DOI:
10.1039/C6TC04613D
(Communication)
J. Mater. Chem. C, 2017,
5, 47-53
A strategy to improve the thermoelectric performance of conducting polymer nanostructures†
Received
25th October 2016
, Accepted 28th November 2016
First published on 29th November 2016
Abstract
Although organic thermoelectric materials have witnessed a rapid progress in recent years, polymer nanostructured thermoelectric materials have received little attention. Here, we report the strong dependence of thermoelectric performance on post-treatments (acid treatment with H2SO4 and chemical reduction using Na2SO3) for the poly(3,4-ethylenedioxythiophene) nanostructure.
Introduction
In recent years, organic thermoelectric materials, including conducting polymers and their composites, have received significant attention and witnessed a rapid progress. Versatile distinctive advantages were identified when compared to most of the conventional inorganic thermoelectric counterparts, such as low thermal conductivity (κ), light-weight, high flexibility, solution processability, low cost and adjustable molecular structures.1–8 So far, the main conducting polymers that have been successfully applied in organic thermoelectric materials include poly(3,4-ethylenedioxythiophene) (PEDOT),9–13 polyaniline (PANI),14–17 polypyrrole (PPy),18–22etc. In addition, by judicious combination with inorganic nanoparticles such as graphene nanosheets or carbon nanotubes (CNTs), the thermoelectric performance of the conducting polymers can achieve a significant enhancement.1–4 The thermoelectric performance of inorganic materials is generally evaluated by a dimensionless thermoelectric figure of merit (ZT), ZT = S2σT/κ, where S, σ and T are the thermopower or Seebeck coefficient, electrical conductivity and absolute temperature, respectively. Because organic polymer materials exhibit low intrinsic thermal conductivity (0.1–0.5 W m−1 K−1), their thermoelectric performance has always been characterized by the power factor (S2σ) instead of ZT.9–22
Morphology tuning has been reported as an effective strategy to greatly improve the thermoelectric performance of both neat conducting polymers and their corresponding composites.1–8 Enwrapping of conducting polymers on the surfaces of inorganic nanoparticles is perhaps the most extensively investigated method to date.2 By surface coating, a series of composites with remarkably enhanced thermoelectric performance, such as PEDOT/graphene,9,10 PEDOT/carbon nanotubes (CNTs),11 PANI/graphene,14–16 PANI/CNTs,17 PPy/graphene,18 PPy/CNTs,19,20 and poly-Schiff base/CNTs,21 have been successfully obtained. Very recently, we reported a convenient tuning of thermoelectric performance by means of PEDOT22 or PPy23 nanostructure evolution. Interestingly, both the electrical conductivity and the Seebeck coefficient are strongly dependent on the polymer nanostructures. In addition, we have developed an interfacial adsorption-soft template polymerization strategy to construct a three-dimensional (3D) network morphology composed of PPy nanowires adsorbed on the graphene nanosheet surfaces, which exhibited excellent thermoelectric properties.24 The layered morphology composed of CNT nanosheets with adjacent PPy nanowires shows the largest power factor for PPy and its composites so far.25 However, except the dependence of thermoelectric performance on polymer nanostructures, many important issues are still unaddressed. The understandings of the conduction mechanism for polymer nanostructures are far from clear. For example, whether the thermoelectric performance can be further adjusted or enhanced by post-treatments of polymer nanostructures such as acid treatment and chemical reduction?
In this work, we report the strong dependence of thermoelectric performance on post-treatments for the PEDOT nanostructure. Two post-treatment procedures including acid treatment and chemical reduction were employed, and PEDOT nanorods were used. First, the morphology and thermoelectric performance of the PEDOT nanorods prepared by aqueous and reverse micro-emulsion polymerization methods were compared with field-emission scanning electron microscopy (FESEM) images. Then, the effects of the concentration of sulfuric acid (H2SO4) and the treatment time of sodium sulfate (Na2SO3) on the thermoelectric properties were investigated. Finally, the molecular mechanism for the post-treatments of the PEDOT nanorods was studied and is discussed using X-ray photoemission spectra (XPS), Raman spectra, powder X-ray diffraction (XRD) patterns and ultraviolet-visible-near infrared (UV-Vis-NIR) spectra.
Results and discussion
Comparisons of the morphology and thermoelectric performance of PEDOT nanorods prepared by aqueous and reverse micro-emulsion polymerization
Fig. 1 shows the FESEM images of the PEDOT nanorods polymerized in (a) an aqueous surfactant solution or (b) a reverse micro-emulsion. The nanorod morphology is distinctly different between (a) and (b). The nanorods prepared in the water-phase are much smaller in both the lengths and the widths than those prepared by reverse phase micro-emulsion polymerization. The average length and width of the nanorods polymerized in the water-phase were about 142.4 ± 15.8 nm and 30.5 ± 4.9 nm, respectively. In contrast, the nanorods polymerized in the reverse micro-emulsion had large dimensions of 282.8 ± 26.3 nm in length and 80.3 ± 16.0 nm in width. Therefore, although both methods can afford PEDOT nanorods, large particles in both the length and the width were obtained by the reverse-phase polymerization method, while short and thin nanorods were obtained through the aqueous phase polymerization procedure. In addition, the nanorods obtained by aqueous-phase polymerization are indeed composites, composed of PEDOT and single crystalline β-akaganeite,26 due to the concurrence of EDOT polymerization and FeCl3 hydrolyzation. The result can also be confirmed by our XRD measurement (Fig. S1, ESI†). Note that β-akaganeite exhibits no thermoelectric performance. In contrast, the nanorods made by reverse-phase polymerization are neat polymers. The deviances in both the nanoparticle morphology and constituents may lead to the distinct difference in the thermoelectric performance between the two PEDOT nanorods.
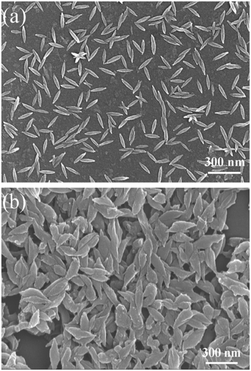 |
| Fig. 1 FESEM images of the PEDOT nanorods prepared via (a) water-phase and (b) reverse-phase micro-emulsion polymerization routes. | |
Moreover, the thermoelectric performance of the PEDOT nanorods prepared by the two polymerization methods is compared in Table 1. The nanorods polymerized in the aqueous phase exhibited a low thermoelectric performance with an electrical conductivity and a Seebeck coefficient of 0.02 S cm−1 and 7.11 μV K−1, respectively. In contrast, the sample prepared by reverse micro-emulsion polymerization exhibited a high electrical conductivity of 8.75 S cm−1 and a large Seebeck coefficient of 16.2 μV K−1. As a consequence, the power factor for the nanorods polymerized in the reverse-phase micro-emulsion (0.23 μW m−1 K−2) was much higher than that polymerized in water (1.01 × 10−4 μW m−1 K−2). Taking into account the above FESEM images and the thermoelectric performances, we conclude that the reverse-phase micro-emulsion method is better than the aqueous surfactant solution polymerization to obtain large nanorods with high thermoelectric performance. Therefore, we adopted the PEDOT nanorods prepared by the reverse-phase polymerization to carry out the following post-treatment studies.
Table 1 Thermoelectric performance of PEDOT nanorods prepared using the aqueous surfactant solution or reverse micro-emulsion polymerization method
|
Aqueous phase |
Reverse micro-emulsion |
Electrical conductivity (S cm−1) |
0.02 |
8.75 |
Seebeck coefficient (μV K−1) |
7.11 |
16.2 |
Power factor (μW m−1 K−2) |
1.01 × 10−4 |
0.23 |
PEDOT nanorod preparation and post-treatment procedures
Fig. 2 shows the preparation route of the PEDOT nanorods and the subsequent post-treatments. The details of the preparation procedure are provided in the ESI.† In brief, the total process can be divided into three steps. First, with the help of the oxidation agent FeCl3, the monomers of EDOT were polymerized inside cylindrical reverse-phase micelles in hexane. The resultant PEDOT nanorods were obtained after washing and drying. Then, the nanorods were post-treated with H2SO4 to study the effect of acid post-treatment on the structure and thermoelectric performance. Finally, a subsequent chemical reduction post-treatment with Na2SO3 was conducted to further enhance the thermoelectric function.
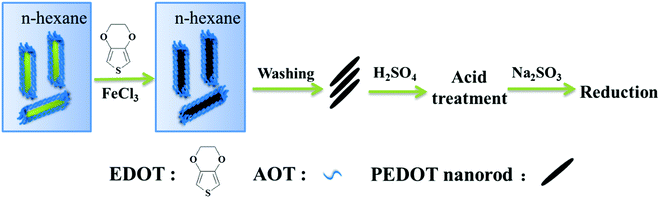 |
| Fig. 2 Schematic illustration of the preparation route of PDOT nanorods obtained by reverse-phase micro-emulsion polymerization, acid post-treatment with H2SO4, and further chemical reduction post-treatment with Na2SO3. | |
Effects of post-treatments on the thermoelectric performance of PEDOT nanorods
In order to shed light on the dependence of the thermoelectric performance on the post-treatments for PEDOT nanostructures, the effects of acid treatment with H2SO4 and its concentration were studied first. As shown in Fig. 3, the acid treatment with H2SO4 adversely affected the electrical conductivity and the Seebeck coefficient in the H2SO4 concentration range of less than 2.0 M. Compared with the pristine PEDOT nanorods before treatment, the electrical conductivity increased significantly, whereas the Seebeck coefficient decreased monotonically. Furthermore, the concentration of H2SO4 had a remarkable impact on the thermoelectric performance. With the increase of the H2SO4 concentration, the electrical conductivity dramatically increased from 8.75 S cm−1 to 41.2 S cm−1, while the Seebeck coefficient simultaneously decreased from 16.2 μV K−1 to 8.55 μV K−1. As a result, the power factor decreased to a small extent first, then dramatically increased, and finally decreased. When the H2SO4 concentration was 1.5 M, the power factor reached a maximum of approximately 0.37 μW m−1 K−2.
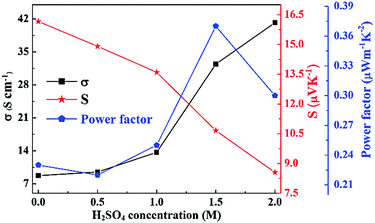 |
| Fig. 3 Effects of H2SO4 concentration on the thermoelectric performance of the acid post-treated PEDOT nanorods. | |
Upon acid post-treatment of the PEDOT nanorods using 1.5 M H2SO4, the nanorods endured a subsequent chemical reduction by Na2SO3 post-treatment. Fig. 4 shows the thermoelectric performance of PEDOT nanorod samples after the chemical reduction treatment with Na2SO3. In sharp contrast to the above acid treatment, the Na2SO3 reduction treatment had a different impact on the thermoelectric performance, namely, the electrical conductivity decreased, while the Seebeck coefficient enhanced. Moreover, with the prolonged Na2SO3 post-treatment period, the electrical conductivity decreased greatly from 32.4 S cm−1 to 0.71 S cm−1. Interestingly, the Seebeck coefficient simultaneously increased dramatically from 10.7 μV K−1 to 35.7 μV K−1. As a whole, the power factor presented an initial increase and a subsequent reduction with the treatment time. When the PEDOT nanorods were treated with Na2SO3 for 10 s, the power factor reached the maximum, i.e. 0.91 μW m−1 K−2, about 4.0 times that of the pristine PEDOT nanorods before any treatment (0.23 μW m−1 K−2). The corresponding electrical conductivity and Seebeck coefficient were 16.9 S cm−1 and 23.2 μV K−1, respectively.
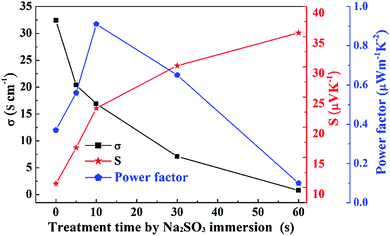 |
| Fig. 4 Effects of Na2SO3 treatment time on the thermoelectric performance of the PEDOT nanorods after 1.5 M H2SO4 treatment and subsequent chemical reduction post-treatment with Na2SO3. | |
Furthermore, the maximum values of the thermoelectric performance measured in this study are compared with the corresponding data for PEDOT nanorods in the previous publications in Table 2. Please note that most of the previous works show only the electrical conductivity, neither the Seebeck coefficient nor the power factor are reported.27–30 The maximum electrical conductivity in this work is only less than the as-prepared PEDOT nanorods prepared by the reverse microemulsion method reported in ref. 28. In addition, the electrical conductivity (16.9 S cm−1) and the Seebeck coefficient (23.2 μV K−1) in this study are greater than and comparable to the corresponding values in our previous work (10.5 S cm−1 and 23.1 μV K−1),22 respectively. Thus, the thermoelectric power factor is 0.91 μW m−1 K−2, and is the highest for the PEDOT nanorods reported to date.
Table 2 Comparison of the thermoelectric properties of the PEDOT nanorods with others reported
Preparation method |
σ (S cm−1) |
S (μV K−1) |
PF = S2σ (μW m−1 K−2) |
Ref. |
Reverse microemulsion |
2–3 |
— |
— |
27
|
Reverse microemulsion |
72 |
— |
— |
28
|
Treated with NH4OH |
10−2 |
— |
— |
28
|
Redoping with HCl |
30 |
— |
— |
28
|
Aqueous solution |
1.96 × 10−2 |
— |
— |
29
|
Reverse microemulsion |
10.5 |
23.1 |
0.56 |
22
|
Liquid crystalline template assisted polymerization |
2.79 |
— |
— |
30
|
Reverse microemulsion |
16.9 |
23.2 |
0.91 |
Present work |
XPS spectra
Doping and oxidation are vital to the electrical conductivity and the Seebeck coefficient for conducting polymers.31 In order to gain deep insights into the conduction mechanism of the carriers, the levels of oxidation and doping were quantitatively measured using XPS spectra according to previous publications.24,32 First, the XPS spectra showed the presence of C, O, S, Cl and Fe in all the samples. The doping agents were mainly the counter ions of bis(2-ethylhexyl) sulfosuccinate (AOT−) and chloride (Cl−) for the PEDOT nanorods. As for the post-treated PEDOT nanorods, the doping ions may also include SO42− (H2SO4 treated) and SO32− anions (Na2SO3 treated), besides the AOT− and Cl− anions. Furthermore, as shown in the S(2p) spectra of Fig. 5, the three main peaks can be de-convoluted using the peak deconvolution software into three or four doublets, namely the neutral PEDOT (∼163.6 and 164.7 eV), the oxidized PEDOT (∼165.1 and 166.2 eV) and the S atoms from other contributions including AOT− surfactants, and SO42− and SO32− anions (∼167.9 and 169.1 eV, ∼169.0 and 170.3 eV), respectively. On the basis of the corresponding peak areas after deconvolution, the doping level and oxidation level per monomer unit were calculated accordingly. The doping levels for the pristine PEDOT nanostructures before treatment, the H2SO4 treated PEDOT, and the Na2SO3 treated PEDOT can be calculated using (AS-AOT + ACl)/(ASN + ASO), (AS-AOT + ACl + AS-SO4)/(ASN + ASO) and (AS-AOT + ACl + AS-SO4 + AS-SO3)/(ASN + ASO), respectively, where AS-AOT, ACl, AS-SO4, AS-SO3, ASO and ASN are the atomic ratios of sulfonate anions from AOT−, chlorine atoms from Cl− anions, sulphur atoms from SO42−, sulphur atoms from SO32−, neutral PEDOT and oxidized PEDOT, respectively. On the other hand, the oxidation levels for all of the PEDOT samples were determined using (ASO/(ASN + ASO)).
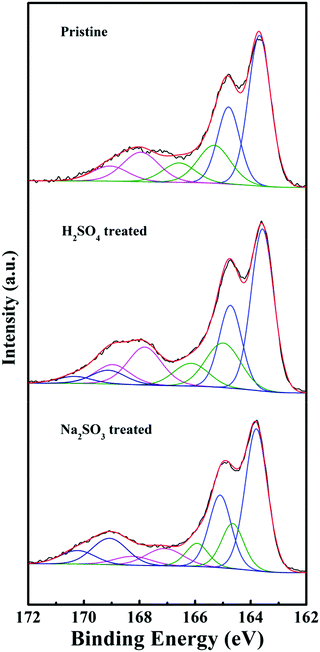 |
| Fig. 5 XPS spectra of the pristine PEDOT nanorods and the post-treated PEDOT samples, including the acid treatment (H2SO4) and the chemical reduction of the above acid-treated PEDOT nanorods by Na2SO3, respectively. | |
The measured values of the levels of doping and oxidation for the pristine PEDOT nanorods before any post-treatment were 0.32 and 0.27, respectively. The data were similar to our previous results obtained for the PEDOT nanorods.24 The H2SO4 treated PEDOT samples displayed increased levels of doping (0.44) and oxidation (0.30). Generally, the acid treatment of PEDOT with H2SO4 leads to an increase in the doping level and the conformational change of macromolecular chains.33,34 Theoretically, a high doping level always results in high electrical conductivity due to the increased carrier concentrations. As a result, after the acid treatment, an enhancement in electrical conductivity was detected, as shown in Fig. 3. In addition, the H2SO4 post-treatment resulted in the conformational transition from coiled arrangement to extended conjugated chains, as shown in Fig. S2 (ESI†) which provided a high mobility path for the carrier transport. On the other hand, the oxidation level was also improved after the acid post-treatment. The reason might be attributed to the generation of charged carriers during the multistage oxidation of polymer chains for chemical p-type doping, which reduced the Seebeck coefficient of PEDOT.
On the other hand, the Na2SO3 treated PEDOT nanorods revealed reductions in both the doping level (0.38) and the oxidation level (0.24). Consequently, the electrical conductivity decreased with the simultaneous enhancements of the Seebeck coefficient. The conformational transition, molecularly ordered alignment and the carrier types are discussed using Raman spectra, XRD patterns and UV-Vis-NIR spectra in the following.
Raman spectra
Raman spectroscopy is a powerful tool to study the doping behaviour of conjugated polymers. Fig. 6 shows the Raman spectra of the pristine PEDOT nanorods, the H2SO4 treated PEDOT nanorods, and the subsequent Na2SO3 post-treated PEDOT nanorods. The bands at 1265 cm−1 and 1365 cm−1 were characteristic of C–C inter-ring stretching and C–C stretching vibrations, respectively. In addition, the strong bands at 1432 (C
C symmetrical stretching mode of the five-membered thiophene ring) and 1510 cm−1 (C
C asymmetrical stretching) are evident.35 Here, the strongest band at 1432 cm−1 was chosen to study the doping/de-doping state. Distinctly, the characteristic band at 1432 cm−1 for the pristine PEDOT nanorods shifted to a higher wavenumber at 1434 cm−1 for the H2SO4 post-treated PEDOT, suggesting the increased doping of PEDOT by bisulfate anions upon H2SO4 post-treatment.33,36 Thus, the acid post-treatment aided the increase of the doping level in PEDOT and thus increased the electrical conductivity.29,32 In addition, after the chemical reduction post-treatment by Na2SO3, the band shifted reversely from 1434 to 1432 cm−1. This indicates a reverse change in the de-doping process. These results are in agreement with the above XPS spectra and the measured thermoelectric performance.
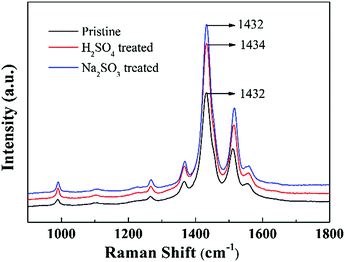 |
| Fig. 6 Raman spectra of the pristine PEDOT nanorods and the PEDOT nanorod samples post-treated with H2SO4 and Na2SO3, respectively. | |
XRD patterns
In order to shed light on the ordered structure of a macromolecular arrangement, the XRD technique was employed. Fig. 7 presents the XRD patterns of the pristine PEDOT nanorods and the post-treated ones. Distinctly, the pristine PEDOT nanorods exhibited a weak peak at around 2θ = 6.6° and a broad diffraction peak with the maximum at approximately 25.6°. The peak at 6.6° corresponded to the interchain ring-packing along an orthorhombic a-axis, while the peak at 25.6° was characteristic of the disordered structure of PEDOT macromolecular chains.37 The pattern for the pristine PEDOT nanorods coincides with our previous study20 very well. Besides these two major diffraction peaks, the post-treated samples showed a new pattern at 2θ = 12.2°, which is the (200) plane reflections of the (100) plane (2θ = 6.6°). In other words, the degrees of the ordered alignment for the post-treated samples were higher than those of the pristine PEDOT nanorods, as shown in Fig. S2 (ESI†). Furthermore, the structural ordering was enhanced with the concentration of the H2SO4 for the acid treated PEDOT nanorods due to the increased intensities of the characteristic peaks for the ordered arrangements.
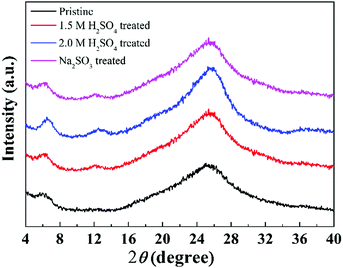 |
| Fig. 7 XRD patterns of the pristine PEDOT nanorods and the PEDOT nanorod samples post-treated with H2SO4 and Na2SO3, respectively. | |
UV-Vis-NIR spectra
It is widely accepted that there are three main types of PEDOT redox states, depending on oxidation levels, namely PEDOT2+ (bipolarons or dications), PEDOT+ (polarons or radical cations) and PEDOT (neutral chains). Fig. 8 clearly depicts the chemical structures of these three different carriers.26,38 Importantly, the bipolarons can be reduced to polarons, and further reduced to neutral chains. In addition, the bipolarons have the highest level of oxidation, while the neutral chains have the largest Seebeck coefficient. Thus, the Seebeck coefficient and the thermoelectric performance of PEDOT can be conveniently and effectively tuned by adjusting the level of oxidation.
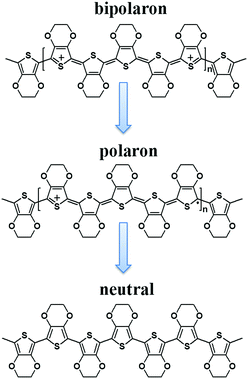 |
| Fig. 8 Chemical structures of charge and neutral carriers for PEDOT, including a bipolaron, a polaron, and a neutral chain. | |
UV-Vis-NIR spectra reveal a qualitative evaluation of the concentration of neutral and charge carriers in polythiophenes (PTh) including PEDOT.39 In order to investigate the types and transition for the carriers, UV-Vis-NIR absorption spectra were collected and are shown in Fig. 9. Pristine PEDOT nanorods exhibited a broad band with strong absorption in the NIR region, suggesting that the main carriers for the pristine PEDOT nanorods were the dipolarons with the lowest Seebeck coefficient relative to the other two carrier types. After chemical reduction by Na2SO3 treatment, a peak centred around ∼580 nm appeared gradually with the elongation of chemical reduction treatment time. In general, the neutral polymer chains for PEDOT reveal a characteristic band around 580–600 nm. In addition, the absorbance around 900 nm, characteristic of polarons, strengthened simultaneously after the Na2SO3 treatments. Therefore, the UV-Vis-NIR spectra provide proof that the chemical reduction by Na2SO3 treatment led to carrier type transitions from bipolarons to polarons and even to neutral chains. With the elongation of the treatment time, the concentrations of the neutral chains and the polarons increased, while the doping level of the bipolarons decreased in the meantime.
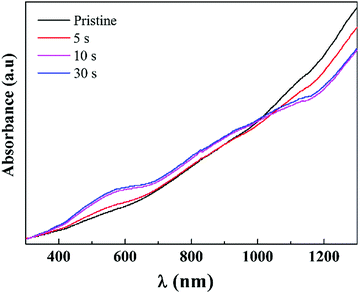 |
| Fig. 9 UV-Vis-NIR spectra of the pristine PEDOT nanorods and the PEDOT samples post-treated with Na2SO3 after a certain period. | |
Because the neutral chains have the largest Seebeck coefficient, the transition of bipolarons → polarons → neutral chains resulted in the enhancement of the Seebeck coefficient, as shown in Fig. 4. On the other hand, the reduction of the doping levels due to the above transition resulted in the decrease of the electrical conductivity.
Conclusions
We proposed the strong dependence of the thermoelectric performance on post-treatments for the PEDOT nanostructure, using both acid and chemical reduction. The PEDOT nanorods prepared by reverse micro-emulsion polymerization, exhibiting better thermoelectric properties than those prepared by aqueous polymerization, were employed in the post-treatments. The H2SO4 post-treatment of PEDOT nanorods resulted in increased electrical conductivity and a reduced Seebeck coefficient, while the chemical reduction by Na2SO3 led to reversed effects. The optimum concentration of H2SO4 and the time of Na2SO3 immersion were 1.5 M and 10 s, respectively. And the maximum power factor was ∼4.0 times that of the pristine PEDOT nanorods. The H2SO4 treatment increased the levels of both doping and oxidation, whereas the Na2SO3 post-treatment reduced both of them. The acid post-treatment benefited the formation of ordered arrangements of PEDOT macromolecular chains. In addition, the chemical reduction by Na2SO3 obviously resulted in the carrier transition of bipolarons → polarons → neutral chains. Therefore, by judicious post-treatments with acid and by chemical reduction, the levels of doping and oxidation, the carrier type transition as well as the chain ordered alignment structure can be adjusted. Thus, the thermoelectric performance of PEDOT nanorods can be conveniently and effectively tuned. It is reasonable to expand this study to other conjugated polymer materials with diverse nanostructures, which will widen and speed up the research of organic thermoelectric materials.
Acknowledgements
We acknowledge the financial support from the National Natural Science Foundation of China (No. 51573190, 51343005 and 51373008).
References
- O. Bubnova, Z. U. Khan, H. Wang, S. Braun, D. R. Evans, M. Fabretto, P. Hojati-Talemi, D. Dagnelund, J.-B. Arlin, Y. H. Geerts, S. Desbief, D. W. Breiby, J. W. Andreasen, R. Lazzaroni, W. M. Chen, M. Zozoulenko, M. Fahlman, P. J. Murphy, M. Berggren and X. Crispin, Nat. Mater., 2014, 13, 190 CrossRef CAS PubMed
.
- M. L. Chabinyc, Nat. Mater., 2014, 13, 119 CrossRef CAS PubMed
.
- C. Gao and G. Chen, Compos. Sci. Technol., 2016, 124, 52 CrossRef CAS
.
- A. M. Glaudell, J. E. Cochran, S. N. Patel and M. L. Chabinyc, Adv. Energy Mater., 2015, 5, 1401072 CrossRef
.
- J. Xie, C.-E. Zhao, Z.-Q. Lin, P.-Y. Gu and Q. Zhang, Chem. – Asian J., 2016, 11, 1489 CrossRef CAS PubMed
.
- W. Zhao, S. Fan, N. Xiao, D. Liu, Y. Y. Tay, C. Yu, D. Sim, H. H. Hng, Q. Zhang, F. Boey, J. Ma, X. Zhao, H. Zhang and Q. Yan, Energy Environ. Sci., 2012, 5, 5364 CAS
.
- J. Wu, Y. Sun, W.-B. Pei, L. Huang, W. Xu and Q. Zhang, Synth. Met., 2014, 196, 173 CrossRef CAS
.
- J. Wu, Y. Sun, W. Xu and Q. Zhang, Synth. Met., 2014, 189, 177 CrossRef CAS
.
- K. Xu, G. Chen and D. Qiu, J. Mater. Chem. A, 2013, 1, 12395 CAS
.
- K. Xu, G. Chen and D. Qiu, Chem. – Asian J., 2015, 10, 1225 CrossRef CAS PubMed
.
- Z. Zhang, G. Chen, H. Wang and X. Li, Chem. – Asian J., 2015, 10, 149 CrossRef CAS PubMed
.
- J. Wang, K. Cai and S. Shen, Org. Electron., 2015, 17, 151 CrossRef CAS
.
- Y. Du, K. Cai, S. Chen, H. Wang, S. Z. Shen, R. Donelson and T. Lin, Sci. Rep., 2015, 5, 6411 CrossRef CAS PubMed
.
- C. Cho, B. Stevens, J.-H. Hsu, R. Bureau, D. A. Hagen, O. Regev, C. Yu and J. C. Grunlun, Adv. Mater., 2015, 27, 2996 CrossRef CAS PubMed
.
- L. Wang, Q. Yao, H. Bi, F. Huang, Q. Wang and L. Chen, J. Mater. Chem. A, 2015, 3, 7086 CAS
.
- K. Xu, C. Gao, G. Chen and D. Qiu, Org. Electron., 2016, 31, 41 CrossRef CAS
.
- J. Liu, J. Sun and L. Gao, Nanoscale, 2011, 3, 3616 RSC
.
- L. Wang, F. Liu, C. Jin, T. Zhang and Q. Yin, RSC Adv., 2014, 4, 46187 RSC
.
- M. Culebras, B. Riol, C. M. Gómez and A. Cantarero, Phys. Chem. Chem. Phys., 2015, 17, 15140 RSC
.
- L. Liang, C. Gao, G. Chen and C.-Y. Guo, J. Mater. Chem. C, 2016, 4, 526 RSC
.
- C. Gao and G. Chen, J. Mater. Chem. A, 2016, 4, 11299 CAS
.
- X. Hu, G. Chen, X. Wang and H. Wang, J. Mater. Chem. A, 2015, 3, 20896 CAS
.
- L. Liang, G. Chen and C.-Y. Guo, Mater. Chem. Front., 2017, 1 10.1039/c6qm00061d
.
- Z. Zhang, G. Chen, H. Wang and W. Zhai, J. Mater. Chem. C, 2015, 3, 1649 RSC
.
- L. Liang, C. Gao, G. Chen and C.-Y. Guo, Compos. Sci. Technol., 2016, 129, 130 CrossRef CAS
.
- H. Miao, X. Lu, D. Chao, L. Cui, Y. Li and W. Zhang, J. Phys. Chem. C, 2008, 112, 20469 Search PubMed
.
- Y.-K. Han, M.-Y. Chang, W.-Y. Huang, H.-Y. Pan, K.-S. Ho, T.-H. Hsieh and S.-Y. Pan, J. Electrochem. Soc., 2011, 158, K88 CrossRef CAS
.
- J. Jang, M. Chang and H. Yoon, Adv. Mater., 2005, 17, 1616 CrossRef CAS
.
- H. Mao, X. Lu, D. Chao, L. Cui, Y. Li and W. Zhang, J. Phys. Chem. C, 2008, 112, 20469 CAS
.
- S. J. Devaki, N. K. Sadanandhan, R. Sasi, H. J. P. Adler and A. Pich, J. Mater. Chem. C, 2014, 2, 6991 RSC
.
- O. Bubnova, Z. U. Khan, A. Malti, S. Braun, M. Fahlman, M. Berggren and X. Crispin, Nat. Mater., 2011, 10, 429 CrossRef CAS PubMed
.
- H. Yoon, J.-Y. Hong and J. Jang, Small, 2007, 3, 1774 CrossRef CAS PubMed
.
- J. Wang, K. Cai and S. Shen, Org. Electron., 2014, 15, 3087 CrossRef CAS
.
- N. Kim, S. Kee, S. H. Lee, B. H. Lee, Y. H. Kahng, Y.-R. Jo, B.-J. Kim and K. Lee, Adv. Mater., 2014, 26, 2268 CrossRef CAS PubMed
.
- S. Garreau, G. Louarn, J. P. Buisson, G. Froyer and S. Lefrant, Macromolecules, 1999, 32, 6807 CrossRef CAS
.
- S. R. S. Kumar, N. Kurra and H. N. Alshareef, J. Mater. Chem. C, 2016, 4, 215 RSC
.
- T. Yamamoto, K. Shiraishi, M. Abla, I. Yamaguchi and L. B. Groenendaal, Polymer, 2002, 43, 711 CrossRef CAS
.
- O. Bubnova, M. Berggren and X. Crispin, J. Am. Chem. Soc., 2012, 134, 16645 CrossRef PubMed
.
- K. Jeuris, L. Groenendaal, H. Verheyen, F. Louwet and F. De Schryver, Synth. Met., 2003, 132, 289 CrossRef CAS
.
Footnotes |
† Electronic supplementary information (ESI) available: Experimental section and schematic structure of the transition of the PEDOT. See DOI: 10.1039/c6tc04613d |
‡ Contributed equally to this work. |
|
This journal is © The Royal Society of Chemistry 2017 |