DOI:
10.1039/C7RA11056A
(Paper)
RSC Adv., 2017,
7, 53847-53854
Construction of C60-decorated SWCNTs (C60-CNTs)/bismuth-based oxide ternary heterostructures with enhanced photocatalytic activity†
Received
8th October 2017
, Accepted 13th November 2017
First published on 23rd November 2017
Abstract
Novel nanostructured carbon/BiVO4 and nanostructured carbon/Bi2MoO6 nanocomposite photocatalysts were fabricated via a facile hydrothermal process by using fullerene (C60)-decorated single-walled carbon nanotubes (SWCNTs) as the carbon source, which are denoted as C60-CNTs. The fabricated nanocomposites were characterized by various analytical techniques. The results showed that the C60-CNTs are intimately bound to the bismuth-based oxide surfaces. The UV-vis diffuse reflectance spectra of C60-CNTs/bismuth-based oxide nanocomposites exhibited increased visible-light absorption compared to pure bismuth-based oxides. Moreover, the ternary nanocomposites demonstrated significantly enhanced photocatalytic activity for the degradation of rhodamine B (Rh B) under visible light. The enhanced performance is attributed to the extended absorption in the visible-light region resulting from the incorporation of C60-CNTs, high specific surface area, and efficient separation of electron–hole pairs by the ternary composite system. In addition, the radical-trapping experiments revealed that the holes and O2˙− play major roles in the decolorization of Rh B under visible-light irradiation.
1. Introduction
Semiconductor photocatalysis is a promising environmental remediation technology that can be used to treat inorganic or organic pollutants in the environment.1–3 To date, many semiconductor photocatalysts have been studied for use in water splitting and/or environmental remediation.4–6 Among them, bismuth-based semiconductors (e.g., Bi2WO6, BiVO4, Bi4Ti3O12, Bi2O2CO3, BiOI and Bi2MoO6) have attracted great attention due to their many advantages, such as superior photocatalytic performance under UV and visible light irradiation, unique layered structures, resistance to photocorrosion, chemical stability, low/non-toxicity, and abundance in the earth.7–12 Among the various bismuth-based semiconductors, BiVO4 with a band gap of around 2.40 eV is a strong candidate because of its properties that include good photoconductivity and significant visible-light response.13,14 However, some researches have revealed that the photocatalytic activity of pure BiVO4 is comparatively low due to the difficulty in the separation of photogenerated electron–hole pairs.14 Bi2MoO6, with excellent intrinsic physical and chemical properties, exhibits photocatalytic activity for water splitting and organic pollutant degradation.15,16 Nevertheless, pure Bi2MoO6 itself has been a bit of a disappointment as a photocatalyst due to the fast recombination of the photo-induced electrons and holes in the material. Much work is centered on methods that can transform BiVO4 or Bi2MoO6 into a more efficient visible light photocatalytic material.17–19 Among the various methods, the construction of composite photocatalysts has shown to be an effective approach for improving the photocatalytic efficiency for the degradation of organic contaminants.19
In recent years, carbonaceous materials such as activated carbon (AC), carbon dots (CDots), fullerene (C60), carbon nanotubes (CNTs) and graphene have been widely employed for the enhancement of photocatalytic performances of semiconductors, which has thus attracted considerable attention.19,20 Carbon nanostructured materials have been used as coupling materials for bismuth-based composites due to their excellent electron accepting and electron-transporting properties.20 Over the past few years, combination of two kinds of carbon materials such as C60-CNTs, C60-RGO, and GO-CDots as a new class of carbon nanostructured materials has attracted much attention due to their extraordinary electronic, optical, and chemical properties.21,22 Moreover, there are many reports on composites containing SWCNTs and C60 clusters, which are known to decorate the SWCNT sidewall through covalent or noncovalent interaction.21 Although MWCNTs, SWCNTs, and C60 have been widely investigated for coupling with semiconductor materials, studies on the photoactivity of the above-mentioned novel C60-CNTs hybrid carbon materials coupled with bismuth-based oxides are still scarce, and the photocatalytic mechanism of the composite photocatalyst remains unclear.
Herein, we report two novel C60-CNTs/bismuth-based oxide composite photocatalysts fabricated through sensitizing leaf-like BiVO4 and sheet-like Bi2MoO6 with C60-CNTs hybrid carbon materials via a facile hydrothermal method for the first time. The photocatalytic performances of the composites were examined by degrading rhodamine B (Rh B) under visible-light irradiation (λ > 420 nm). The experimental results showed that the as-prepared C60-CNTs/bismuth-based oxide composites exhibited excellent photocatalytic activity. Moreover, the possible photocatalytic mechanism of the composite materials related to the band positions of the semiconductors have also been discussed in detail.
2. Experimental section
2.1 Preparation of photocatalysts
2.1.1 Preparation of C60-CNTs. All reagents for synthesis and analysis were commercially available and used without further treatments. C60-CNTs hybrids were prepared by using the Zhang's method:21 50 mg of SWCNTs and 50 mg of C60 were dispersed by ultrasonication in 100 mL toluene for 1 h, and then stirred at room temperature for 12 h. After volatilization of toluene, the resultant black powder was washed with ethanol for several times and dried in vacuum at 80 °C for 12 h to obtain the C60-decorated SWCNTs (50 wt% C60-CNTs, denoted as C60-CNTs).
2.1.2 Preparation of C60-CNTs/BiVO4 and C60-CNTs/Bi2MoO6. The nanostructured carbon/bismuth-based oxide nanocomposites were prepared by a hydrothermal method. For example, in a typical synthetic process for nanostructured carbon/BiVO4, 15 mg of C60-CNTs was dispersed in 15 mL water by sonication, and Bi(NO3)3·5H2O (0.88 mmol) and NH4VO3 (0.88 mmol) were added to the mixture and stirred for additional 1 h at room temperature. The theoretical weight ratio of C60-CNTs to BiVO4 was 1
:
19 for the nanostructured carbon/BiVO4 (2.5 wt% C60/2.5 wt% CNTs/BiVO4, denoted as C60-CNTs/BiVO4). After carefully adjusting the pH value to 3 using 25 wt% NH3·H2O solution, the resulting mixture was transferred into a 20 mL Teflon-lined stainless steel autoclave and kept at 150 °C for 24 h. The obtained product was collected by centrifugation, washed with water and ethanol several times, and then dried at 80 °C overnight. C60-CNTs/Bi2MoO6 was prepared via a similar process.
2.2 Characterization of photocatalysts
X-ray diffraction (XRD) was carried out on a D/MAX 2500V diffractometer (Rigaku, Japan) with monochromatized Cu Kα radiation, λ = 0.154 18 nm, in the 2θ range of 10 to 70°. The morphologies and microstructures of the products were characterized by transmission electron microscopy (TEM, JEM-2100F). X-ray photoelectron spectroscopy (XPS, VGScientific) using 300 W Al Kα radiation as the excitation source was applied to study the composition and chemical states of the elements. The FT-IR spectra were recorded on an FTIR spectrometer (America Perkin Elmer, Spectrum One) using the standard KBr disk method. UV-vis absorption spectra of the samples were tested on a scan UV-vis spectrophotometer (Shimadzu, UV-2550) equipped with an integrating sphere using BaSO4 as the reference sample. The surface areas were measured by the nitrogen adsorption Brunauer–Emmett–Teller (BET) method (BET/BJH surface area, 3H-2000PS1). The photoluminescence (PL) spectra of the photocatalysts were obtained using a F4500 (Hitachi, Japan) photoluminescence detector with an excitation wavelength of 325 nm.
2.3 Photocatalytic activities studies
The photocatalytic properties of the as-prepared samples were evaluated using Rh B as a model compound. In the experiments, the Rh B solution (0.01 mmol L−1, 100 mL) containing 0.02 g of photocatalyst was mixed in a pyrex reaction glass. The reactivity experiments were carried out in air at room temperature. A 300 W Xe lamp (λ > 420 nm) with 100 mW cm−2 illumination intensity was employed to provide visible-light irradiation. A 420 nm cut-off filter was inserted between the lamp and the sample to filter out UV light (λ < 420 nm). Prior to visible-light illumination, the suspension was strongly stirred in dark for 40 min. The solution was then exposed to visible-light irradiation under magnetic stirring. At specific time intervals, 4 mL of the suspension was periodically collected and analyzed after centrifugation. The Rh B concentration was analyzed using a UV-2550 spectrometer to record the intensity of the maximum band at 552 nm in the UV-vis absorption spectra.
2.4 Active species trapping experiments
To detect the active species during photocatalytic reactivity, some sacrificial agents such as 2-propanol (IPA), disodium ethylenediamine tetraacetic acid (EDTA-2Na), and 1,4-benzoquinone (BQ) were used as scavengers for hydroxyl radical (˙OH), hole (h+), and superoxide radical (O2˙−), respectively. The method was similar to the former photocatalytic activity test with the addition of 1 mmol of quencher in the presence of Rh B.
3. Results and discussion
The XRD patterns of SWCNTs, C60, and C60-CNTs are shown in Fig. 1a. In the diffraction pattern of SWCNTs, an apparent wide peak at 2θ = ∼26° appeared, corresponding to the (002) plane.21 C60 exhibits diffraction peaks corresponding to (111), (220), (311), and (222) planes at 2θ = 10.7°, 17.7°, 20.7°, and 21.7°, which can be readily indexed to a face centered cubic (fcc) structure (JCPDS no. 44-558).21 When the two compounds were coupled, the main characteristic diffraction peaks of SWCNTs and C60 exhibited no obvious change, indicating that C60 clusters in C60-CNTs can form an fcc crystallite. Fig. 1b displays the XRD patterns of the bare Bi2MoO6 and C60-CNTs/Bi2MoO6 composite. All the diffraction peaks in the pattern of Bi2MoO6 can be indexed to the specific crystal planes of the Bi2MoO6 phase (JCPDS no. 21-0102). Besides the peaks of Bi2MoO6, the diffraction peaks of C60 in the pattern of C60-CNTs/Bi2MoO6 can be observed, indicating the coexistence of C60. Doping with SWCNTs did not significantly change the structure of Bi2MoO6. It was reported that SWCNTs show a weak but characteristic diffraction peak at 26.0°. However, this peak was not apparent in the patterns of the as-prepared composites, which could be attributed to the small amount of SWCNTs in the composites. In the C60-CNTs/BiVO4 composite, the diffraction peaks of BiVO4 (JCPDS no. 14-0688) and C60 were detected (Fig. 1c), suggesting the existence of C60 and BiVO4 phases.
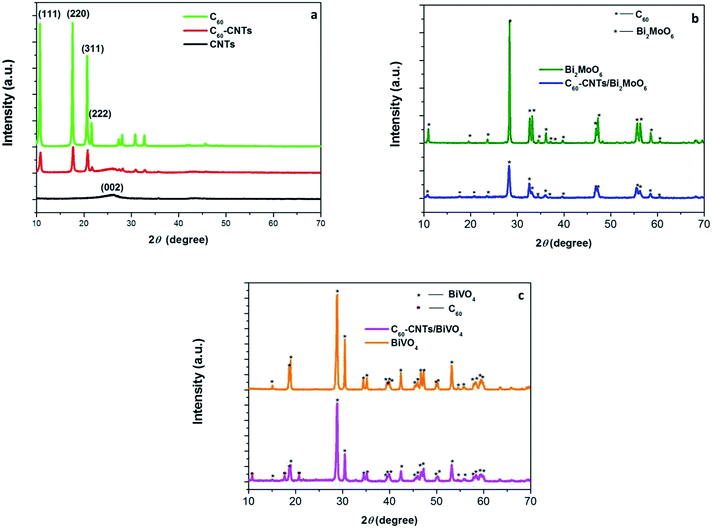 |
| Fig. 1 XRD patterns of SWCNTs, C60 and C60-CNTs (a), Bi2MoO6 and C60-CNTs/Bi2MoO6 (b), and BiVO4 and C60-CNTs/BiVO4 (c). | |
Fig. 2 displays the microstructures of C60-CNTs/BiVO4 and C60-CNTs/Bi2MoO6. Usually, the produced SWCNTs have a tendency to form tight bundles.23,24 C60-CNTs are also made of bundles of entangled SWCNTs. As shown in Fig. 2a, the C60-CNTs are intimately bound to the leaf-like BiVO4 nanostructure surfaces. However, some single or double SWCNTs with an average diameter of ∼10 nm can still be observed (Fig. 2b). C60 molecules with a diameter of ca. 0.7 nm is too small to be directly resolved by HRTEM observation, which is similar to the reported work.21 The lattice fringe pattern of BiVO4 was measured as 0.224 nm (Fig. 2c), consistent with the interplanar spacing of the BiVO4 (121) plane. A similar phenomenon occurred in the C60-CNTs/Bi2MoO6 nanocomposite. As can be seen in Fig. 2d and e the C60-CNTs are intimately bound to the surfaces of the Bi2MoO6 nanosheets. The lattice fringe pattern of Bi2MoO6 was measured as 0.38 nm (Fig. 2f), which is consistent with the interplanar spacing of the Bi2MoO6 (111) plane.
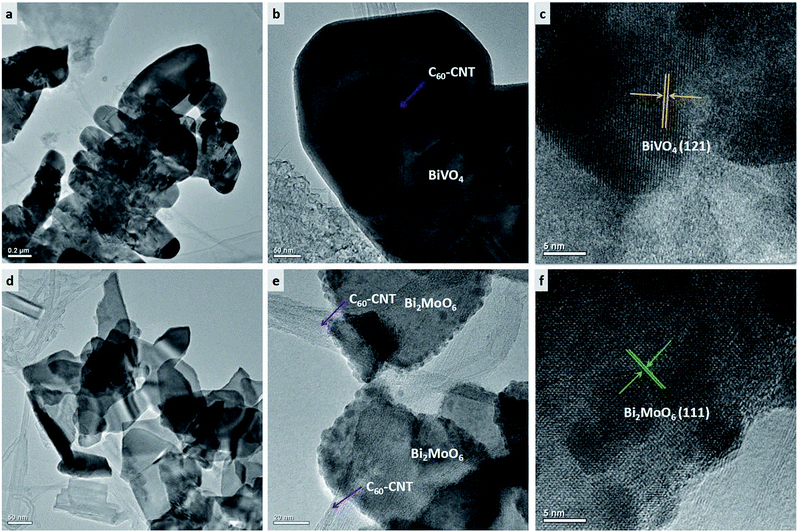 |
| Fig. 2 TEM images of as-prepared samples: (a, b) C60-CNTs/BiVO4, (c) HRTEM image of C60-CNTs/BiVO4, (d, e) C60-CNTs/Bi2MoO6, (f) HRTEM image of C60-CNTs/Bi2MoO6. | |
FTIR spectroscopy was applied to distinguish the microstructures of nanostructured carbons and their composites. Fig. 3a shows the FTIR spectra of C60, CNTs, C60-CNTs, C60-CNTs/Bi2MoO6, and C60-CNTs/BiVO4. The bands at 1182, 1429, and 1630 cm−1 are attributed to the internal modes of the C60 molecule.25 It can be observed that both C60-CNTs/BiVO4 and C60-CNTs/Bi2MoO6 nanocomposites reveal the characteristic bands for C60 molecule, showing the existence of C60-CNTs. The successful loading of C60-CNTs was also illustrated by XPS. Fig. 3b displays the deconvolution of the C 1s peaks of C60-CNTs, C60-CNTs/Bi2MoO6, and C60-CNTs/BiVO4. For C60-CNTs, the main peak at 282.3 eV was assigned to the sp2-hybridized carbon of CNTs, which can be ascribed to the C–C bond of the sp2-hybridized carbon in the graphene sheet. In case of C60-CNTs/Bi2MoO6 and C60-CNTs/BiVO4, the binding energy of C 1s shifted by about 0.2 and 0.5 eV, respectively, toward higher binding energies. A similar phenomenon was observed for Bi 4f (Fig. 3c). This implied the successful incorporation of C60-CNTs into Bi2MoO6 or BiVO4. The spin-orbit of Bi 4f in Bi2MoO6 and BiVO4 could be well deconvoluted as two peaks at around 159 and 164 eV, which correspond to the Bi 4f7/2 and Bi 4f5/2 orbits of Bi3+, respectively.
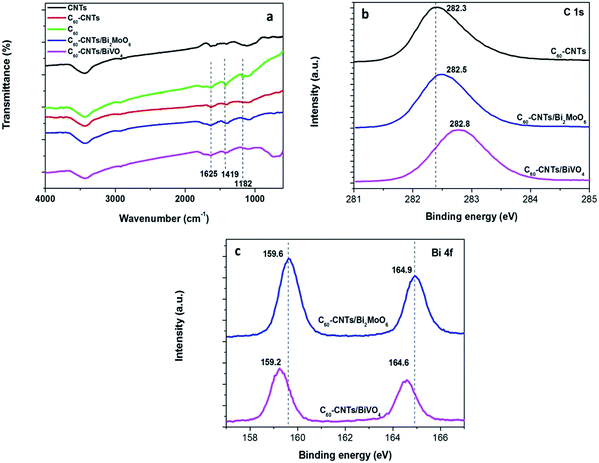 |
| Fig. 3 (a) FTIR of as-prepared samples. XPS spectra of as-obtained samples: (b) C 1s, (c) Bi 4f. | |
Fig. 4a shows the UV-vis absorption spectra of bare Bi2MoO6, BiVO4, C60-CNTs, and C60-CNTs/Bi2MoO6, and C60-CNTs/BiVO4 nanocomposites. All the samples exhibit the typical absorptions with an intense transition in the visible region. In addition, the introduction of nanostructured carbons (C60-CNTs) into the Bi2MoO6 or BiVO4 leads to an increase in absorption in the visible-light region, indicating an intense electronic interaction between the bismuth-based oxide and nanostructured carbon, when C60-CNTs were used as the carbon source.21
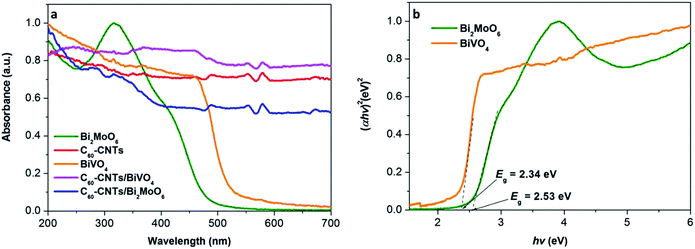 |
| Fig. 4 (a) UV-vis absorption spectra of the as-obtained samples. (b) Plots of (αhv)2 versus photon energy (hν) for the band gap energies of BiVO4 and Bi2MoO6. | |
In general, for a crystalline semiconductor, the optical absorption band edge can be estimated according to the formula, αhv = A(hv − Eg)n/2, where α, v, Eg, and A are the absorption coefficient, light frequency, band-gap energy, and a constant, respectively. Among these parameters, n is determined by the type of optical transition in a semiconductor. The value of n is 1 for direct transition and 4 for indirect transition. For Bi2MoO6 or BiVO4, the value of n is 1 for direct transition, thus, the band gaps were estimated as about 2.34 eV for BiVO4 and 2.53 eV for Bi2MoO6, as shown in Fig. 4b. In addition, the potentials of the valence band (VB) and conduction band (CB) for BiVO4 can be calculated according to the two formulas which are proposed by Butler and Ginley:
Here,
EVB is the valence band edge potential,
ECB is the conduction band edge potential,
X is the electronegativity of the semiconductor, which is the geometric mean of the electronegativity of the constituent atoms,
Ee is the energy of free electrons on the hydrogen scale (about 4.5 eV), and
Eg is the band gap energy of the semiconductor. Based on the above formulas, the VB potential and CB potential of BiVO
4 were calculated as 2.828 eV and 0.488 eV, respectively, whereas the CB and VB edge potentials of Bi
2MoO
6 were −0.26 eV and 2.27 eV, respectively.
The photocatalytic activities of the samples were evaluated by photocatalytic degradation of a Rh B solution under visible light. Adsorption equilibrium was reached for all the examined photocatalysts after stirring for 40 min in dark (Fig. S1†). As can be seen in Fig. 5a, when the solution is irradiated with visible light for 30 min in the absence of any catalyst, little change in Rh B concentration is observed, which is in agreement with previous reports.26,27 This indicates that the self-photodegradation of Rh B is negligible. As shown in Fig. 5a, pure Bi2MoO6 and BiVO4 exhibited generally low photocatalytic activities, with only 43.7% and 74.0% Rh B degradation, respectively, after visible-light irradiation for 30 min. This low performance is possibly due to the fast recombination of photo-induced electrons and holes in the single semiconductor. The results indicate that the photocatalytic activity of C60-CNTs/bismuth-based oxide nanocomposites toward Rh B is much higher than that of either pure Bi2MoO6 or BiVO4. The introduction of C60-CNTs to bismuth-based oxides resulted in significant improvement in the photocatalytic performances of Bi2MoO6 and BiVO4. After 30 min of visible-light irradiation, the photocatalytic degradation percentages of Rh B were about 96.1% and 88.4% for C60-CNTs/BiVO4 and C60-CNTs/Bi2MoO6 composites, respectively. This is because the efficient heterojunction interface between two or three components can restrain the recombination of photo-induced charges effectively.28,29 The C60-CNTs coating can improve the visible-light absorption efficiency (Fig. 4), which is beneficial for the ternary composite in photolyzing Rh B.30,31 Further, the surface areas of the samples were measured by N2 adsorption–desorption isotherms. Fig. 5b shows the N2 adsorption–desorption isotherms at 77 K. The obtained BET specific surface areas for BiVO4, Bi2MoO6, C60-CNTs, C60-CNTs/BiVO4, and C60-CNTs/Bi2MoO6 samples are 20.913, 44.202, 188.426, 146.071, and 179.465 m2 g−1, respectively (Table 1). It can be observed that the BET specific areas increased after the loading of C60-CNTs, which can facilitate more efficient contact of the composite samples with organic contaminants, leading to the enhancement of photocatalytic efficiency.32,33 Because a renewable catalyst is another important criterion for photocatalytic application,34 the stabilities of C60-CNT/Bi2MoO6 and C60-CNTs/BiVO4 composites were investigated by a recycling test (Fig. 5c). After four cycles, there was no significant loss of activity, which indicated that the composite photocatalysts were stable during the photocatalytic test.
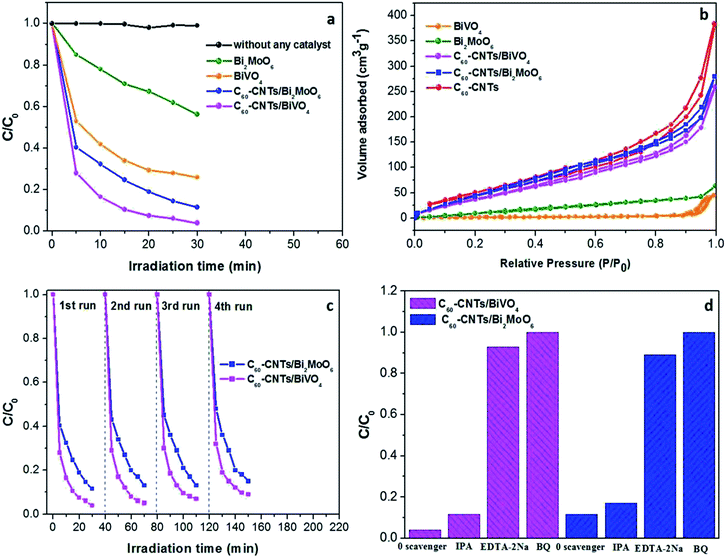 |
| Fig. 5 (a) Photodegradation efficiencies of Rh B as a function of irradiation time for different photocatalysts. (b) N2 adsorption–desorption isotherm curves of the as-prepared samples. (c) Cycling runs for photocatalytic degradation of Rh B over the ternary nanocomposites under visible-light irradiation. (d) Trapping experiment of active species during photocatalytic degradation of Rh B over C60CNTs/bismuth-based oxide nanocomposites under visible-light irradiation. | |
Table 1 BET specific surface area of the as-prepared samples
Photocatalysts |
BET specific surface area (m2 g−1) |
Photocatalysts |
BET specific surface area (m2 g−1) |
BiVO4 |
20.913 |
C60-CNTs/BiVO4 |
146.071 |
Bi2MoO6 |
44.202 |
C60-CNTs/Bi2MoO6 |
179.465 |
C60/CNTs |
188.426 |
|
|
To further probe the underlying mechanism of the ternary nanocomposites, different scavengers for the various active species were added into the reaction system. In particular, IPA, BQ, and EDTA-2Na were used to capture ˙OH, ˙O2−, and h+, respectively. As shown in Fig. 5d, the photocatalytic performance of C60-CNTs/Bi2MoO6 toward Rh B degradation was clearly inhibited by the addition of EDTA-2Na and BQ. Similar results were observed with the trapping of active species during the photocatalytic degradation of Rh B over C60-CNTs/BiVO4. These results indicate that ˙O2− and h+ play critical roles in the photocatalytic degradation of Rh B for the ternary composites.
The possible photocatalytic mechanism of the C60-CNTs/Bi2MoO6 nanocomposite is illustrated in Fig. 6. First, the large specific surface areas of the ternary nanocomposite and its enhanced adsorption ability toward the contaminants contribute to the photocatalytic process. Particularly, the loading of C60-CNTs promotes the visible-light absorption of Bi2MoO6, which enables photoexcitation of more electrons from the VB to the CB.21 Further, the small C60 molecules can serve as an efficient electron reservoir to capture the photogenerated electrons, thus hindering the recombination of electron–hole carriers.22 Meanwhile, CNTs can also help to suppress the charge recombination by capturing the photogenerated electrons owing to their excellent electron conductivity and mobility.23 On the other hand, owing to the formation of heterojunctions between C60-CNTs and Bi2MoO6 and their intimate interfacial interaction, photogenerated electrons can efficiently transfer to C60 and CNTs, thus showing enhanced separation efficiency for the photogenerated holes–electrons pairs. In this way, the excited electrons participate in the reduction of O2 to ˙O2−. Subsequently, the ˙O2− and holes on the valence band of Bi2MoO6 oxidize the Rh B to CO2 and H2O.
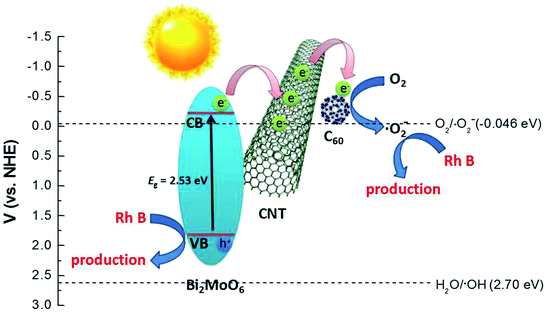 |
| Fig. 6 Schematic diagram of the separation and transfer of photogenerated charges in the C60-CNTs/Bi2MoO6 composite photocatalyst under visible-light irradiation. | |
4. Conclusions
In this study, C60-decorated SWCNTs (C60-CNTs) were prepared by a solution method. Thereafter, novel nanostructured carbon/bismuth-based oxide nanocomposites were successfully synthesized via a facile hydrothermal method with nanostructured carbons. These nanostructured carbon/bismuth-based oxide nanocomposites are beneficial for improving the photocatalytic efficiency. The enhanced performance is due to the extended absorption in the visible-light region resulting from C60-CNTs loading, high specific surface area, and efficient separation of electron–hole pairs by the ternary composite system. This study suggests that the C60-CNTs, as a new carbon nanostructured material, can be employed as an effective co-catalyst for photocatalytic application.
Conflicts of interest
There are no conflicts to declare.
Acknowledgements
This work was supported by the Project of State Forestry Administration of China (201504502), the National Natural Science Foundation of China (21407059) and the Open Subject of the State Key Laboratory of Rare Earth Resource Utilization (RERU2017011).
References
- B. Qiu, M. Xing and J. Zhang, J. Am. Chem. Soc., 2014, 136, 5852–5855 CrossRef CAS PubMed.
- P. P. Gai, R. B. Song, C. Zhu, Y. S. Ji, Y. Chen, J. R. Zhang and J. J. Zhu, Chem. Commun., 2015, 51, 14735–14738 RSC.
- W. B. Li, C. Feng, S. Y. Dai, J. G. Yue, F. X. Hua and H. Hou, Appl. Catal., B, 2015, 168, 465–471 CrossRef.
- J. N. Hui, G. Zhang, C. S. Ni and J. T. S. Irvine, Chem. Commun., 2017, 53, 10038–10041 RSC.
- R. C. Pawar and C. S. Lee, Appl. Catal., B, 2014, 144, 57–65 CrossRef CAS.
- K. A. Tsai and Y. J. Hsu, Appl. Catal., B, 2015, 164, 271–278 CrossRef CAS.
- Z. F. Huang, L. Pan, J. J. Zou, X. W. Zhang and L. Wang, Nanoscale, 2014, 6, 14044–14063 RSC.
- D. F. Hou, X. L. Hu, P. Hu, W. Zhang, M. F. Zhang and Y. H. Huang, Nanoscale, 2013, 5, 9764–9772 RSC.
- T. T. Li, X. L. Hu, C. C. Liu, C. M. Tang, X. K. Wang and S. L. Luo, J. Mol. Catal. A: Chem., 2016, 425, 124–135 CrossRef CAS.
- N. Liang, M. Wang, L. Jin, S. S. Huang, W. L. Chen, M. Xu, Q. Q. He, J. T. Zai, N. H. Fang and X. F. Qian, ACS Appl. Mater. Interfaces, 2014, 6, 11698–11705 CAS.
- J. C. Wang, H. C. Yao, Z. Y. Fan, L. Zhang, J. S. Wang, S. Q. Zang and Z. J. Li, ACS Appl. Mater. Interfaces, 2016, 8, 3765–3775 CAS.
- S. Yuan, Y. Zhao, W. B. Chen, C. Wu, X. Y. Wang, L. N. Zhang and Q. Wang, ACS Appl. Mater. Interfaces, 2017, 9, 21781–21790 CAS.
- L. Zhou, Y. Yang, J. Zhang and P. M. Rao, ACS Appl. Mater. Interfaces, 2017, 9, 11356–11362 CAS.
- T. Saison, N. Chemin, C. Chanéac, O. Durupthy, L. Mariey, F. Maugé, V. Brezová and J. P. Jolivet, J. Phys. Chem. C, 2015, 119, 12967–12977 CAS.
- Y. C. Hao, X. L. Dong, S. R. Zhai, X. Y. Wang, H. C. Ma and X. F. Zhang, RSC Adv., 2016, 6, 35709–35718 RSC.
- S. J. Li, X. F. Shen, J. S. Liu and L. S. Zhang, Environ. Sci.: Nano, 2017, 4, 1155–1167 RSC.
- S. N. Lou, J. Scott, A. Iwase, R. Amal and Y. H. Ng, J. Mater. Chem. A, 2016, 4, 6964–6971 CAS.
- T. Yan, Q. Yan, X. D. Wang, H. Y. Liu, M. M. Li, S. X. Lu, W. G. Xu and M. Sun, Dalton Trans., 2015, 44, 1601–1611 RSC.
- J. S. Cai, J. Y. Huang and Y. K. Lai, J. Mater. Chem. A, 2017, 5, 16412–16421 CAS.
- J. L. Li, X. J. Liu, X. Q. Piao, Z. Sun and L. K. Pan, RSC Adv., 2015, 5, 16592–16597 RSC.
- B. Chai, T. Y. Peng, X. H. Zhang, J. Mao, K. Li and X. G. Zhang, Dalton Trans., 2013, 42, 3402–3409 RSC.
- B. Chai, X. Liao, F. Song and H. Zhou, Dalton Trans., 2014, 43, 982–989 RSC.
- L. F. Yue, S. F. Wang, G. Q. Shan, W. Wu, L. W. Qiang and L. Y. Zhu, Appl. Catal., B, 2015, 176–177, 11–19 CrossRef CAS.
- S. Reddy, R. Du, L. X. Kang, N. N. Mao and J. Zhang, Appl. Catal., B, 2016, 194, 16–21 CrossRef CAS.
- J. G. Yu, T. T. Ma, G. Liu and B. Cheng, Dalton Trans., 2011, 40, 6635–6644 RSC.
- H. P. Li, J. Y. Liu, W. G. Hou, N. Du, R. J. Zhang and X. T. Tao, Appl. Catal., B, 2014, 160–161, 89 CrossRef CAS.
- H. Q. Pan, X. K. Li, Z. J. Zhuang and C. Zhang, J. Mol. Catal. A: Chem., 2011, 345, 90 CrossRef CAS.
- S. Juntrapirom, D. Tantraviwat, S. Suntalelat, O. Thongsook, S. Phanichphant and B. Inceesungvorn, J. Colloid Interface Sci., 2017, 504, 711–720 CrossRef CAS PubMed.
- S. J. Li, S. W. Hu, K. B. Xu, W. Jiang, Y. Liu and Z. Leng, J. Colloid Interface Sci., 2017, 504, 561–569 CrossRef CAS PubMed.
- Y. L. Qi, Y. F. Zheng, H. Y. Yin and X. C. Song, J. Alloys Compd., 2017, 712, 535–542 CrossRef CAS.
- Q. Z. Luo, X. L. Yang, X. X. Zhao, D. S. Wang, R. Yin, X. Y. Li and J. An, Appl. Catal., B, 2017, 204, 304–315 CrossRef CAS.
- Y. R. Zhao, J. Z. Ma, J. L. Liu and Y. Bao, Colloids Surf., A, 2017, 518, 57–63 CrossRef CAS.
- Y. Q. Yang, W. K. Zhang, R. X. Liu, J. M. Cui and C. Deng, Sep. Purif. Technol., 2018, 190, 278–287 CrossRef CAS.
- Y. F. Zhang, M. Park, H. Y. Kim and S. J. Park, J. Alloys Compd., 2016, 686, 106–114 CrossRef CAS.
Footnote |
† Electronic supplementary information (ESI) available. See DOI: 10.1039/c7ra11056a |
|
This journal is © The Royal Society of Chemistry 2017 |