DOI:
10.1039/C7RA13490H
(Paper)
RSC Adv., 2018,
8, 6200-6205
A highly efficient nano-sized Cu2O/SiO2 egg-shell catalyst for C–C coupling reactions†
Received
20th December 2017
, Accepted 22nd January 2018
First published on 7th February 2018
Abstract
Mesoporous SiO2-supported Cu2O nanoparticles as an egg-shell type catalyst were prepared by impregnation method. The obtained Cu2O/SiO2 egg-shell nanocatalyst had a large surface area and narrow pore size distribution. In addition, most of the Cu2O nanoparticles, with sizes around 2.0 nm, were highly dispersed in the mesoporous silica. Accordingly, fast reactant diffusion to the active sites would occur, especially when the active metal sites are selectively located on the outer part of the support, i.e., the outer region of the egg shell. In solvent-free Sonogashira reactions for the synthesis of ynones from acyl chlorides and terminal alkynes, this catalyst exhibited a very high catalytic activity. The excellent catalytic performance can be attributed to the synergistic advantages of mesoporous structure and monodispersed Cu2O nanoparticles.
Introduction
Supported nanocatalysts exploiting robust metal-oxide structures have been effectively used for various heterogeneous catalytic processes.1–3 In particular, mesoporous silica supports have been widely used as catalyst support based on their high thermal stability and controllable surfaces as well as their high pore volumes and large surface areas.4–8 For example, many types of metal/silica nanocatalysts, such as metal@silica yolk–shell9 and core–shell catalysts,10,11 have been developed to enhance the catalytic activity and stability against sintering or agglomeration problems of active metal sites.
In recent years, silica-12,13 and alumina-based14–16 egg-shell-type catalysts in millimeter scale have been prepared in order to avoid the diffusional restrictions of the reactants for some catalytic reactions, such as Fischer–Tropsch synthesis.17,18 Simply, fast reactant diffusion to the active sites would occur, especially when the active metal sites are selectively located on the outer part of the support, such as the outer region of the egg shell. However, most egg-shell-type catalysts prepared using the controlled penetration of molten salt have still been focused on the millimeter scale, not the micrometer or nanometer scale.
Accordingly, we utilized the synthesized egg-shell nano-sized particles in the catalytic system. The benefits of the number of potentially reactive small-sized atoms exposed on the surface are expected to include high activity and selectivity.19 Due to these significant properties, Cu nanoparticles have been used as catalysts for a very broad range of organic transformations, such as click reactions, A3 coupling, C–H functionalization, borylation, cross-coupling and so on.20–25
In this work, we set out to use the Sonogashira protocol, which has emerged as one of the most straightforward and powerful methods for carbon–carbon formation.26 The products of ynones are a kind of conjugated alkynyl ketones which are useful intermediates of heterocyclic compounds as building blocks for natural products, pharmaceuticals, and molecular organic materials.27–30 Herein, we report a new type of Cu2O/SiO2 egg-shell nanocatalyst as an active and stable catalyst for the synthesis of 1,3-diphenyl-2-propyn-1-one from benzoyl chloride and phenylacetylene.
Experimental
Materials
Copper(II) nitrate trihydrate (Cu(NO3)2·3H2O, ≥98%), tetraethyl orthosilicate (TEOS, 98%) and hexadecyltrimethylammonium bromide (C16TAB, ≥98%) were purchased from Aldrich. Ammonium hydroxide (NH4OH, 28% in water) and ethanol (99.9%) were obtained from Junsei and Baker, respectively. The reagents were used as received without further purification.
Synthesis of mSiO2 egg-shell support
NH4OH aqueous solution (2.5 mL) was added to a mixture of ethanol (100 mL) and distilled water (8.0 mL), and then stirred for 5 min. TEOS (10 mL) was added to the prepared solution and stirred for 2 h at room temperature. The resulting silica particles were precipitated by centrifugation at 8000 rpm for 20 min, then washed thoroughly with ethanol (100 mL). The dispersed silica in ethanol (100 mL) was diluted with distilled water (200 mL). A solution of C16TAB (1.2 g, 3.3 mmol) dissolved in a solvent mixture of distilled water (20 mL) and ethanol (10 mL) was injected into the diluted silica solution. This mixture was vigorously stirred under ambient conditions for 30 min. After 30 min, TEOS (2.15 mL, 9.6 mmol) was added to the reaction solution and stirred for 12 h. The resulting mSiO2 egg-shell nanoparticles were precipitated by centrifugation at 8000 rpm for 10 min, and then washed thoroughly with ethanol (150 mL) and acetone (150 mL). After drying at 100 °C in a drying oven, the white powder was placed in an alumina boat in a tube-type furnace, heated at a ramp rate of 4 °C min−1 to 500 °C, and calcined at 500 °C for 8 h in air.
Synthesis of nano-sized Cu2O/SiO2 egg-shell catalyst
For synthesis of the Cu2O/SiO2 egg-shell nanocatalyst containing 10 wt% Cu, aqueous copper nitrate solution (8.74 M, 0.1 mL) was added dropwise onto the mSiO2 egg-shell support (0.5 g). Infiltration was performed by grinding the mixture under ambient conditions for 10 min until the powder was homogeneously pale blue. Next, the mixed powder was placed in an autoclave reactor and aged in an oven at 120 °C. After aging for 24 h, the sample was cooled in ambient atmosphere and transferred into an alumina boat in a tube-type furnace. Finally, the copper-incorporated silica powder with pale blue color was slowly heated under H2 flow of 200 mL min−1, at a ramp rate of 2.7 °C min−1, to 350 °C. The sample was thermally treated under continuous H2 flow at 350 °C for 4 h. After calcination, the resulting powder with pale green color was cooled down to room temperature.
Characterization
High-resolution transmission electron microscopy (HRTEM) was performed using a Tecnai TF30 ST and a Titan double Cs-corrected TEM instrument (Titan cubed G2 60-300). Energy-dispersive X-ray spectroscopy (EDS) elemental mapping data were collected using a higher-efficiency detection system (Super-X detector). High-power X-ray powder diffraction (XRD) (Rigaku D/MAX-2500, 18 kW) was also used for the analysis. N2 sorption isotherms were measured at 77 K with a Tristar II 3020 surface area analyser. Before the measurement, the sample was degassed under N2 flow at 300 °C for 4 h.
Activity tests
In a typical run of the solvent-free Sonogashira coupling reaction,31 0.50 mmol phenylacetylene, 0.75 mmol benzoyl chloride, 4.0 equiv. triethylamine (base) and 1.0 mol% Cu2O/SiO2 egg-shell nanocatalyst were mixed in a 25 mL oven-dried Schlenk reaction tube. After reacting in argon atmosphere at 80 °C for 12.0 h, the reaction mixture was filtered and extracted with dichloromethane, then analyzed using a gas chromatograph (SHIMADZU, GCMS-QP2010 SE).
 |
| Fig. 1 Schematic illustration of the synthesis of the Cu2O/SiO2 egg-shell nanocatalyst. | |
Results and discussion
Preparation of Cu2O/SiO2 egg-shell nanocatalyst
Fig. 1 shows a brief synthetic scheme for the Cu2O/SiO2 egg-shell nanocatalyst. The solid SiO2 cores within mesoporous SiO2 shells (mSiO2 egg-shell supports) were prepared by a modified sol–gel method as reported in the literature.32 The transmission electron microscopy (TEM) images show uniform silica nanospheres with average diameter of 305 ± 18 nm (Fig. 2a and b). Mesoporous silica shells were coated onto the silica nanospheres using the cationic surfactant C16TAB, both as a structure-directing agent and a sacrificial porogen. The TEM images show mSiO2 egg-shell supports around 435 nm, larger than the initial SiO2 nanospheres (Fig. 2c). The thickness of the uniformly coated mesoporous silica shell on the solid silica core was observed as approximately 65 nm in the HRTEM image (Fig. 2d). Ordered mesoporous channels in the silica shell could be generated by thermal removal of C16TAB.
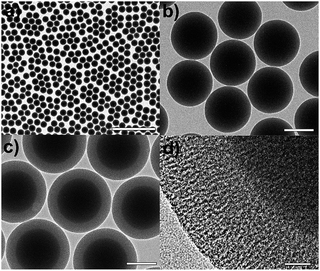 |
| Fig. 2 (a and b) TEM images of silica nanospheres, and (c) TEM and (d) HRTEM images of mSiO2 egg-shell support. The bars represent 2 μm (a), 200 nm (b, c), and 20 nm (d). | |
Next, the copper(I) oxide/silica catalyst (designated as Cu2O/SiO2 egg-shell nanocatalyst), with controlled Cu content of 10 wt% on the basis of Cu converted from the copper nitrate salt after thermal treatment, was prepared by incorporating Cu2O particles formed inside the pores of mSiO2 egg-shell supports. Impregnation of copper nitrate and subsequent hydrogen reduction yielded small and uniform Cu2O particles in channel-like SiO2 pores. The chemical reaction for the thermal decomposition of Cu(NO3)2 and Cu2O formation is proposed to be:
2Cu(NO3)2 (s) + H2 (g) → Cu2O (s) + 4NO2 (g) + H2O (g) + O2 (g) |
The TEM image indicates that the Cu2O nanoparticles were incorporated well into the mesopores of silica (Fig. 3a). The high-angle annular dark-field scanning transmission electron microscopy (HAADF-STEM) image also shows regions of varying brightness. Relatively bright spots correspond to Cu2O nanoparticles (Fig. 3b). In the elemental mapping, Cu (green) and Si (red) are confirmed (Fig. 3c–e). The magnified TEM image shows black dots in channel-like pores, indicating small Cu2O crystals (Fig. 3f). The HRTEM image and the corresponding Fourier-transform (FT) pattern indicate the single crystalline nature of the Cu2O particle (Fig. 3g). The inside particle size was observed to be between 2 and 3 nm. The lattice distance of 0.246 nm between neighbouring fringes corresponds to the (111) lattice spacing in cubic phase Cu2O. In the XRD pattern, Cu2O/SiO2 egg-shell nanocatalyst is also well matched with cuprite (Fig. 3h, space group: Pn3m, JCPDS no. 77-0199). The broad peak at 2θ = 36.5° corresponds to the (111) plane of Cu2O. The mean particle size was estimated to be 2.0 nm using the Debye–Scherrer equation from the full width at half maximum (fwhm) of the (111) peak.
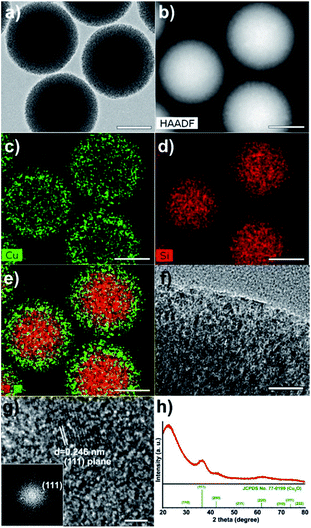 |
| Fig. 3 (a) TEM and (b) HADDF images, (c–e) scanning TEM image with elemental mapping, and (f and g) HRTEM images with the corresponding FT pattern (inset of g), and (h) XRD spectrum of Cu2O/SiO2 egg-shell nanocatalyst. The bars represent 200 nm (a–e), 20 nm (f), and 2 nm (g). | |
N2 sorption experiments for the pristine mSiO2 egg-shell support and the Cu2O/SiO2 egg-shell nanocatalyst show type IV adsorption–desorption hysteresis (Fig. 4a). The Brunauer–Emmett–Teller (BET) surface areas were calculated to be 481.2 m2 g−1 for the pristine mSiO2 egg-shell support and 228.9 m2 g−1 for the Cu2O/SiO2 egg-shell nanocatalyst, respectively. The total pore volume of the Cu2O/SiO2 egg-shell nanocatalyst was found to be 0.19 cm3 g−1, which is about 54% of the pristine mSiO2 egg-shell support (0.35 cm3 g−1). The significant decrease in pore volume of the Cu2O/SiO2 egg-shell nanocatalyst was attributed to the occupied copper oxide nanoparticle in the silica pore. The pore size distributions of the initial mSiO2 egg-shell support and Cu2O/SiO2 egg-shell nanocatalyst were identically observed in the range of 2–3 nm, well reflecting the embedded Cu2O crystallite size (Fig. 4b).
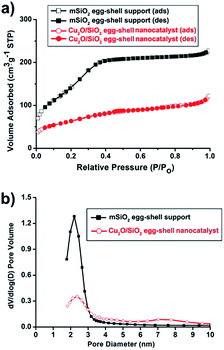 |
| Fig. 4 (a) N2 adsorption/desorption isotherms and (b) pore size distribution diagrams using the Barrett–Joyner–Halenda (BJH) method from the adsorption branches of the mSiO2 egg-shell support and the Cu2O/SiO2 egg-shell nanocatalyst. | |
Sonogashira coupling reactions
We explored the catalytic performance of the synthesized Cu2O/SiO2 egg-shell nanocatalyst toward Sonogashira coupling reactions. It was found that supported copper nanoparticles efficiently catalyzed the synthesis of ynones of acyl chlorides and alkynes.33,34 In this type of reaction, Cu2O nanoparticles activate phenylacetylene to form copper(I) acetylide compounds, which subsequently react with acyl chloride.35,36 In addition, this reaction does not require any ligand and palladium source under solvent-free conditions.
The effect of several factors, such as reaction time, temperature, base, and amount of catalyst, was studied. The results are listed in Table 1. Initially, it was demonstrated that changes in the catalyst loading have marked impacts on the conversions. It was shown that increasing the amount of catalyst loading from 1 mol% to 1.5 mol% resulted in higher conversion (86%) (Table 1, entries 1 and 2). In order to improve the conversion of the reaction, we increased the temperature to 80 °C, which resulted in higher conversion than at 40 °C (Table 1, entries 3 and 4). Thereafter, when the reaction time was extended to 12 h, the conversion of 99% was achieved (Table 1, entry 5). In addition, when the amount of base was increased (4.0 equiv.), the conversion was 100% (Table 1, entry 8), and the selectivity was 99% owing to Cu2O/SiO2 egg-shell nanocatalyst hindering the homocoupling of benzoyl chloride and phenylacetylene. Subsequently, the optimized reaction conditions were phenylacetylene (0.5 mmol), benzoyl chloride (0.75 mmol), Cu2O/SiO2 egg-shell nanocatalyst (1 mol%), and Et3N (4 equiv.) at 80 °C for 12 h under Ar atmosphere. Furthermore, when we conducted the reaction without base or catalysts under the optimized reaction conditions, each reaction hardly proceeded (Table 1, entries 6 and 7). Thus, a basic medium is essential for these cross-coupling reactions. However, under the same condition, but replacing the Cu2O/SiO2 egg-shell nanocatalyst with commercial Cu2O powder as catalyst, the coupling reaction was obtained with lower conversion and selectivity (Table 1, entry 12). Even though the conventional SiO2-supported Cu2O catalyst showed similar catalytic activity, low reusability was obtained due to decomposed catalyst structures (Table 1, entries 13 and 14). The Cu2O/SiO2 egg-shell nanocatalyst was recycled up to three times without any loss of its initial high activity (>94%) in subsequent experiments (Table 1, entries 9–11). Therefore, this indicates that the high dispersion and excellent accessibility of the Cu2O NPs cause the high efficiency of the Cu2O/SiO2 egg-shell nanocatalysts. Moreover, they showed enhanced catalytic activity and facilitated considerably positive synergistic effects with nanosized porous support substrates,37–40 as compared to Cu2O nanoparticles without the mesoporous support.41 All reactions are carried out in the void inside the shell. In other words, the egg-shell structure acts as a “nanoreactor framework”, which contains enough space between the core and shell. Each of the active nanoparticles experiences a homogeneous environment in a void surrounded by the silica shell. We also studied the role of solvent system under the optimized conditions (ESI, Table S1†).42 In addition, when we carried out the reactions with dipolar aprotic solvents such as dimethylformamide (DMF) and tetrahydrofuran (THF), results were inferior because of the formation of the corresponding anhydride as a by-product. However, the reaction under nonpolar solvent, such as toluene, gave 84% conversion. Therefore, all reactions were performed neat under anhydrous conditions. Encouraged by the above results, with these optimized reaction conditions, the scope of the developed protocol was extended for the synthesis of ynone derivatives using different substrates (Table 2). As shown in Table 2, most of the substrates gave good conversions despite electron-donating substituents (methyl, tert-butyl and methoxy groups) and electron-withdrawing substituents (fluoro, cyano groups). Furthermore, benzoyl chloride substituted with a nitro group still gave the corresponding ynones with good conversion rate (Table 2, entry 6).
Table 1 Solvent-free Sonogashira reactions for the synthesis of ynones from acyl chlorides and terminal alkynes catalyzed by Cu2O/SiO2 egg-shell nanocatalysta

|
Entry |
Cat. (mol%) |
Temp (°C) |
Base (eq.) |
Time (h) |
Conv. (%) |
Select. (%) |
Reaction conditions: phenylacetylene (0.50 mmol), benzoyl chloride (0.75 mmol), base Et3N, cat. Cu2O/SiO2 egg-shell nanocatalyst, Ar atmosphere. Determined by using gas chromatography-mass spectrometery (GC-MS). Cat. commercial Cu2O powder purchased from Aldrich (no. 208825). Cat. conventional SiO2-supported Cu2O catalyst. |
1 |
1 |
40 |
3 |
8 |
66 |
99 |
2 |
1.5 |
40 |
3 |
8 |
86 |
98 |
3 |
1 |
80 |
3 |
8 |
93 |
97 |
4 |
1.5 |
80 |
3 |
8 |
100 |
98 |
5 |
1 |
80 |
3 |
12 |
99 |
97 |
6 |
— |
80 |
4 |
12 |
8 |
0 |
7 |
1 |
80 |
— |
12 |
5 |
0 |
8 |
1 |
80 |
4 |
12 |
100 |
99 |
9 |
Recover from #8 |
80 |
4 |
12 |
100 |
99 |
10 |
Recover from #9 |
80 |
4 |
12 |
98 |
99 |
11 |
Recover from #10 |
80 |
4 |
12 |
94 |
99 |
12b |
1 |
80 |
4 |
12 |
76 |
99 |
13c |
1 |
80 |
4 |
12 |
100 |
91 |
14 |
Recover from 13 |
80 |
4 |
12 |
7 |
0 |
Table 2 Substrate study for the coupling reaction of alkynes and acyl chloridesa
Entry |
Acyl chloride |
Alkyne |
Product |
Conv. (%) |
Select. (%) |
Reaction conditions: alkyne (0.5 mmol), acyl chloride (0.75 mmol), Et3N (4.0 equiv.), 1.0 mol% Cu2O/SiO2 egg-shell nanocatalyst, 80 °C, 12.0 h. |
1 |
 |
 |
 |
66 |
100 |
2 |
 |
 |
 |
98 |
99 |
3 |
 |
 |
 |
88 |
87 |
4 |
 |
 |
 |
89 |
98 |
5 |
 |
 |
 |
98 |
85 |
6 |
 |
 |
 |
100 |
100 |
Conclusions
In this paper, egg-shell-type mesoporous silica-supported copper nanoparticle catalyst was prepared. The obtained Cu2O/SiO2 egg-shell nanocatalyst had a large surface area and narrow pore size distribution. In addition, most of the Cu2O nanoparticles, with sizes around 2.0 nm, were highly dispersed in the mesoporous silica. Accordingly, fast reactant diffusion to the active sites would occur, especially when the active metal sites are selectively located on the outer part of the support, i.e., the outer region of the egg shell. As a result, they enhanced the reaction kinetics. In palladium-free, ligand-free and solvent-free Sonogashira reactions for the synthesis of ynones from acyl chlorides and terminal alkynes, this catalyst exhibited a very high catalytic activity. The excellent catalytic performance can be attributed to the synergistic advantages of the mesoporous structure and the monodispersed Cu2O nanoparticles. The egg-shell structure acts as a “nanoreactor framework”, which contains sufficient space and catalytically active surface within its structure. The egg-shell nanoparticles have potential for application as nanoreactors and catalysts, drug delivery carriers, and surface-enhanced Raman scattering substrates.
Conflicts of interest
There are no conflicts to declare.
Acknowledgements
This research was supported by Basic Science Research Program through the National Research Foundation of Korea (NRF) and the Research and Development Program of the Korea Institute of Energy Research (KIER) (No. B8-2461-01), and funded by the Ministry of Science, ICT & Future Planning (NRF-2017R1A4A1015533 and NRF-2017R1D1A1B03036303).
References
- M. J. Albaladejo, F. Alonso, Y. Moglie and M. Yus, Eur. J. Org. Chem., 2012, 2012(16), 3093–3104 CrossRef CAS.
- T. D. Nguyen, C. T. Dinh, D. Mrabet, M. N. Tran-Thi and T. O. Do, J. Colloid Interface Sci., 2013, 394, 100–107 CrossRef CAS PubMed.
- M. L. Kantam, V. S. Jaya, B. Sreedhar, M. M. Rao and B. M. Choudary, J. Mol. Catal. A: Chem., 2006, 256(1–2), 273–277 CrossRef CAS.
- Z. Tai, M. A. Isaacs, C. M. Parlett, A. F. Lee and K. Wilson, Catal. Commun., 2017, 92, 56–60 CrossRef CAS.
- M. V. Zakharova, F. Kleitz and F.-G. Fontaine, Dalton Trans., 2017, 46(12), 3864–3876 RSC.
- J. Ren, P. Hao, W. Sun, R. Shi and S. Liu, Chem. Eng. J., 2017, 328, 673–682 CrossRef CAS.
- Q. Gao, H. T. Li, Y. Ling, B. Han, K. S. Xia and C. G Zhou, Microporous Mesoporous Mater., 2017, 241, 409–417 CrossRef CAS.
- P. Verma, K. Yuan, Y. Kuwahara, K. Mori and H. Yamashita, Appl. Catal., B, 2018, 223, 10–15 CrossRef CAS.
- J. Fang, Y. Zhang, Y. Zhou, S. Zhao, C. Zhang, H. Zhang, X. Sheng and K. Wang, Langmuir, 2017, 33(11), 2698–2708 CrossRef CAS PubMed.
- Y. Han, B. Wen and M. Zhu, Catalysts, 2017, 7(1), 21–31 CrossRef.
- X. Zhang, Y. Zhang, X. Zhang, S. Li and Y. Huang, J. Hazard. Mater., 2017, 337, 1–9 CrossRef CAS PubMed.
- S. A. Gardezi, J. T. Wolan and B. Joseph, Appl. Catal., A, 2012, 447, 151–163 CrossRef.
- Y. Zhuang, M. Claeys and E. Van Steen, Appl. Catal., A, 2006, 301(1), 138–142 CrossRef CAS.
- J. Kolena, G. Šťávová, P. Morávek and K. Štěpánek, Univers. J. Chem., 2017, 5(1), 19–28 Search PubMed.
- E. H. Cho, K. Y. Koo, H. W. Lee, Y.-K. Park, W. L. Yoon and C. H. Ko, Int. J. Hydrogen Energy, 2017, 42, 18350–18357 CrossRef CAS.
- M.-S. Jang, E. H. Cho, K. Y. Koo, W. L. Yoon and C. H. Ko, Appl. Catal., A, 2017, 530, 211–216 CrossRef CAS.
- S. A. Gardezi, L. Landrigan, B. Joseph and J. T. Wolan, Ind. Eng. Chem. Res., 2011, 51(4), 1703–1712 CrossRef.
- E. Peluso, C. Galarraga and H. De Lasa, Chem. Eng. Sci., 2001, 56(4), 1239–1245 CrossRef CAS.
- K. Larmier, S. Tada, A. Comas-Vives and C. Copéret, J. Phys. Chem. Lett., 2016, 7(16), 3259–3263 CrossRef CAS PubMed.
- M. B. Gawande, A. Goswami, F.-X. Felpin, T. Asefa, X. Huang, R. Silva, X. Zou, R. Zboril and R. S. Varma, Chem. Rev., 2016, 116(6), 3722–3811 CrossRef CAS PubMed.
- M. R. Decan, S. Impellizzeri, M. L. Marin and J. C. Scaiano, Nat. Commun., 2014, 5, 4612 CAS.
- H. Cheng, J. Wen and C. Bolm, Chem.–Eur. J., 2017, 23, 12100–12103 CrossRef CAS PubMed.
- M. Sengoden, A. Bhowmick and T. Punniyamurthy, Org. Lett., 2016, 19(1), 158–161 CrossRef PubMed.
- L. Mao, K. l. n. J. Szabó and T. B. Marder, Org. Lett., 2017, 19(5), 1204–1207 CrossRef CAS PubMed.
- S. Thapa, P. Basnet and R. Giri, J. Am. Chem. Soc., 2017, 139(16), 5700–5703 CrossRef CAS PubMed.
- R. Chinchilla and C. Nájera, Chem. Rev., 2007, 107(3), 874–922 CrossRef CAS PubMed.
- D. B. Grotjahn, S. Van, D. Combs, D. A. Lev, C. Schneider, M. Rideout, C. Meyer, G. Hernandez and L. Mejorado, J. Org. Chem., 2002, 67(26), 9200–9209 CrossRef CAS PubMed.
- J. P. Waldo and R. C. Larock, J. Org. Chem., 2007, 72(25), 9643–9647 CrossRef CAS PubMed.
- Z. Wang, L. Li and Y. Huang, J. Am. Chem. Soc., 2014, 136(35), 12233–12236 CrossRef CAS PubMed.
- B. M. Trost and A. Quintard, Org. Lett., 2012, 14(17), 4698–4700 CrossRef CAS PubMed.
- M. Bakherad, A. Keivanloo and S. Samangooei, Tetrahedron Lett., 2012, 53(43), 5773–5776 CrossRef CAS.
- P.-W. Xiao, L. Zhao, Z.-Y. Sui and B.-H. Han, Langmuir, 2017, 33(24), 6038–6045 CrossRef CAS PubMed.
- K. Wang, L. Yang, W. Zhao, L. Cao, Z. Sun and F. Zhang, Green Chem., 2017, 19, 1949–1957 RSC.
- W. Sun, Y. Wang, X. Wu and X. Yao, Green Chem., 2013, 15(9), 2356–2360 RSC.
- H. Lang, A. Jakob and B. Milde, Organometallics, 2012, 31, 7661–7693 CrossRef CAS.
- J. Santandrea, A.-C. Bédard and S. K. Collins, Org. Lett., 2014, 16, 3892–3895 CrossRef CAS PubMed.
- Z. Zhang, J. C. Jung and N. Yan, Nanoscale, 2016, 8, 19684–19695 RSC.
- X. Yan, J. Yang, L. Ma, X. Tong, Y. Wang, G. Jin and X.-Y. J. Guo, J. Solid State Electrochem., 2015, 19, 3195–3199 CrossRef CAS.
- J. Zhang, Y. Wang, C. Yu, X. Shu, L. Jiang, J. Cui, Z. Chen, T. Xiee and Y. Wu, New J. Chem., 2014, 38, 4975–4984 RSC.
- W. Yin, R. Liu, G. He, W. Lv and H. Zhu, RSC Adv., 2014, 4, 37773–37778 RSC.
- M. A. Bhosale, T. Sasakib and B. M. Bhanage, Catal. Sci. Technol., 2014, 4, 4274–4280 CAS.
- H. Yuan, Y. Shen, S. Yu, L. Shan, Q. Sun and W. Zhang, Synth. Commun., 2013, 43, 2817–2823 CrossRef CAS.
Footnote |
† Electronic supplementary information (ESI) available. See DOI: 10.1039/c7ra13490h |
|
This journal is © The Royal Society of Chemistry 2018 |