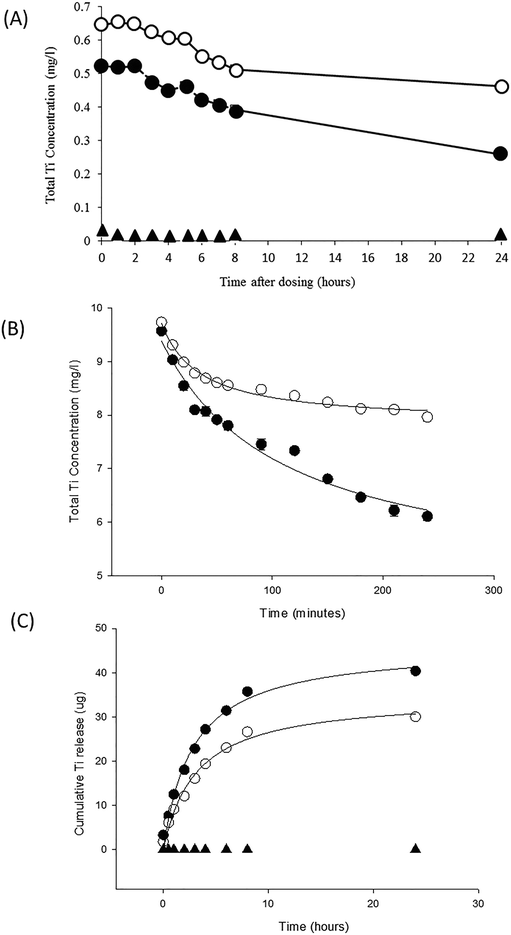 |
| Fig. 2 Chemistry triggers for an ENM of concern in tier 1 of the testing strategy, using Degussa P25 titanium dioxide nanoparticles (open circles) compared to a TiO2 bulk material of similar composition with a primary particle diameter of 147 nm (closed circle) and unexposed controls (triangles). (A) Particle settling from a 1 mg L−1 exposure measured by the decrease in total Ti concentration in the water column over time in a 20 L fish tank containing aerated Plymouth freshwater (Handy and Ramsden, unpublished observations). (B) Particle settling in unstirred Krebs physiological saline from initial 10 mg L−1 stock dispersions of each TiO2 material (Handy and Butcher unpublished observations). Note in panel A and B that the bulk material settles faster than the equivalent ENM in both freshwater and saline. (C) Dissolution curves for TiO2 materials measured by dialysis in a fish gut saline. The cumulative appearance of total Ti metal through the dialysis tubing into the external medium is shown with curves fitted to a rectangular hyperbola. The dialysis bag contained a total of 800 μg of Ti metal, thus only 5% or less of the materials dissolved. The unexposed saline was at or below the detection limit. Redrawn from Al-Jubory and Handy.11 All values are means ± S.E. of triplicate measurements. Note, the error bars are often too small to visualise. | |
Clearly, the weight of evidence for ‘concern’ should also include evaluation of the existing published data on the physicochemical properties of the ENM of concern, or similar ENMs, and their environmental chemistry. This evaluation should be done in a systematic way and minimum quality criteria should be applied before using data from published articles (see ref. 47). For example, whether or not the publication reports the characterisation in the environmental media and ultrapure water for reference, uses environmentally-relevant concentrations, whether measured or nominal values are used for the exposure, and whether appropriate bulk (micron scale) material or metal salts are included in the experimental design to enable comparisons of effects with the ENM. Reports that do not meet these requirements, along with other general measures of robustness such as replication of the experimental design, would not be used, or considered with caveats on the data limitations.
Evaluation of published data should also include attempts at grouping materials of concern and read-across approaches with the aim to reduce the burden of testing on companies producing ENMs or nano-enabled products. This is critically important for ENMs where so many combinations of (surface) chemistry, shape and size are possible. For example, there may not be data on the specific ENM of interest, but data may be available on ENMs that are structurally similar, or have similar physicochemical attributes (settling rate, propensity to show dissolution, etc.). Grouping on the basis of such similarities can increase the weight of evidence that an exposure with bioaccumulation potential could occur (problem formulation), and clarify the need for new testing to generate new data (i.e., only fill the data gaps). This would be an iterative process, where read-across from (eco)toxicological properties could (partly) inform on the level of bioaccumulation concern for a new ENM. There are already some attempts at grouping and/or read-across for ENMs (e.g., ref. 36, 48 and 49), although these are generally aimed at concerns for human health. Approaches include for example, the British Standard Institute proposal for grouping of ENMs: (https://nanohub.org/groups/gng/guidelines), or the specific guidance for the grouping of ENMs by ECHA.50
Existing data sets might also be used for predicting the bioaccumulation potential. The approaches here include QSARs and other in silico predictions. The current QSAR-style models are also tending to focus on exposure modelling or predicting toxicity (e.g., ref. 49 and 51), but models should be developed for bioaccumulation risk as well. There are some ADME models for traditional chemicals and medicines (e.g., ref. 52). These models are not yet validated for ENMs, partly because many aspects of distribution, metabolism and excretion of ENMs are not well understood.5 The existing ADME models could be modified for ENMs. For example, where an ENM is restricted to the effective circulating volume of an animal (i.e., just the blood space) by virtue of particle size, unlike solutes such as Na+ that spread rapidly into the entire extracellular fluid space, the model could be corrected for the fraction of the fluid space occupied by the ENM. Alternatively, data could be substituted from an existing organic chemical that fortuitously occupies the same proportion of blood space as the ENM (e.g., pesticides that bind to lipoproteins in the blood). Nonetheless, predictions of this kind should find utility, after considering the limitations of these ‘conventional’ solute models (see Handy et al.19 on the latter). Existing data outputs from current QSARs and related modelling could be used as part of the weight of evidence to establish concern now.
Tier 2: ENM-specific in silico models and screening tools
This is the first ‘biological’ tier in the proposed strategy and can include looking at existing published data that show the bioaccumulation potential in fish, with the ENM of concern, or similar ENMs. Quality criteria, as outlined above for chemistry, would also apply to the biological literature. This tier is focused on computational tools for ENMs and laboratory screening tests that have been modified already to work for ENMs. The objective in tier 2 includes two approaches: (i) to use nano-specific in silico fate and behaviour modelling to identify environmental compartments of concern and if a bioaccumulation exposure scenario is likely; and (ii) to use available data from soil, microbial and other tests of relevance to bioaccumulation that have been validated for ENMs. The latter can include ecotoxicity studies where the bioaccumulation-related data is ‘incidental’ to the main purpose of the experiment.
In silico
Various in silico models for ENMs are emerging.49 These include nano-specific QSARs to estimate the potential for toxicity (e.g., ref. 51, 53 and 54), and exposure modelling (e.g., ref. 55 and 56) to identify if a soil or sediment is at risk (i.e., the base of food webs). This can include models that simulate the bioaccessible fraction of ENMs in the pore water of soils or sediments. There are also some new computational tools that go beyond traditional QSARs to include biological effects in the hazard predictions.57 Some of these new approaches use systems biology to predict modes of action or biological effects (e.g., ref. 58). The latter systems biology approaches offer much greater certainty in the predictions than traditional QSARs, and can be used from the bottom up (i.e., chemical properties to predict uptake or hazard) or top down (i.e., take a biological effect and predict the causative chemical characteristics). They therefore offer a big step forward in predictive modelling. However, it should be pointed out that even after 20 years of genomics, there are no omics models accepted by the OECD. Regulatory bodies may need to be more open to the evaluation and validation of new computational tools for ENMs, although scientists may need further investments to explain and clarify how such new tools can facilitate the regulatory needs. Currently, regulatory acceptance is some way off, but such tools can inform the decision making in this early tier, with caveats of the status of validation or limitations. Such data can also be included in the background as part of the weight of evidence for a bioaccumulation risk assessment.
Data from microbes and invertebrates
There are a variety of OECD test methods from microbes and invertebrates that, although primarily intended for other areas of regulatory testing, can be useful to inform on the bioaccumulation problem. Risk assessment for bioaccumulation considers aspects such as the functional properties of soil or evidence of bioaccumulation from microbes, as well as other soil organisms. These approaches for tests that have been re-validated for ENMs can be used in tier 2. For example, the OECD already has a suite of test guidelines for bacterial testing (see Crane et al.,59). For example, the TGs 301 and 310 (ready biodegradability) may provide information on the degradation of the carbon-based ENMs or organic coating on inorganic cores of some ENMs and at least help towards understanding the persistence of ENMs at the base of food webs. Abbreviated bioaccumulation tests using earthworms or Caenorhabditis elegans might also be applied, but the intention here is to keep the tier reasonably quick and robust. A lengthy soil organism test that might delay the testing strategy or the final outcome is not desirable in the early tiers. In our example case of TiO2 where the chemistry data has already indicated a concern for bioaccumulation (Fig. 2), one might consider the values of kinetic rate constants for bioaccumulation in invertebrates,60 or ‘bioaccumulation factors’ calculated for simple food web experiments from algae to invertebrates.61 Such information may provide further circumstantial evidence of a bioaccumulation potential concern.
Data from in vitro studies with fish gill or gut cell lines
Considering the financial and time burden on industry for testing, it is not intended for new experiments on fish gill or gut cell lines to be a mandatory step of the data collection. Pragmatically, the ex vivo gut sac method in tier 3 (below) is faster and cheaper to demonstrate uptake at external epithelial than fish cell culture studies, as well as being closer to in vivo as it uses the intact intestine. Nonetheless, there is an emerging body of data on the effects of ENMs on fish cell lines that could be used as part of the weight of evidence to at least corroborate epithelial uptake. The use of existing data would need to consider quality criteria for ENMs in cell culture conditions; such as an appropriate exposure period (e.g., 48–72 h), appropriate sample preparation to enable bioaccumulation in the cells to be shown, evidence that the cells were thoroughly washed to avoid the interference of materials adsorbed to the cell surface, and the ENM content of the cells analysed by appropriate methods. For the latter, this could include the total metal or particle number concentration by single particle inductively coupled plasma mass spectrometry (spICP-MS) for metal-containing particles. Other techniques have been successfully used for determining the cellular content of organic ENMs. For instance, intracellular concentration of poly(amidoamine) dendrimers was determined in rainbow trout RTG-2 cells after exposure to these ENMs for 72 hours using liquid chromatography-hybrid quadrupole/time-of-flight mass spectrometry.62 Tiers 3 and 4 of the testing strategy (below) focus on dietary exposure, and for parity with this exposure route, it would be preferred to have data on fish gut cells. Gut epithelial cell lines are now available for some species of fish (e.g., ref. 63), but their use is relatively new to ecotoxicology and data is yet to be collected with most ENMs. As a nearest alternative for an external epithelia of fish, gill cells might also be used (e.g., rainbow trout gill cells,26,64). Fish gut, and secondly, fish gill cells are the preferred cells for collecting new data on bioaccumulation potential. It is not suggested to collect new data from fish cell lines that represent the cells from internal organs, but existing data might be considered. For example, the liver is a central compartment for the metabolism/storage of many substances, and while this role for the liver in vivo is still to be proven for most ENMs, it would be prudent to consider any existing bioaccumulation data on fish liver cells. Although so far, the reports on fish liver cells have been mainly concerned with toxicity (e.g., ref. 65).
Preference should be given to data from fish cells in keeping with the further tiers on fish tissue or live fish (below). Existing data from mammalian cells is the least preferred. Differences are expected in the accumulation rates of ENMs between mammalian and fish cells arising from the dissimilarity of temperature and the innate permeability of the cells. However, bioaccumulation has been studied on well-established mammalian gut cell lines, such as Caco-2 cells. For example, Gitrowski et al.27 demonstrated accumulation of Ti after exposure of human intestinal Caco-2 cells to different forms of TiO2 ENMs and showed that this accumulation was crystal-structure dependent and involved endocytosis mechanisms. Whether or not crystal-structure dependent uptake occurs in fish epithelial cells is yet to be confirmed. More complex culture systems, such as co-cultures of Caco-2 cells and human immune cell lines to represent inflammation of the intestine,66 are becoming available. But for simplicity, it is not proposed to use such complex systems here for what is intended as a fairly rapid tier.
Tier 3: in chemico and ex vivo testing on fish
The existing data sets on bioaccumulation, the in silico and in vitro approaches, and information on soil/sediment organisms (tier 2), may corroborate a concern and justifying proceeding to tier 3. This latter tier involves mandatory experiments to generate new data. The initial step in tier 3 is an in chemico digestibility assay that simulates the digestive processes of the fish gut. The in chemico digestion should release the test substance from the matrix of the food, so that the test substance is bioavailable to the gut lumen. Some ENMs may be resistant to the digestion protocol and can be released as an intact particle from the digestible food matrix (e.g., TiO2 particles that tolerate dilute hydrochloric acid). This released fraction of nanoparticles is then assumed to be bioavailable to the gut epithelium. Theoretically, other ENMs may partially or completely dissolve during the in chemico digestion. If the digestibility assay determines an appreciable bioavailable fraction of dissolved substances, such as metals from metal-containing ENMs, then it would be possible to exclude further nano-specific testing on the basis that the existing bioaccumulation data for the soluble chemical applies.
However, any labile and persistent nanoparticulate fraction liberated from the digested food matrix should be considered potentially bioavailable, and would justify proceeding to ex vivo gut sac preparations which can be used to measure the accumulation in the gut mucosa itself (see below). This level of detail can provide a strong justification for proceeding (or not) to in vivo experiments with fish. However, no in vitro method has yet been validated for the use in the risk assessment on bioaccumulation within the EU. Arguably, this has been due to the lack of standardised protocols offered to the regulatory community and limited validation of the applicability and usefulness of methods (see ECHA42 and67 respectively for guidance R11 and R7c). More dialogue between the broader scientific community and regulatory bodies on possible protocols is to be encouraged. Although not formally accepted for regulatory purposes, standardised methods have been used for many years in fish biology and by the animal feed industry (see below). Pragmatically, it appears simply a matter of resourcing inter-laboratory validation (ring testing) of these existing standardised methods for the purposes of regulatory protocols. This should involve the fish physiology and animal nutrition experts who use these methods routinely and commercially. Nevertheless, current regulatory guidance for REACH67 describes ways to build a weight-of-evidence and conclude on bioaccumulation potential without vertebrate testing. From an animal welfare perspective, the ethical imperative is that reasonable cause has been demonstrated by several independent techniques. Thus, a weight of evidence from tiers 1–3 would justify the need to proceed to in vivo testing. For example, if the invertebrate data shows no concern for bioaccumulation potential, and the ENM is not bioavailable as measured by the digestibility and/or gut sacs, then there would be no ethical justification to proceed to in vivo testing with fish.
In chemico digestibility assays
Several variants of the digestibility assay approach have been standardised with regulatory testing of traditional chemicals in mind. Most notably, these include the approach by Oomen et al.68 for evaluating the soil ingestion risk in the human gut, and the serial incubation approach called BARGE for human ingestion risks from metals in soils.69,70 Standardised methods agreed within the aquaculture industry are also available for determining the digestibility of nutrients in aquafeeds (e.g., ref. 71). These latter applications in fish nutrition tend to be more complex because the interest is about matching the assay as closely as possible to the digestive processes of a particular species of fish, and may even use crude enzyme mixtures extracted from the pyloric caeca of the animals (e.g., ref. 72). Nonetheless, the digestibility technique can be a realistic proxy of the gut lumen in vivo, and is often used as a first in chemico tier in the assessment of the bioavailability of a substance in food.
Here, we present a digestibility assay that is intended as a simplified, rapid, standardised method for an ENM in fish food (Fig. 3A). The approach involves a progressive step-wise extraction of the potentially bioavailable fractions in the test sample, starting with a simple salt solution for an initial extraction and then working up to more complex media involving the use of digestive enzymes. The final step is a strong acid digestion in aqua regia to dissolve all that remains of the sample (Fig. 3A). This last step enables the extractable fractions to be calculated as a percentage of the total substance present in the sample, and is especially useful for metals and metal oxides. For example, the percentages of extractable silver from NaCl, EDTA and dilute HCl steps are shown in Fig. 3B. This shows that, regardless of whether the silver is presented in the fish food as silver nitrate, Ag nanoparticles, or as an Ag2S particle; only a few percent of the total silver is released from the food pellets in each type of simple extraction. Together, at best, this is about 6% of the silver in the animal feed. This may not seem a lot, but the data should be considered in context with dissolved metals. In Fig. 3B, the Ag nanoparticles are similar to silver nitrate in their digestibility, and dissolved metal bioavailability to the gut is usually a few percent of the ingested dose.32 Thus from a digestibility view point, the nanosilver hazard is similar to that known for dissolved forms of silver. For carbon-based ENMs, a different total extraction method will be needed for example, toluene extraction in the case of C60.73 In animal nutrition, digestion apparatus have been developed to simulate the anatomy and stomach volumes of particular animals, such as the Dacon bag technique for ruminants.74 For regulatory screening, a simple apparatus is more practical and the protocol suggested (Fig. 3A) can be performed in test-tubes.
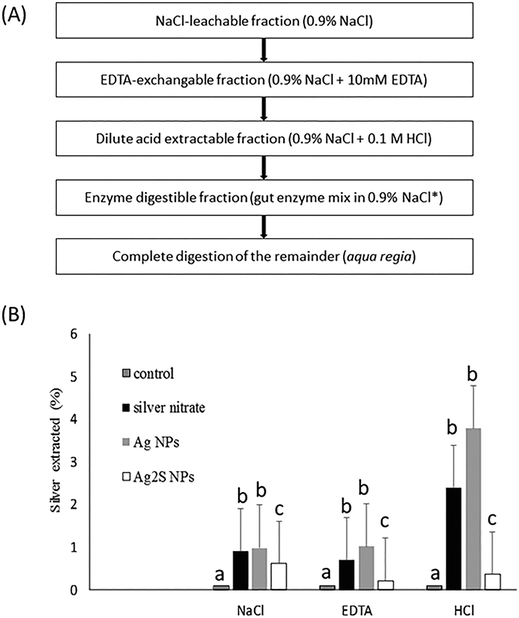 |
| Fig. 3 (A) Summary of the step-wise in chemico digestibility assay simulating digestion in fish gut. The first three steps can be done with simple shaking at room temperature for 1 hour, followed by 12 h (e.g., overnight) for the enzymatic digestion and a final strong acid digestion of the remainder. *The enzyme mix is simplified from Carter et al.71 as trypsin 0.8 mg mL−1, chymotrypsin 1.6 mg mL−1, protease 0.7 mg mL−1 at pH 8 in 0.9% NaCl. (B) Example in chemico digestibility data for 1 g samples of fish pellets loaded with Ag or Ag2S ENMs (Handy and Teape, unpublished observations from the EU Nanofase project materials). Only a few percent of the total silver are extracted by NaCl, EDTA or HCl extractions respectively, suggesting low oral bioavailability of these materials from fish food. Values are means ± S.E., n = 6 digestions each. Different letters indicate a significant difference between substances with extraction method (Kruskal Wallis or ANOVA, P < 0.05, note the controls had <0.1% extractable silver). | |
One concern is whether or not ENMs will interfere with in chemico digestibility assays.75 However, for steps such as NaCl or acid extractions, the apparent extractable fraction in each step will not be limited, provided the reagents are in excess in terms of both molarity and volume. For example, 1 g of fish food containing 100 mg kg−1 of total Ag as Ag NPs will contain a total of 100 μg of Ag (the absolute amount). In a 2 mL digestion, if all this silver dissolved (in theory), this would equate to 50 μg mL−1, or 0.46 mmol L−1 of silver. Clearly, even if the Ag precipitated with Cl− ions on an equimolar basis, as long as the NaCl concentration was in excess of 1 mmol L−1 the reagent would not be limited. Such calculations have been considered in the recipe, and for the NaCl extraction the 0.9% solution is in excess (154 mmol L−1 NaCl), but also representative of the salt concentrations in fish gut lumen.
The steps involving enzymatic digestion, in theory, may be vulnerable to enzyme inhibition by ENMs. However, digestive enzymes are resilient and have evolved to achieve their biological function in the harsh conditions found in the gut. Indeed, this trait is being exploited in nanotechnology where exogenous gut enzymes are attached to ENMs for enhanced catalysis.76 Of course, for ENMs with organic surface coatings, the enzymatic digestion step will remove them. For example, proteases to degrade the protein corona on a particle, and lipases for the destruction of phospholipid coatings. The concentrations of total nitrogen from protein, or carbon from lipids could be determined after recovering and washing the particles using existing standard methods to assess the loss of any coating (e.g., carbon/hydrogen/nitrogen analysis,77). Similarly, for metal-containing ENMs that dissolve in the gut lumen, the dissolved metals released can be measured using routine atomic absorption spectrometry methods provided that the ENM has been totally dissolved. Where ENMs remain present, then particle number concentrations might be determined by single particle ICP-MS. Regardless, for metals the instruments may need optimising for the detection method to work with nanomaterials (e.g., TiO2,78).
Ex vivo gut sacs from fish
Whole gut sac preparations have been used for many years. They were originally employed in physiology to determine which part(s) of the gut was involved in solute absorption (amino acids, sugars, and minerals). In addition, they have been used in (eco)toxicology to determine which parts of the gastrointestinal tract are mainly involved in the absorption of toxic metals (e.g., Hg,79 Cu,80) or organic chemicals.81 This well-established physiological technique involves removing the whole gut from the animal, filling the lumen with the test substance of interest, and then suturing closed the different anatomical parts of the gut so that regional uptake into the tissue can be measured over (typically) four hours. After the incubation, the gut mucosa and underling muscle tissue (muscularis mucosae) are dissected in each region of the gut and processed for quantification of the test substance. The methodology for gut sacs has been described in detail for use with ENMs (TiO2,11). The main advantages of the preparation include that it is a close match to the gut barrier as it would exist in vivo, a reasonably fast method that can precisely identify which regions of the gut are of concern, as well as numerically demonstrating the uptake potential to the gut mucosa (and beyond) of both dissolved metal or particulate fractions. The latter would also depend on analytical techniques for determining the presence of intact ENMs in the tissue. The main disadvantage is that it is a closed system of defined volume (as is fish cell culture), and so should not be used to determine physiological uptake and excretion rates per se, although it is useful to obtain initial estimates. It is recommended that the gut sac method is used with physiological salines (see ref. 80) to obtain measurements as close to in vivo as possible. For example, in our case of the TiO2 ENMs (Fig. 4A), the gut sac preparation shows that the mucosa will accumulate Ti (form unknown, measured as total Ti) from exposure to either the nano or bulk (micron scale) form of TiO2, and in all regions of the gut. This would firmly predict that bioaccumulation into the gut mucosa would be a concern in vivo, and this is indeed the case. In Fig. 4B, for an in vivo dietary exposure of trout, the total Ti accumulation occurs in the intestine, but not much is transferred to the liver. These latter observations also suggest that the bioaccumulation potential is mainly associated with the route of entry rather than the internal organs. Nonetheless, the gut sac method correctly identified the in vivo concern. The gut sac approach works with 0.9% NaCl solutions over a few hours, although physiological gut saline is recommended. It is also possible to test more complex liquids such as slurries of food ingredients (wet animal feeds), or even foods intended as liquids (e.g., drinks, soups for human consumption), although the latter have yet to be tested with the gut sac method above and may provide difficulties in measurements.
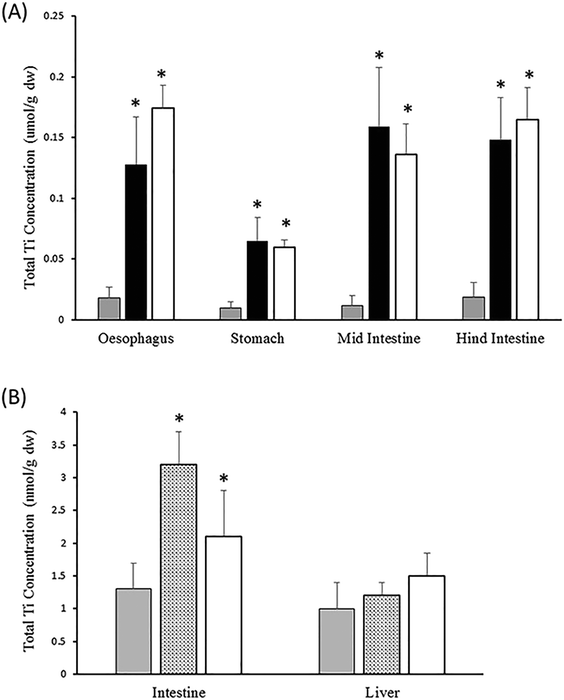 |
| Fig. 4 Example data on TiO2 for tier 3 (panel A) and tier 4 (panel B) of the testing strategy. (A) Total Ti metal concentrations in the mucosa of whole gut sacs from rainbow trout exposed to 1 mg L−1 TiO2 in the gut lumen for 4 h (data from Al-Jubory and Handy11). Control (grey bars), bulk TiO2 (black bars), P25 TiO2 ENMs (white bars). The mucosa accumulates Ti indicating some potential for bioaccumulation. (B) In vivo results from trout fed diets containing no added TiO2 (control, grey bars), or 10 (stippled bars), or 100 mg P25 TiO2 ENMs kg−1 food (white bars) for six weeks. Data redrawn from Ramsden et al.34 Note, like the gut sac studies, the intestine in vivo shows Ti accumulation, but not the liver. In both panels, data are means ± S.E., n = 6. *Significantly different from the control (ANOVA or Kruskal Wallis, P < 0.05). The in vivo studies were performed at Plymouth University with ethical approval from the local ethics committee and authorisation by a project license (under the UK Home Office Scientific Procedures Act) held by R. D. Handy. | |
Tier 4: an in vivo dietary bioaccumulation test for ENMs
Purpose and design of the in vivo test
Tier 4 is to conduct an in vivo dietary exposure bioaccumulation test in fish for ENMs. This tier would only be used on selected ENMs, where the results from tier 3 especially, identify the ENM as bioavailable to the gut and therefore ‘of concern’. The in vivo bioaccumulation test aims to measure the ingested dose (i.e., concentration of the ENM in the food multiplied by ration when all the food is eaten) and compare this with the concentration of the ENM achieved inside the animal of a known body mass at the end of the experiment (i.e., the accumulated internal dose). The presence of ENM remaining in/on the gastrointestinal tract may confound the total body burden measurement, and it is recommended to exclude the gut tissue from the body burden determination, although it should also be analysed separately for data interpretation and reporting. This allows to differentiate bioaccumulation in the gut as the route of exposure from any bioaccumulation by the internal organs, as illustrated from the data in Fig. 4B for TiO2.
The test design can follow that of the ‘dietary exposure bioaccumulation fish test’ in part III of the OECD TG 305 with some modifications for ENMs as described below. The statistical power, feasibility, costs and animal welfare issues should also be considered as explained in the TG 305, and as it would be for any substance. Fundamental research on dietary exposure to ENMs have mostly adopted the long-established triplicate tank experimental design of the fish nutrition industry; where the tank not the fish is the unit of replication. The OECD TG 305 uses pseudo-replication where the individual fish is the experimental unit and taken from one tank at each exposure dose. This pseudo-replication is partly driven by animal welfare/ethical issues to minimise the number of animals used in the test. However, while pseudo-replication is adopted here, it is yet to be determined experimentally if this is adequate replication for ENMs.
The treatments in the study design can follow TG 305 with the control animals being fed normal uncontaminated food, a diet containing the ENM of concern; and where relevant a metal salt control and/or a bulk (micron-scale) powder control. To determine potential concentration dependence of ENM uptake, additional exposure concentration(s) could be included. A 1–2% maintenance ration should be used to avoid excessive growth. The feeding of the fish should be done carefully so that a known amount of food is added to the tank and is all eaten within one or two minutes. The time points and duration of the test need to be considered for ENMs. OECD TG 305 recommends an uptake phase of 7–14 days in a dietary exposure test, and a depuration phase of up to 28–42 days. Some dietary studies conducted so far show that steady accumulation in the tissues can occur after four to six weeks of exposure (e.g., TiO2,34). However, a clear accumulation of Zn in rainbow trout tissues exposed to ZnO nanoparticles through the diet was observed after just 11 days of exposure, following the recommended protocol of OECD TG 305.82 Clearly for ENMs, range finding trials should be done, and the range finding protocol in TG 305 should be used. The uptake rates for ENMs may be a little slower than traditional organic chemicals, and so increasing the dose may ensure a usable 14 day uptake phase. There are very few data on clearance of ENMs from fish following a dietary exposure, or even data to show if clearance occurs, and with uncertainty regarding the duration of the clearance phase, the principles outlined in TG 305 would apply.
In TG 305 several species are recommended, but the experience with ENMs in dietary bioaccumulation studies so far is limited mostly to larger fish that are easier to dissect, for instance, rainbow trout that is a good candidate for BMF studies as a predator with acid digestion. Carp and other herbivorous fish have a different digestion system (e.g., small/no stomach, other pH conditions in the upper gut). It is not the species of fish per se that is important, but the feeding habit of the animal and the anatomy/physiology of the gut that is functionally matched to the type of food normally eaten. Thus, it is likely that the apparent digestibility and bioavailable fractions from a feed will be different for carnivores and herbivores. Arguably, the stronger acid digestion of carnivores might inform on a ‘worse case’ situation for the uptake of ENMs. On the other hand, herbivores are better designed to process complex carbohydrates and may be a better test organism for some of the organic ENMs (e.g., dendrimers) or ENMs with organic coatings. In terms of the observations during the test and the sampling of the fish, this can generally follow TG 305, with some caveats (see below).
Methods for preparing diets containing nanomaterials and confirming composition
The methods for preparing fish foods for toxicological studies are well-established in the scientific literature,32 and in OECD protocols.23,24 The methods have also been applied to ENMs.34,35 The approaches include preparing the diets from the raw dry ingredients (i.e., the fish meal, a powdered carbohydrate source, vitamins and minerals pre-mix, etc.) and then adding the test substance to the dry mix as a powder, or by spraying it as a liquid (i.e., a stock dispersion of the ENM) onto the dry ingredients to form a food paste. The wet paste is then extruded into pellets and dried. The choice of adding the ENM as a dry powder or as a dispersion might be defined by how the ENM is supplied by the manufacturer; but in either case the food ingredients must be carefully mixed such that the ENM is evenly dispersed in the food. The aim is to achieve a dry, pelleted animal feed where the ENM is likely to be found in each pellet at a similar concentration.
To confirm that the pellets contain the test substance of interest, some analytical chemistry should be performed. For metal-containing ENMs, the total metal concentration of sub-samples of the pellets from the batch of food can be analysed by inductively coupled plasma optical emission spectrophotometry (ICP-OES) or ICP-MS after chemically digesting the food pellets in neat nitric acid or aqua regia (e.g., TiO2 (ref. 34 and 78)). This serves to confirm the measured and nominal total metal concentrations in the feed, but also the homogeneity of the pellets, such that it is reasonable that a fish would ingest the ENM in a single feeding of the pellets. However, some consideration of the likely background metal concentrations in the food is also needed when initially formulating the exposure concentrations, so that the test diets include higher concentrations than the controls of normal animal food. For example, for dietary bioaccumulations tests involving nano-iron, commercial fish foods unavoidably contain around 100–200 mg kg−1 of Fe, or higher.83 Similar arguments apply to the high Ca background in fish food (1–2 g Ca kg−1 of food84) and studies on nano-hydroxyapatite.
The detection of carbon-based ENMs in food pellets is more problematic because of the high carbon background. One approach to at least understanding if the ingredients are mixed properly is to add an inert marker to the dry ingredients just before mixing, such as chromic oxide that has been used in fish nutrition.85 Yttrium oxide has also been used for CNT and C60 diets,35 although some consideration of the subsequent analytical method is needed (yttrium oxide is often used as an internal standard on ICP-MS for example). It may not be necessary to intentionally add an inert tracer to the food ingredients. The presence of trace contaminants in the ENM itself could serve as a marker (e.g., trace cobalt or gold from the synthesis of carbon nanotubes). The ENMs can also be radio-labelled during production, for example 14C labelled CNT.86 OECD TG 305 allows the use of radiolabelled substances, and so the approach is simply extended to ENMs here.
If the ENM is added as a liquid, it is preferable to avoid the use of organic solvents (the fish can taste them) and dispersing agents which can be toxic to fish (see ref. 19). However, if a dispersing agent must be used, then the control diet should be made with the dispersing agent without the ENM present. Regardless of the method, the blending of the ingredients should achieve uniform food pellets of homogeneous composition with respect to nutritional value and the concentration of the test substance or ENM (see also the OECD23,24).
An alternative to incorporating the ENM into the food pellets, and much easier from the view point of a routine test method, is to simply top coat a commercially available food pellet with the ENM. The advantages of this approach include: speed, no equipment is needed for making animal feed, and no expertise in fish food formulation with ENMs is required. Typically, a top coating is achieved by spraying a few % gelatine solution containing the test substance onto the commercial food pellets and then letting them dry (e.g., ref. 87). This approach with gelatine is likely the best method for a particulate material as the ENM would be trapped in the matrix of the gelatine. In any event, a simple leaching test with the food pellets can determine if losses from the top coat is a concern. The current TG 305 (ref. 23 and 24) uses a variant of this approach with organic chemicals in mind, such as mixing the test substance in a small amount of edible oil and then allowing this to soak into the feed. This last methodology, using oily suspensions of ZnO nanoparticles, has been successfully used in a bioaccumulation study on rainbow trout.82 In this latter study, the nominal and measured total Zn concentrations in the food were in agreement and there was no appreciable leaching of Zn from the food over 5 hours in water.
Whatever method is used to make the food, it is critical that the final animal feed is both palatable and of nutritional value to the fish. TG 305 also asks for nutritional value information to be reported. For example, metals such as Cu are known to oxidise animal feed causing a subsequent oxidative stress (vitamin E depletion) in the animal.88 There may be similar concerns for oxidising ENMs. Furthermore, ENMs have physical properties that may affect the food. For example, carbon nanotubes unduly harden the food pellets,35 also changing the colour or buoyancy of the food to influence feeding behaviour. Low palatability and the refusal of the feed by the fish will invalidate the test, because the exposure has not proceeded as intended. Uneaten food also creates uncertainty about the actual ingested dose. It is recommended to check that the fish will eat any experimental diets, by trying the feed on a few fish before the start of the main experiment (also recommended in OECD protocols23,24). The primary concern is to make a food that is readily eaten and to record the water quality during the experiment to confirm the absence of the test substance in the water column.
Measuring tissue concentrations of ENMs
A bioaccumulation-style test inevitably requires some determination of the test substance in the internal organs or tissues of the fish. For ENMs, the rules with respect to target organs and ADME are yet to be established with dietary exposures in fish. However, pragmatically there is no reason to deviate from the usual target organs. This should include the liver as a central compartment, the gut mucosa (the route of exposure) and the gill to help exclude the notion of waterborne exposure.31,32 Where human health is a concern from edible fish, the skeletal muscle from the flank can be collected. The skeletal muscle also accounts for two thirds or more of the body mass, and enables an estimate of total body burden.
The analytical challenges for measuring ENMs in fish tissues are broadly the same as for the food (above). Modified ICP-OES methods are available for measuring total metal in the internal organs of fish from metal-containing ENM exposures (Cu nanoparticles;12 TiO2 (ref. 78)). However, a central question for the bioaccumulation hazard assessment is whether or not the ENM has been taken up in particulate or dissolved form. For ENMs that are resistant to all but hydrofluoric acid digestion, such as TiO2, it is possible to digest the fish tissue in nitric acid for a general determination of total metals by ICP-OES and yet enable some attempt at single particle ICP-MS with the same sample (see ref. 78). However, in most cases, this is not possible and two different types of chemical digestion of the tissue are needed to determine the body burden of metal-containing ENMs. These are: (i) a concentrated nitric acid or aqua regia digest for a selection of electrolytes and metal by ICP-OES or MS, and (ii) a strong alkali digestion using tetramethylammonium hydroxide in order to generate a liquid sample that can be used for spICP-MS.89 This latter technique can provide information on particle number concentration per gram of tissue as well as the metal concentration. However, there are limitations. The spICP-MS technique, currently, cannot detect particles smaller than around 10–20 nm, depending on the material.90 For fish tissues, the detection limit for the analyte in particulate form may not be sufficient to measure the control tissue samples, other than to say that they are, or are not, contaminated. However pragmatically, spICP-MS is currently the only realistic method that could be available routinely. For carbon-based ENMs, the methodologies are currently limited to either using radiolabelled materials or using extractions with organic solvents for stable and diffusible ENMs such as pristine C60. For most types of carbon-based ENMs, adequate standard methods for measuring tissue samples are yet to be developed, although some promising approaches are developing.62 Alternative methods such as collecting tissues for electron microscopy to count particles are too labour intensive, time consuming and ultimately semi-quantitative.
Data analysis and reporting from the in vivo test
Data reporting from the in vivo test should follow that given in TG 305, with additional commentary on the nano-specific issues for the test described above. The reporting should confirm the conduct of the test and any unexpected deviations from it, as recommended in TG 305.23 The aim of the experiment is of course to measure the concentration of the ENM in the animals compared to the ingested dose to determine the bioaccumulation potential. The calculations set out in TG 305 (ref. 23) and its Guidance24 may be used with the caveats on terminology and assumptions of apparent bioaccumulation for ENMs described above. The calculations of the apparent net accumulation of the ENM can be derived from curve fitting of the raw data points of the measured tissue concentrations against time for the uptake and depuration phases of the experiment. At this time, given the uncertainties in the theoretical foundations for any uptake kinetics for ENMs, an empirical approach of showing the best curve fit to the data is preferred.24 The uptake and elimination rate constants from the curves can also be used to calculate the apparent net accumulation. However, it is also important to recognise that whatever value of apparent accumulation is derived, it will be specific to the administered dose. For solutes such as metals, the absorption efficiency across the gut declines (often exponentially) with the ingested dose. Such absorption efficiency curves have not been derived for ENMs, and the extrapolation of the calculated apparent bioaccumulation factor to higher or lower doses will have considerable uncertainty and is best avoided.
In vivo bioaccumulation experiments represent a significant investment of time and resources, and with only a few ENMs tested so far via the dietary route, researchers should not feel restricted to only the endpoints in TG 305. Although not strictly required for regulatory purposes, there are other biochemical and physiological endpoints that could be informative. For example, for oxidising ENMs the determination of total glutathione in the gills, gut and liver can inform on oxidative stress. Ionoregulatory toxicity from metallic ENMs can be determined from tissue electrolyte concentrations, plasma ions and blood osmolarity (e.g., ref. 34 for these endpoints). For animal health, routine haematology and organ histology can be especially helpful since the aetiology of pathology from ENMs is subtly different to other similar chemicals (e.g., Cu ENMs91). Dossiers for environmental risk assessment permit background or supporting information, and such new data on ENMs could be used to strengthen the weight of evidence for the risk assessment.
Conclusions and recommendations
A tiered approach to the testing for bioaccumulation potential in fish is proposed and recommended. The potentially vast array of different shapes, sizes, surface coatings and chemical compositions of ENMs, in our opinion, present too many combinations for each ENM to be tested as a ‘new substance’ in vivo. Our tiered approach is partly driven by the need to rationalise the burden of work on industry and regulators, so that only the ENMs of most concern go forward to full in vivo testing. It is also driven by animal welfare and reduces the use of animals for in vivo testing by having chemistry triggers of concern and screening steps using computational tools, as well as in chemico and ex vivo measurements. The final in vivo step in the testing strategy is a last resort and intended only for ENMs identified as a significant concern from the earlier tiers. We firmly recommend that this testing strategy is considered by regulatory agencies and to independently validate the methods proposed here in a regulatory setting. This includes new chemistry triggers of concern of relevance to ENMs, rather than continuing with the traditional octanol–water partition coefficient test. The process of validating the in chemico digestion method and ex vivo gut sac approach for regulatory use can draw on the experience and data from the fish physiology community. Similarly, with the computational tools and data mining in the strategy, the thinking is to adapt and apply existing tools to ENMs. The final in vivo step also uses the wealth of experience in the OECD TG 305, but with some caveats for ENMs that could be incorporated into guidance documents on bioaccumulation testing.
Conflicts of interest
There are no conflicts of interest.
Acknowledgements
The authors have been engaged in drafting a TG on bioaccumulation testing with ENMs following an OECD meeting of the Working Part on Manufactured Nanomaterials (WPMN) in Berlin 2013. This current manuscript is the work of the authors alone, but the fruitful discussions at the Berlin meeting are acknowledged, especially those from Steve Diamond on the concept of a tiered approach to effects testing. Handy and von der Kammer were partly supported by the EU H2020 NanoFase project, grant agreement No 646002.
References
- S. Strempel, M. Scheringer, C. A. Ng and K. Hungerbuhler, Environ. Sci. Technol., 2012, 46, 5680–5687 CrossRef PubMed.
- S. J. Klaine, P. J. J. Alvarez, G. E. Batley, T. F. Fernandes, R. D. Handy, D. Y. Lyon, S. Mahendra, M. J. McLaughlin and J. R. Lead, Environ. Toxicol. Chem., 2008, 27, 1825–1851 CrossRef PubMed.
- G. V. Lowry, K. B. Gregory, S. C. Apte and J. R. Lead, Environ. Sci. Technol., 2012, 46, 6893–6899 CrossRef PubMed.
- W. Peijnenburg, M. Baalousha, J. W. Chen, Q. Chaudry, F. Von der kammer, T. A. J. Kuhlbusch, J. Lead, C. Nickel, J. T. K. Quik, M. Renker, Z. Wang and A. A. Koelmans, Crit. Rev. Environ. Sci. Technol., 2015, 45, 2084–2134 CrossRef.
- R. D. Handy, T. B. Henry, T. M. Scown, B. D. Johnston and C. R. Tyler, Ecotoxicology, 2008, 17, 396–409 CrossRef PubMed.
- A. Kahru and H. C. Dubourguier, Toxicology, 2010, 269, 105–119 CrossRef PubMed.
- S. J. Klaine, A. A. Koelmans, N. Horne, S. Carley, R. D. Handy, L. Kapustka, B. Nowack and F. von der Kammer, Environ. Toxicol. Chem., 2012, 31, 3–14 CrossRef PubMed.
- A. G. Schultz, D. Boyle, D. Chamot, K. J. Ong, K. J. Wilkinson, J. C. McGeer, G. Sunahara and G. G. Goss, Environ. Chem., 2014, 11, 207–226 CrossRef.
-
R. D. Handy and F. B. Eddy, in Physicochemical Kinetics and Transport at Chemical-Biological Interphases, ed. H. P. V. L. W. Köster, John Wiley, Chichester, 2004, pp. 337–356 Search PubMed.
- B. J. Shaw and R. D. Handy, Environ. Int., 2011, 37, 1083–1097 CrossRef PubMed.
- A. R. Al-Jubory and R. D. Handy, Nanotoxicology, 2013, 7, 1282–1301 CrossRef PubMed.
- B. J. Shaw, G. Al-Bairuty and R. D. Handy, Aquat. Toxicol., 2012, 116, 90–101 CrossRef PubMed.
- F. R. Khan, S. K. Misra, J. Garcia-Alonso, B. D. Smith, S. Strekopytov, P. S. Rainbow, S. N. Luoma and E. Valsami-Jones, Environ. Sci. Technol., 2012, 46, 7621–7628 CrossRef PubMed.
- Y. Wang, A. J. Miao, J. Luo, Z. B. Wei, J. J. Zhu and L. Y. Yang, Environ. Sci. Technol., 2013, 47, 10601–10610 CrossRef PubMed.
- L. C. Felix, V. A. Ortega, J. D. Ede and G. G. Goss, Environ. Sci. Technol., 2013, 47, 6589–6596 CrossRef PubMed.
- R. Bjorkland, D. A. Tobias and E. J. Petersen, Environ. Sci.: Nano, 2017, 4, 747–766 RSC.
- G. D. Veith, D. L. Defoe and B. V. Bergstedt, J. Fish. Res. Board Can., 1979, 36, 1040–1048 CrossRef.
-
OECD, OECD Series on Testing and Assessment, No. 23. Guidance Document on Aquatic Toxicity Testing of Difficult Substances and Mixtures ENV/JM/MONO(2000)6, Organisation for Econonomic Co-operation and Development (OECD), Paris, France, Available from: http://www.oecd.org/officialdocuments/publicdisplaydocumentpdf/?doclanguage=en&cote=env/jm/mono(2000)6, 2000 Search PubMed.
- R. D. Handy, G. Cornelis, T. Fernandes, O. Tsyusko, A. Decho, T. Sabo-Attwood, C. Metcalfe, J. A. Steevens, S. J. Klaine, A. A. Koelmans and N. Horne, Environ. Toxicol. Chem., 2012, 31, 15–31 CrossRef PubMed.
- K. Law, V. P. Palace, T. Halldorson, R. Danell, K. Wautier, B. Evans, M. Alaee, C. Marvin and G. T. Tomy, Environ. Toxicol. Chem., 2006, 25, 1757–1761 CrossRef PubMed.
- M. C. Barber, Environ. Toxicol. Chem., 2008, 27, 755–777 CrossRef PubMed.
- J. A. Arnot and C. L. Quinn, Environ. Sci. Technol., 2015, 49, 4783–4796 CrossRef PubMed.
-
OECD, Bioaccumulation in fish: aqueous and dietary exposure. OECD guidelines for testing of chemicals. Technical Guidance (TG) 305, Organisation for Economic Cooperation and Development (OECD) Publishing, Paris, France, Available at: DOI:10.1787/9789264185296-en, 2012.
-
OECD, OECD Series on Testing and Assessment, No. 264. Guidance Document on Aspects of OECD TG 305 on Fish Bioaccumulation, Organisation for Economic Cooperation and Development (OECD) Publishing, Paris, France, Available at: http://www.oecd.org/env/ehs/testing/series-testing-assessment-publications-number.htm, 2017 Search PubMed.
- R. D. Handy, F. von der Kammer, J. R. Lead, M. Hassellov, R. Owen and M. Crane, Ecotoxicology, 2008, 17, 287–314 CrossRef PubMed.
- L. C. Felix, V. A. Ortega and G. G. Goss, Aquat. Toxicol., 2017, 192, 58–68 CrossRef PubMed.
- C. Gitrowski, A. R. Al-Jubory and R. D. Handy, Toxicol. Lett., 2014, 226, 264–276 CrossRef PubMed.
- J. L. Ferry, P. Craig, C. Hexel, P. Sisco, R. Frey, P. L. Pennington, M. H. Fulton, I. G. Scott, A. W. Decho, S. Kashiwada, C. J. Murphy and T. J. Shaw, Nat. Nanotechnol., 2009, 4, 441–444 CrossRef PubMed.
- L. M. Skjolding, M. Winther-Nielsen and A. Baun, Aquat. Toxicol., 2014, 157, 101–108 CrossRef PubMed.
- M. N. Croteau, A. D. Dybowska, S. N. Luoma and E. Valsami-Jones, Nanotoxicology, 2011, 5, 79–90 CrossRef PubMed.
- S. J. Clearwater, A. M. Farag and J. S. Meyer, Comp. Biochem. Physiol., Part C: Toxicol. Pharmacol., 2002, 132, 269–313 CrossRef.
-
R. D. Handy, J. C. McGeer, H. E. Allen, P. E. Drevnick, J. W. Gorsuch, A. S. Green, A.-K. Lundebye-Haldorsen, S. E. Hook, D. R. Mount and W. A. Stubblefield, in Toxicity of Dietborne Metals to Aquatic Organisms, ed. J. S. Meyer, W. J. Adams, K. V. Brix, S. N. Luoma, D. R. Mount, W. A. Stubblefield and C. M. Wood, SETAC Press Pensacola, USA, 2005, pp. 59–112 Search PubMed.
- A. T. Fisk, R. J. Norstrom, C. D. Cymbalisty and D. C. G. Muir, Environ. Toxicol. Chem., 1998, 17, 951–961 CrossRef.
- C. S. Ramsden, T. J. Smith, B. J. Shaw and R. D. Handy, Ecotoxicology, 2009, 18, 939–951 CrossRef PubMed.
- T. W. K. Fraser, H. C. Reinardy, B. J. Shaw, T. B. Henry and R. D. Handy, Nanotoxicology, 2011, 5, 98–108 CrossRef PubMed.
- J. H. E. Arts, M. Hadi, M. A. Irfan, A. M. Keene, R. Kreiling, D. Lyon, M. Maier, K. Michel, T. Petry, U. G. Sauer, D. Warheit, K. Wiench, W. Wohlleben and R. Landsiedel, Regul. Toxicol. Pharmacol., 2015, 71, S1–S27 CrossRef PubMed.
-
ECHA, Usage of (eco)toxicological data for bridging data gaps between and grouping of nanoforms of the same substance – Elements to consider, European Chemicals Agency (ECHA), Joint Research Centre (JRC), and Dutch National Institute for Public Health and the Environment (RIVM), Helsinki, Finland, Available from: https://echa.europa.eu/documents/10162/13630/eco_toxicological_for_bridging_grouping_nanoforms_en.pdf/245bf47d-4955-4202-b0d0-1d3c531346d4, 2016 Search PubMed.
- A. G. Oomen, E. A. J. Bleeker, P. M. J. Bos, F. van Broekhuizen, S. Gottardo, M. Groenewold, D. Hristozov, K. Hund-Rinke, M. A. Irfan, A. Marcomini, W. Peijnenburg, K. Rasmussen, A. S. Jimenez, J. J. Scott-Fordsmand, M. van Tongeren, K. Wiench, W. Wohlleben and R. Landsiedel, Int. J. Environ. Res. Public Health, 2015, 12, 13415–13434 CrossRef PubMed.
-
K. Sellers, N. M. Deleebeeck, M. Messiean, M. Jackson, E. A. J. Bleeker, D. T. H. M. Sijm and F. A. Van Broekhuizen, Grouping nanomaterials: A strategy towards grouping and read-across. Rijksinstituut voor Volksgezondheid en Milieu RIVM., 2015 Search PubMed.
- T. Walser and C. Studer, Regul. Toxicol. Pharmacol., 2015, 72, 569–571 CrossRef PubMed.
- K. D. Hristovski, P. K. Westerhoff and J. D. Posner, J. Environ. Sci. Health, Part A: Toxic/Hazard. Subst. Environ. Eng., 2011, 46, 636–647 CrossRef PubMed.
-
ECHA, Guidance on information requirements and chemical safety assessment, Chapter R.7c: Endpoint specific guidance, Version 3.0. Guidance for the implementation of REACH, European Chemicals Agency (ECHA), Helsinki, Finland, Available from: https://echa.europa.eu/documents/10162/13632/information_requirements_r7c_en.pdf, 2017 Search PubMed.
- S. Ottofülling, F von der Kammer and T. Hofmann, Environ. Sci. Technol., 2011, 45, 10045–10052 CrossRef PubMed.
- A. Praetorius, J. Labille, M. Scheringer, A. Thill, K. Hungerbuehler and J.-Y. Bottero, Environ. Sci. Technol., 2014, 48, 10690–10698 CrossRef PubMed.
-
OECD, Dispersion Stability of Nanomaterials in Simulated Environmental Media. OECD guidelines for testing of chemicals. Technical Guidance (TG) 318, Organisation for Economic Cooperation and Development (OECD) Publishing, Paris, France, Available at: DOI:10.1787/9789264284142-en, 2017.
-
OECD, Test No. 107: Partition Coefficient (n-octanol/water): Shake Flask Method, 1995 Search PubMed.
- M. L. Fernández-Cruz, D. Hernández-Moreno, J. Catalán, R. Cross, H. Stockmann-Juvala, J. Cabellos, V. R. Lopes, M. Matzke, N. Ferraz and J. Izquierdo, Environ. Sci.: Nano, 2018, 5, 381–397 RSC.
- R. Landsiedel, Environ. Pollut., 2016, 218, 1376–1380 CrossRef PubMed.
-
A. Worth, K. Aschberger, D. Asturiol Bofill, J. Bessems, K. Gerloff, R. Graepel, E. Joossens, L. Lamon, T. Palosaari and A. Richarz, Evaluation of the availability and applicability of computational approaches in the safety assessment of nanomaterials, EUR 28617 EN, Publications Office of the European Union, Luxembourg, 2017, ISBN 978-92-79-68708-2, DOI:10.2760/248139, JRC106386, 2017.
-
ECHA, Appendix R.6-1 for nanomaterials applicable to the Guidance on QSARs and Grouping of Chemicals. Guidance on information requirements and chemical safety assessment Version 1.0, European Chemicals Agency (ECHA), Helsinki, Finland, Available from: https://echa.europa.eu/documents/10162/23036412/appendix_r6_nanomaterials_en.pdf, 2017 Search PubMed.
- A. P. Toropova and A. A. Toropov, Mini-Rev. Med. Chem., 2015, 15, 608–621 CrossRef PubMed.
- Y. L. Wang, J. Xing, Y. Xu, N. N. Zhou, J. L. Peng, Z. P. Xiong, X. Liu, X. M. Luo, C. Luo, K. X. Chen, M. Y. Zheng and H. L. Jiang, Q. Rev. Biophys., 2015, 48, 488–515 CrossRef PubMed.
- P. Ambure, R. B. Aher, A. Gajewicz, T. Puzyn and K. Roy, Chemom. Intell. Lab. Syst., 2015, 147, 1–13 CrossRef.
- G. C. Chen, W. Peijnenburg, Y. L. Xiao and M. G. Vijver, Int. J. Mol. Sci., 2017, 18(7), 1504 CrossRef PubMed.
- F. Gottschalk, T. Y. Sun and B. Nowack, Environ. Pollut., 2013, 181, 287–300 CrossRef PubMed.
- T. Y. Sun, D. M. Mitrano, N. A. Bornhoft, M. Scheringer, K. Hungerbuhler and B. Nowack, Environ. Sci. Technol., 2017, 51, 2854–2863 CrossRef PubMed.
- D. A. Winkler, Toxicol. Appl. Pharmacol., 2016, 299, 96–100 CrossRef PubMed.
- P. M. Costa and B. Fadeel, Toxicol. Appl. Pharmacol., 2016, 299, 101–111 CrossRef PubMed.
- M. Crane, R. D. Handy, J. Garrod and R. Owen, Ecotoxicology, 2008, 17, 421–437 CrossRef PubMed.
- A. Bourgeault, C. Cousin, V. Geertsen, C. Cassier-Chauvat, F. Chauvat, O. Durupthy, C. Chanéac and O. Spalla, Environ. Sci. Technol., 2015, 49, 2451–2459 CrossRef PubMed.
- S. Dalai, V. Iswarya, M. Bhuvaneshwari, S. Pakrashi, N. Chandrasekaran and A. Mukherjee, Aquat. Toxicol., 2014, 152, 139–146 CrossRef PubMed.
- M. D. Hernando, P. Rosenkranz, M. M. Ulaszewska, M. L. Fernandez-Cruz, A. R. Fernandez-Alba and J. M. Navas, Anal. Bioanal. Chem., 2012, 404, 2749–2763 CrossRef PubMed.
- M. Geppert, L. Sigg and K. Schirmer, Environ. Sci.: Nano, 2016, 3, 388–395 RSC.
- Y. Yue, R. Behra, L. Sigg, P. F. Freire, S. Pillai and K. Schirmer, Nanotoxicology, 2015, 9, 54–63 CrossRef PubMed.
- A. Bermejo-Nogales, M. Connolly, P. Rosenkranz, M. L. Fernandez-Cruz and J. M. Navas, Ecotoxicol. Environ. Saf., 2017, 138, 309–319 CrossRef PubMed.
- J. Susewind, C. de Souza Carvalho-Wodarz, U. Repnik, E.-M. Collnot, N. Schneider-Daum, G. W. Griffiths and C.-M. Lehr, Nanotoxicology, 2016, 10, 53–62 Search PubMed.
-
ECHA, Guidance on information requirements and chemical safety assessment, Chapter R.11: PBT Assessment, Version 3.0. Guidance for the implementation of REACH, European Chemicals Agency (ECHA), Helsinki, Finland, Available from: https://echa.europa.eu/documents/10162/13632/information_requirements_r11_en.pdf, 2017 Search PubMed.
- A. G. Oomen, A. Hack, M. Minekus, E. Zeijdner, C. Cornelis, G. Schoeters, W. Verstraete, T. Van de Wiele, J. Wragg, C. J. M. Rompelberg, A. Sips and J. H. Van Wijnen, Environ. Sci. Technol., 2002, 36, 3326–3334 CrossRef PubMed.
- S. Denys, J. Caboche, K. Tack, G. Rychen, J. Wragg, M. Cave, C. Jondreville and C. Feidt, Environ. Sci. Technol., 2012, 46, 6252–6260 CrossRef PubMed.
- J. Wragg, M. Cave, N. Basta, E. Brandon, S. Casteel, S. Denys, C. Gron, A. Oomen, K. Reimer, K. Tack and T. Van de Wiele, Sci. Total Environ., 2011, 409, 4016–4030 Search PubMed.
- C. G. Carter, M. P. Bransden, R. J. van Barneveld and S. M. Clarke, Aquaculture, 1999, 179, 57–70 CrossRef.
- K. Rungruangsak-Torrissen, A. Rustad, J. Sunde, S. Eiane, H. Jensen, J. Opstvedt, E. Nygard, T. Samuelsen, H. Mundheim, U. Luzzana and G. Venturini, J. Sci. Food Agric., 2002, 82, 644–654 CrossRef.
- K. Tervonen, G. Waissi, E. J. Petersen, J. Akkanen and J. V. K. Kukkonen, Environ. Toxicol. Chem., 2010, 29, 1072–1078 Search PubMed.
- J. D. Hopson, R. R. Johnson and B. A. Dehority, J. Anim. Sci., 1963, 22, 448–453 CrossRef.
- K. J. Ong, T. J. MacCormack, R. J. Clark, J. D. Ede, V. A. Ortega, L. C. Felix, M. K. M. Dang, G. B. Ma, H. Fenniri, J. G. C. Veinot and G. G. Goss, PLoS One, 2014, 9(3), e90650 CrossRef PubMed.
- B. H. San, S. Kim, S. H. Moh, H. Lee, D. Y. Jung and K. K. Kim, Angew. Chem., 2011, 123, 12130–12135 CrossRef.
- A. Besinis, T. De Peralta and R. D. Handy, Nanotoxicology, 2014, 8, 1–16 CrossRef PubMed.
- B. J. Shaw, C. S. Ramsden, A. Turner and R. D. Handy, Chemosphere, 2013, 92, 1136–1144 CrossRef PubMed.
- I. Hoyle and R. D. Handy, Aquat. Toxicol., 2005, 72, 147–159 CrossRef PubMed.
- R. D. Handy, M. M. Musonda, C. Phillips and S. J. Falla, J. Exp. Biol., 2000, 203, 2365–2377 Search PubMed.
- N. W. E. Clark and J. K. Chipman, Toxicol. Lett., 1995, 81, 33–38 CrossRef PubMed.
- M. Connolly, M. Fernandez, E. Conde, F. Torrent, J. M. Navas and M. L. Fernandez-Cruz, Sci. Total Environ., 2016, 551, 334–343 CrossRef PubMed.
- F. Andersen, A. Maage and K. Julshamn, Aquacult. Nutr., 1996, 2, 41–47 CrossRef.
- J. Vielma and S. P. Lall, Aquaculture, 1998, 160, 117–128 CrossRef.
- S. J. Davies and A. Gouveia, Aquacult. Nutr., 2006, 12, 451–458 CrossRef.
- E. J. Petersen, J. Akkanen, J. V. K. Kukkonen and W. J. Weber, Environ. Sci. Technol., 2009, 43, 2969–2975 CrossRef PubMed.
- B. J. Shaw and R. D. Handy, Aquat. Toxicol., 2006, 76, 111–121 CrossRef PubMed.
- R. T. M. Baker, R. D. Handy, S. J. Davies and J. C. Snook, Mar. Environ. Res., 1998, 45, 357–365 CrossRef.
- E. P. Gray, J. G. Coleman, A. J. Bednar, A. J. Kennedy, J. F. Ranville and C. P. Higgins, Environ. Sci. Technol., 2013, 47, 14315–14323 CrossRef PubMed.
- S. Lee, X. Y. Bi, R. B. Reed, J. F. Ranville, P. Herckes and P. Westerhoff, Environ. Sci. Technol., 2014, 48, 10291–10300 CrossRef PubMed.
- G. A. Al-Bairuty, B. J. Shaw, R. D. Handy and T. B. Henry, Aquat. Toxicol., 2013, 126, 104–115 CrossRef PubMed.
|
This journal is © The Royal Society of Chemistry 2018 |