DOI:
10.1039/C7RA10525H
(Paper)
RSC Adv., 2017,
7, 51374-51381
Rieche formylation of carbon nanotubes – one-step and versatile functionalization route†
Received
22nd September 2017
, Accepted 24th October 2017
First published on 6th November 2017
Abstract
We present the direct and one-step Rieche formylation of carbon nanotubes (CNTs) as the most effective (4.00 mmol g−1) functionalization route via formylation. Indeed, classical methods, i.e. three-step protocols based on CNT oxidation–reduction–partial oxidation (COOH ≫ CH2OH ≫ CHO), were revealed as less effective and more troublesome. All CNT-CHO products were comprehensively analysed by cross-verified potentiometric titration, scanning electron microscopy (SEM), thermogravimetry (TGA), Fourier transform infrared spectroscopy (FT-IR) and Raman spectroscopy.
Introduction
Exohedral functionalization of carbon nanotubes (CNTs)—inspired by organic chemistry—has opened up numerous toolboxes toward CNT applications in various fields, from electronics to materials engineering to medicine.1 Typically, the CNT-anchored functional groups or nanoparticles provide better dispersibility in solvents and enhanced compatibility with polymer (or metal or ceramic) matrices. Consequently, this approach makes CNTs processable and conducive to a ‘properties-by-design’ approach.2 Those physicochemical CNT outer-shell modifications include several methods—leading to nanotube disentanglement and individualization—based on the formation of non-covalent and covalent bonds.3 Among the latter, i.e. the ones most influencing the electronic and morphological skeleton of CNTs, several different methods have been reported, and they are predominantly based on oxidation using HNO3,4 nitrating mixtures,5 KMnO4,6 H2O2
7 or O3.8 Furthermore, a number of other CNT reactions with radicals,9–11 cycloadditions,12–15 and electrophilic additions16–18 have also been studied. Nevertheless, in total, the aforementioned oxidation has been, by now, undeniably the most frequently and widely used method to alter/tune the physicochemistry of CNTs. This fact derives from usually high functionalization degrees, as well as the convenience and versatility of the introduced carboxyl (–COOH) and hydroxyl (–OH) groups as the anchoring points, mainly for further covalent modifications.19 This approach, however, induces defects in the nanotube walls, decreases the nanotube aspect ratio (nanotubes can be cut) and consequently aggravates the mechanical and electrical properties of CNTs; oxidized CNTs also become hydrophilic.20 As for non-destructive treatments, Tessonnier et al.21 reported the modification of CNTs with tertiary amino groups without the oxidation step. The procedure involved deprotonation–lithiation/carbometalation reaction followed by an electrophilic substitution by 2-(diethylamino)ethyl bromide. In this two-step approach, concentration of the 3°-amino functional groups was found to be 1 mmol g−1; nonetheless, the method did not require harsh conditions.
In the quest for alternative routes, we have turned to a synthetic precursor of the carboxyl group, i.e., the aldehyde group, which might emerge as even more powerful – indeed, the high and multi-target reactivity of aldehydes (RCHO) locates them among the most important organic compounds. Definitely, the formyl group (–CHO) appears as a powerful surface modifier, as it constitutes a convenient starting point for the synthesis of alcohols,22,23 amines,24,25 acids,26 oximes,27,28 alkenes,29,30 alkynes,31 epoxides,32,33 etc. Therefore, formylation of CNTs is a natural synthetic route to practically countless post-modifications of formylated CNTs that are susceptible to many readily available nucleophiles. However, to our extreme surprise, literally just one synthetic strategy of CNT formylation has been applied to date,34,35 i.e., the pre-formation of lithiated CNTs (CNT anion = ‘carbon nanotubide’) and their subsequent reaction with N-formylpiperidine (which is, formally, transfer of the formyl group).
In order to explore new areas of CNT chemistry—against the background of organic chemistry methods that have been reserved mainly for aromatic low-molecular-weight molecules—we report on studies of CNT formylation. Herein, so as to perform direct (one-step) formylation of CNTs, we have elaborated a protocol based on Rieche formylation, i.e. employing dichloromethyl methyl ether (Cl2CHOCH3) as a formylating agent in the presence of TiCl4 as Lewis acid. The intermediate product in this reaction is an unstable α-alkoxybenzyl chloride which further decomposes to yield exclusively the appropriate aldehyde. Nevertheless, application of the Rieche reaction could be a challenge, as it was initially reserved for activated aromatic compounds bearing electron-donating groups. Hence, it could provide higher functionalization levels, but only for suitably pre-activated CNTs. On the other hand, one might expect that MWCNTs, as metallic tubes, could be more susceptible to the attack of reactive electrophiles.36,37 In order to place the above hypothesis in the appropriate context, for comparative purposes, as the commonly applied alternative approach, CNTs were initially oxidized (the first general step) to CNT-COOH, which was further reduced with lithium aluminium hydride (LAH) to hydroxymethyl-CNTs (CNT-CH2OH). The resulting hydroxymethyl groups were oxidized to the formyl ones using CrO3, K2Cr2O7 or HBr.
Experimental
Materials
MWCNTs (9.6 nm diameter, 1.5 μm length, 90% purity) were purchased from Nanocyl, Belgium. Longer and thicker in-house MWCNTs were synthesized by catalytic chemical vapour deposition (c-CVD) (a detailed procedure is presented in ESI†). SEM images of in-house MWCNTs (see further) showed that most of them had a diameter ranging between 60–70 nm and length up to 200 μm. Single-walled CNTs (SWCNTs) were purchased from TUBALL™ (Luxembourg) and were used additionally as the important model nanotubes. General characteristics of all CNTs used in the work are presented in Table 1. LiAlH4 (95%), HBr(aq) (48%, ACS reagent), DMSO (>99%), TiCl4 (>99%), dichloromethyl methyl ether (98%), CaH2 (pure p.a.), ferrocene (FeCp2) (98%), and NH2OH HCl (99%) from Sigma-Aldrich, Poland; H2SO4 (95%, pure p.a.), HNO3 (65%, pure p.a.), HCl (35–38%, pure p.a.), NaOH (pure p.a.), anhydrous MgSO4 (pure p.a.), NaHCO3 (pure p.a.), Na2CO3 (pure p.a.), and toluene (pure p.a.) from Chempur, Poland; and CrO3 (pure), K2Cr2O7 (pure), and KBr (pure) from Avantor Performance Materials, Poland, were used as received. Prior to use, methylene chloride and toluene were extracted with conc. H2SO4, saturated NaHCO3(aq) solution and water, dried with anhydrous MgSO4, and then distilled from CaH2. Filtrations were performed using PTFE filters (Merck Milipore pore size 0.2 μm).
Table 1 Characterization of CNTs
Properties |
Nanocyl NC7000™ |
In-house MWCNTs |
TUBALL™ SWCNTs |
Average diameter, nm |
9.5 |
60–70 |
1.6 |
Average length, μm |
1.5 |
200 |
>5 |
Aspect ratio |
150 |
3000 |
3000 |
Carbon purity, wt% |
90 |
98 |
85 |
Fe-based catalyst residue, wt% |
<1 |
5.4 |
<15 |
Synthesis of carboxy-MWCNTs (MWCNT-COOH)
MWCNTs (5.00 g) were treated with a mixture of HNO3
:
H2SO4 (1
:
3; v/v) (128 mL). The reaction mixture was refluxed for 10 min, with the vigorous evolution of NO2. Next, after cooling down to room temperature (2 h), the oxidized nanotubes were filtered off. Subsequently, MWCNT-COOH was rinsed thoroughly with water until pH = 7. MWCNT-COOH was dried at 85 °C for 12 h. The reaction scheme is presented in Fig. 1.
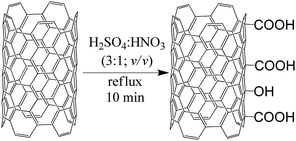 |
| Fig. 1 Procedure for synthesis of MWCNT-COOH. | |
Reduction of MWCNT-COOH to hydroxymethyl-MWCNTs (MWCNT-CH2OH) [modified procedure from ref. 38]
To a magnetically stirred, 250 mL three-necked, round-bottomed flask containing MWCNT-COOH (1.00 g) and LiAlH4 (0.50 g), and equipped with: (1) a drying pipe (cont. CaCl2), (2) a dropping funnel, and (3) an Ar balloon, anhydrous toluene (150 mL) was added over 30 min. The reaction mixture was stirred for 24 h. Then, the reaction mixture was quenched, and MWCNT-CH2OH was filtered off and rinsed with 2 M HClaq (50 mL). MWCNT-CH2OH was washed thoroughly with water until neutral pH of the filtrate. The resulting material was dried at 85 °C overnight. The reaction scheme is presented in Fig. 2.
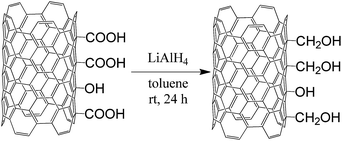 |
| Fig. 2 Procedure for reduction of MWCNT-COOH to hydroxymethyl-MWCNTs (MWCNT-CH2OH). | |
Partial oxidation of MWCNT-CH2OH to formyl-MWCNTs (MWCNT-CHO)
Method 1 [modified procedure from ref. 39 for aromatic compounds]. To a magnetically stirred dispersion of MWCNT-CH2OH (0.20 g) in DMSO (25 mL) in a round-bottomed flask equipped with a reflux condenser, a solution of CrO3 (0.50 g) in DMSO (5 mL) was added dropwise over 5 min. After stirring for 4 h at room temperature, cold distilled water (100 mL) was added to the reaction mixture, and the product was filtered off. MWCNT-CHO was washed with water (100 mL) and acetone (50 mL) until a colourless filtrate was observed. The resulting material was dried at 85 °C overnight. The reaction scheme is presented in Fig. 3.
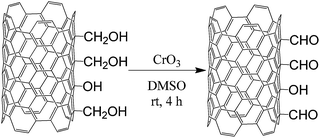 |
| Fig. 3 Procedure for partial oxidation of MWCNT-CH2OH to formyl-MWCNTs (MWCNT-CHO) using chromium trioxide (CrO3) in DMSO. | |
Method 2 [modified procedure from ref. 40 for aromatic compounds]. MWCNT-CH2OH (0.20 g), K2Cr2O7 (1.00 g) and DMSO (25 mL) were placed in a round-bottomed flask equipped with a reflux condenser. The mixture was heated at 100 °C for 4 h. After cooling down to room temperature, the product was filtered off. MWCNT-CHO was washed with water (100 mL) and acetone (50 mL) until a colourless filtrate was observed. The resulting material was dried at 85 °C overnight. The reaction scheme is presented in Fig. 4.
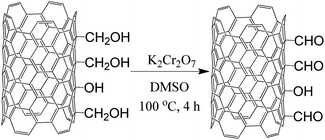 |
| Fig. 4 Procedure for partial oxidation of MWCNT-CH2OH to formyl-MWCNTs (MWCNT-CHO) with potassium dichromate (K2Cr2O7) in DMSO. | |
Method 3 [modified procedure from ref. 41 for aromatic compounds]. A mixture of MWCNT-CH2OH (0.20 g), 48% HBraq (0.5 mL) and DMSO (20 mL) was magnetically stirred in an oil bath at 100 °C for 4 h. After cooling down to room temperature, the product was filtered off. MWCNT-CHO was washed with water (100 mL) and acetone (50 mL) until a colourless filtrate was observed. The resulting material was dried at 85 °C overnight. The reaction scheme is presented in Fig. 5.
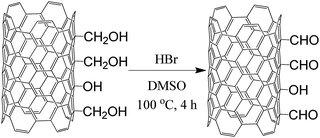 |
| Fig. 5 Procedure for oxidation of MWCNT-CH2OH to formyl-MWCNTs (MWCNT-CHO) with hydrobromic acid (HBraq) in DMSO. | |
Rieche formylation of MWCNTs
To a round-bottomed flask cooled to 0 °C containing MWCNTs (1.00 g) and dichloromethyl methyl ether (1.5 mL) in anhydrous CH2Cl2 (50 mL), TiCl4 (1 mL) was added dropwise under N2, and the mixture, under the above conditions, was magnetically stirred for 1 h.42 The stirring was continued at room temperature for 20 h. The product was filtered off. MWCNT-CHO precursor was washed with water (100 mL) and acetone (50 mL) until a colourless filtrate was observed. The resulting material was dried at 85 °C overnight. The reaction scheme is presented in Fig. 6.
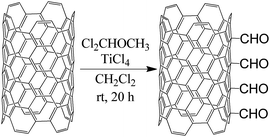 |
| Fig. 6 Rieche formylation of CNTs. | |
Characterization
Scanning electron microscopy (SEM), titration, thermogravimetry (TGA), Fourier transform infrared (FTIR) spectroscopy and Raman spectroscopy were used to characterize the changes in morphology and surface physicochemistry of functionalized CNTs. SEM images were taken on Hitachi TM3000. Samples were analyzed at 5 kV with a working distance of 4.5 mm. TGA curves were recorded under nitrogen using a TGA/DSC1 Mettler-Toledo at a heating rate of 10 °C min−1. FTIR spectra were acquired using a Specord M80 (KBr pellet – 0.5 mg CNTs/1 g KBr, 500–4000 cm−1 range). Raman spectra were obtained using a InVia Renishaw at 633 nm (He/Ne laser).
The content of CNT surface oxygen groups was determined by Boehm titration,43 and the procedure was as follows: functionalized MWCNTs (60 mg) were dispersed for 48 h under continuous stirring in the specified aqueous reaction base solutions: (1) freshly prepared 0.01 M NaOH, 60 mL; (2) 0.01 M Na2CO3, 30 mL; (3) 0.01 M NaHCO3, 60 mL. The mixture was filtered, then 10 mL (or 5 mL in the case of Na2CO3) of the filtrates was titrated with 0.01 M HClaq (20 mL). The pH of the final solutions was measured using a pH meter (EcoTestr 2). Each titration was performed in triplicate. As blank samples, 10 mL of each reaction base (5 mL in the case of Na2CO3) was used. For quantification of surface formyl groups in CNT-CHO, reaction of the aldehyde groups with hydroxylamine was used (Fig. 7): a given MWCNT sample (20 mg) and hydroxylamine hydrochloride (35 mg) solution in water (50 mL) were ultrasonicated for 5 min. Then, the analyzed mixture was heated to 60 °C and kept at this temperature for 20 min. The evolved HCl was titrated with 0.01 M NaOHaq. Each titration was performed in triplicate. A schematic of the titration setup is presented in Fig. 8.
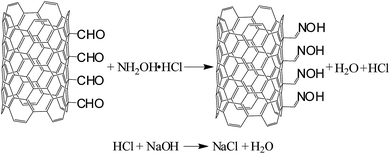 |
| Fig. 7 Reactions during quantification of the formyl groups in CNT-CHO determined by potentiometric titration—formation of CNT-oximes with a simultaneous release of hydrogen chloride. | |
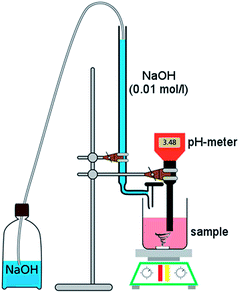 |
| Fig. 8 Setup of the titration experiment. | |
Results and discussion
Morphology of CNT-CHO versus unmodified CNTs
The morphology of CNTs and their functionalized counterparts was analyzed by SEM; Fig. 9 shows SEM micrographs for pristine Nanocyl™, in-house and TUBALL™ Fig. 9(a, b, c) and formylated CNTs. Nanocyl MWCNTs were found as highly twisted and entangled nanoparticles forming nearly spherical (cauliflower-like) agglomerates. In contrast, in-house MWCNTs (of the highest diameter) and TUBALL™ SWCNTs, due to the similar and high aspect ratio (3000) among the studied CNTs, were visible as mostly vertically aligned tubes forming separate icelands (‘carpets’) and sets of fibrils, respectively. Further, Fig. 9(d–g) show formylated Nanocyl™, Fig. 9(h–k) formylated in-house MWCNTs and Fig. 9(l) formylated TUBALL™. As can be seen, none of the treatments have led to alteration of the nanotube morphology, leaving the nanotube intact on the micro-scale. Critically, in light of the results on effective functionalization presented later—as well as from the further application point of view—CNTs after Rieche formylation procedure also remained unchanged (long and straight tubes are present) and also, due to a straightforward one-step protocol, practical lack of impurities could be observed. This comparison has important consequences for three-step formylation procedures, which potentially generate more extensive additional contamination.
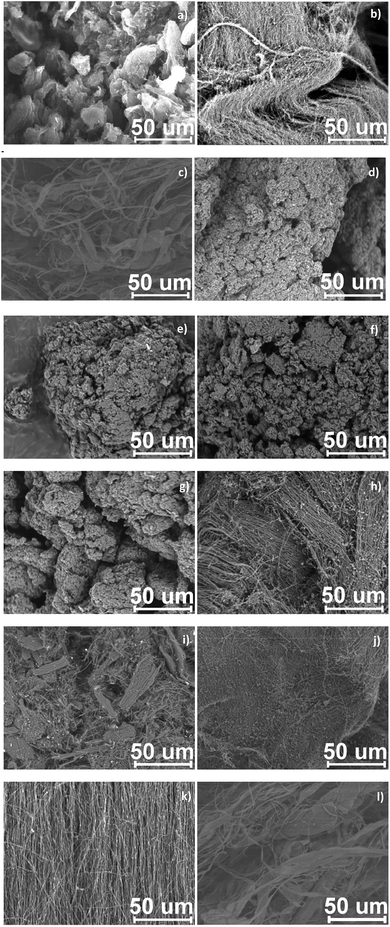 |
| Fig. 9 SEM images of CNTs: (a) Nanocyl™ MWCNTs, (b) in-house MWCNTs, (c) TUBALL™ SWCNTs, (d) f-Nanocyl™ by CrO3/DMSO, (e) f-Nanocyl™ by K2Cr2O7/DMSO, (f) f-Nanocyl™ by HBr/DMSO, (g) f-Nanocyl™ by Cl2CHOCH3, TiCl4, (h) f-in-house by CrO3/DMSO, (i) f-in-house by K2Cr2O7/DMSO, (j) f-in-house by HBr/DMSO, (k) f-in-house by Cl2CHOCH3, TiCl4, (l) f-TUBALL™ by Cl2CHOCH3, TiCl4. | |
FTIR spectroscopy
Fig. 10 shows the FTIR spectra of SWCNTs before (black line) and after (red line) Rieche formylation. For pristine SWCNTs, the bands at 3450 (νO–H) and 1262 cm−1 (νC–O) could be attributed to the presence of surface hydroxyl groups, which are believed to result from ambient atmospheric moisture bound to the outermost SWCNT shells.44 Nota bene, the presence of surface hydroxyl groups activates the graphene wall to the electrophilic attack.
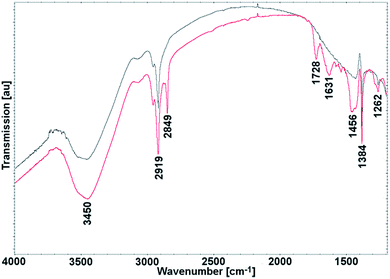 |
| Fig. 10 FTIR spectra of SWCNTs, (black) before and (red) after direct formylation. | |
For the formylated SWCNTs, two new bands appeared at 1728 and 2849 cm−1. The emergence of an absorption peak at 1728 cm−1 in the FTIR spectrum of functionalized SWCNTs is a clear indication of carbonyl (C
O) groups. The peak can be assigned to the C
O stretching vibration (νC
O). Importantly, a peak at 2849 cm−1 corresponds to asymmetrical stretching vibrations of O
C–H bonds in aldehydes (νC–H). Bands at around 3000, 1631, 1456, and 1384 cm−1 are connected with the aromatic structure of CNTs. Weak signals at ca. 3000 cm−1 correspond to stretching vibrations of CAr–H (νC–H). Skeletal vibrations, involving carbon–carbon stretching within the ring, absorbed energy at 1631, 1456 and 1384 cm−1 (νC
C). Two very weak bands between 1456 and 1384 cm−1 can be attributed to overtone bands.
Quantification of carboxyl groups and formyl groups in CNT-CHO via titration
The amount of carboxyl groups was calculated as follows:
where CHCl – concentration of the titrant [mol L−1], V0HCl – volume of the titrant in blank titration [mL], VHCl – volume of the titrant [mL], mCNTs – amount of CNTs used for the titration analysis [g].
The concentrations of the determined formyl groups for CNT samples after formylation are summarized in Table 2.
Table 2 The carboxyl group content in MWCNT samples after –COOH to –CHO conversion as determined by titrationb
Functionalising agent in DMSO |
Functionalization degree [mmol COOH g−1 CNTs] |
Nanocyl™ NC7000 |
In-house |
Beforea |
Aftera |
Beforea |
Aftera |
Before – titration for MWCNT-COOH; after – titration for MWCNT-CHO. In the case of Rieche formylation, no carboxyl groups were detected. |
K2Cr2O7 |
3.0 ± 0.10 |
1.0 ± 0.12 |
1.5 ± 0.08 |
0.50 ± 0.02 |
CrO3 |
1.0 ± 0.09 |
0.30 ± 0.01 |
HBr |
0.5 ± 0.04 |
0.80 ± 0.01 |
Degree of functionalization was calculated using the equation:
where
VNaOH – volume of the titrant [mL],
CNaOH – molar concentration of the titrant [mol L
−1],
V0NaOH – volume of the titrant in blank titration [mL],
mCNTs – amount of CNTs used for the titration analysis [g].
The determined concentrations of the formyl groups in CNT samples after formylation are summarized in Table 3.
Table 3 The formyl group content in Rieche-formylated CNTs in comparison with alternatively synthesized CNT-CHO via the ‘COOH ≫ CH2OH ≫ CHO’ route as determined by titration
Functionalising agent in DMSO |
Functionalization degree [mmol g−1 CNTs] |
Nanocyl™ NC7000 |
In-house |
TUBALL™ |
K2Cr2O7 |
1.90 ± 0.06 |
1.00 ± 0.03 |
— |
CrO3 |
1.96 ± 0.05 |
1.14 ± 0.03 |
— |
HBr |
2.38 ± 0.07 |
0.75 ± 0.02 |
— |
Cl2CHOCH3, TiCl4 |
3.68 ± 0.11 |
1.25 ± 0.04 |
4.00 ± 0.12 |
The influence of dichloromethyl methyl ether concentration on the functionalization degree was further verified by titration of the Rieche-formylated Nanocyl MWCNTs, and the results are presented in Fig. 11. It can be seen that at lower concentration of the electrophile, with its increasing concentration, degree of functionalization has also increased, with a linear dependence. But when concentration of the ether was higher than 16 mmol g−1 CNTs, only a slight further increase in the functionalization level could be observed, indicating saturation of the MWCNT active sites. Those reactive hot-spots could be ascribed to the graphene wall defects. This relationship proves the quantitative tunability of this type of functionalization.
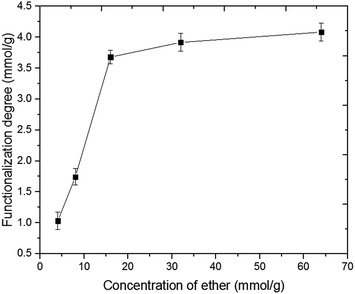 |
| Fig. 11 Effect of the ether concentration (mmol g−1 MWCNTs) on the functionalization degree with formyl groups; the line serves as the eye-guide only; the titrations for each measuring point were performed in triplicate. | |
Thermogravimetric analyses
In order to further confirm functionalization degrees in CNT-CHO, TG analyses were performed in argon atmosphere (Fig. 12). It can be assumed that the weight loss above 300 °C could be ascribed to the actual quantity of covalently bonded formyl groups (temperature of decarbonylation of aromatic compounds and above the range for volatilization of water and organic solvents). This characteristic was exploited in other studies.45,46 The weight loss in the range 320–360 °C corresponds to thermal decarbonylation in the CNT-CHO, in which formyl groups were covalently attached to the CNT walls.35 Therefore, functionalization degrees were calculated by a projection of the peak onset and offset points in derivative thermogravimetry (DTG) curves onto the corresponding TGA curves. The as-estimated functionalization degrees are presented in Table 4.
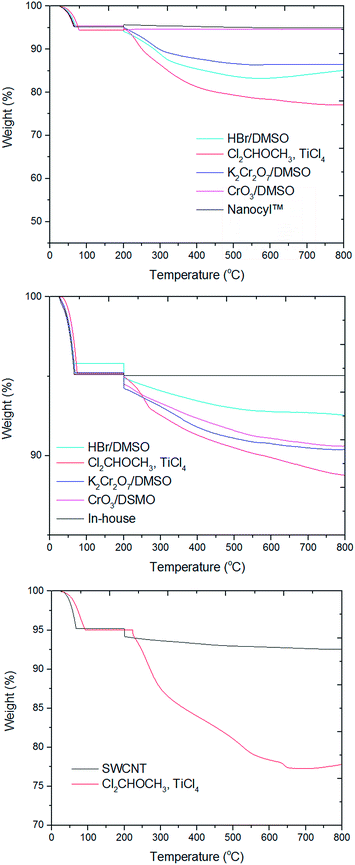 |
| Fig. 12 Representative TGA plots for pristine (Nanocyl™ NC7000, in-house and TUBALL™) versus formylated CNTs using different functionalizing agents. | |
Table 4 Functionalization degrees (mmol g−1 CNTs) estimated from TGA for CNT-CHO
Functionalising agent |
Functionalization degree [mmol g−1 CNTs] |
Nanocyl™ NC7000 |
In-house |
TUBALL™ |
K2Cr2O7, DMSO |
1.76 |
0.94 |
— |
CrO3, DMSO |
1.81 |
0.94 |
— |
HBr, DMSO |
2.37 |
0.67 |
— |
Cl2CHOCH3, TiCl4 |
3.66 |
1.12 |
3.90 |
The highest functionalization degrees were observed for the CNT samples after Rieche formylation, the lowest for Nanocyl™ NC7000 after K2Cr2O7 and in-house after HBr/DMSO. SWCNTs and shorter/thinner Nanocyl™ NC7000 were more reactive in formylation reactions than longer and thicker in-house MWCNTs. The obtained values fully correspond with the values determined by titration. Additionally, the conversion of carboxyl to formyl groups was incomplete and ranged from 47 to 67%. In comparison, in formylation studies presented by Bayazit et al.,34 N-formylpiperidine has been used for the transfer of formyl groups to lithiated SWCNTs. The authors have observed for SWCNT-CHO in the TG curves weight loss of 25 wt% (with 5 wt% for purified SWCNT). However, at the same time, they have claimed that each introduction of the formyl group caused a simultaneous introduction of an ‘ortho-located’ n-butyl group. Hence, the actual degree of functionalization as counted solely per formyl group was in fact lower, i.e. approximately 2–3 mmol g−1.
Raman characterization of SWCNTs vs. SWCNT-CHO
The Raman spectrum of SWCNTs basically contains four characteristic features. Radial breathing mode (RBM) can be generally found between 100–400 cm−1.47 The feature in the region between 1500–1600 cm−1 is related to tangential G-mode and is an intrinsic feature in all sp2-carbon materials. Lineshape of the G-band differs in accordance with whether the nanotubes are semiconducting or metallic.48 The Raman feature around 1350 cm−1 corresponds to disorder-induced D-mode. The D-band involves scattering from defects that break the basic symmetry of the graphene sheet, and it is observed in sp2-carbons containing porous fraction, impurities, functionalization or other defects.49 The Raman spectrum also contains a band at 2500–2700 cm−1 (G′-band), and it depends on the diameter of CNT.50
Here, the Raman spectrum (Fig. 13) of formylated SWCNTs (SWCNT-CHO) (B) were found as significantly different from that of pristine SWCNTs (A). With respect to the tangential mode at 1593 cm−1 (G-band), the intensity of the disorder peak at 1330 cm−1 (D-band) has changed significantly in SWCNT-CHO, and the ID/IG ratio increased from ca. 0.02 to 0.11, indicating conversion of sp2-to sp3-carbons in formyl-SWCNT. The disorder mode involves the resonance-enhanced scattering of an electron via phonon emission, which is induced by defects breaking the basic symmetry of the graphene plane. Thus, ID/IG ratio reflects the relative number of sp3-carbon atoms on SWNTs, and it can be used further to indicate the functionalization. The RBM band intensity reduction could be also used as evidence of successful covalent functionalization.51 Furthermore, the frequency of the tangential vibrational modes of the carbon atoms in the SWCNT-CHO shifted to higher frequencies compared to pristine SWCNTs (1589 cm−1). Those shifts provide evidence for charge transfer between the dopant and the SWCNT. Due to the electron-acceptor character of the formyl group, electrons from the π-state carbon transfer to the doping functional group and create hole carriers in the SWCNTs. A similar situation was observed for chemical CNT doping with iodine and bromine.52,53
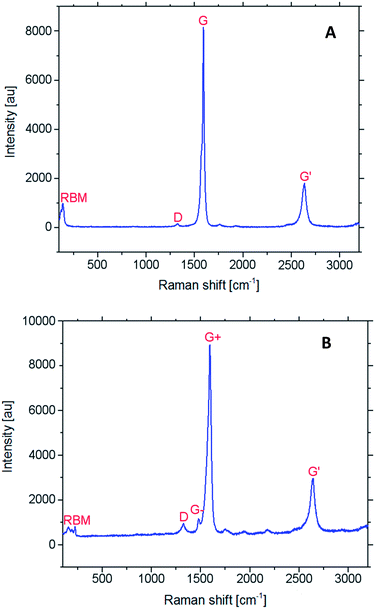 |
| Fig. 13 Raman spectra of SWCNTs: (A) before and (B) after formylation. | |
Conclusions
Rieche reaction was found as the most effective route of CNT formylation. The main advantages of this protocol include: a broad scope of non-disturbing functional groups possibly present in the pre-functionalized CNTs, lack of oxidative disruption of the CNT skeleton, lack of necessity of operating with carcinogenic chromium(VI) compounds and, last but not least, the one-step, one-pot synthetic procedure based on a wet workup. Moreover, the relative reactivity of in-house and Nanocyl MWCNTs resembles our findings from 1,3-dipolar cycloaddition of nitrile N-oxides to nanotubes,54 confirming that such thinner MWCNTs are ca. thrice more reactive, as shown here, against electrophiles.
Conflicts of interest
There are no conflicts to declare.
Acknowledgements
The authors are greatly indebted to Silesian University of Technology (BKM-530/RCH2/2016), National Science Centre and The National Centre for Research and Development (TANGO, TANGO1/266702/NCBR/2015), both Poland. Golden Open Access mode for this work was generously supported from Silesian University of Technology Rector Pro-Quality Grant No. 04/020/RGJ17/0038. Moreover authors acknowledge Dr Eng. Dawid Janas for Raman spectra.
References
- Y.-P. Sun, K. Fu and W. Huang, Acc. Chem. Res., 2002, 35, 1096–1104 CrossRef CAS PubMed.
- S. Mallakpour and S. Soltanian, RSC Adv., 2016, 6, 109916–109935 RSC.
- L. Meng, C. Fu and Q. Lu, Prog. Nat. Sci., 2009, 19, 801–810 CrossRef CAS.
- B. Smith, et al., Langmuir, 2009, 25, 9767–9776 CrossRef CAS PubMed.
- N. P. Blanchard, R. A. Hatton and S. R. P. Silva, Chem. Phys. Lett., 2007, 434, 92–95 CrossRef CAS.
- H. Hiura, T. W. Ebbesen and K. Tanigaki, Adv. Mater., 1995, 7, 275–276 CrossRef CAS.
- Y. Peng and H. Liu, Ind. Eng. Chem. Res., 2006, 45, 6483–6488 CrossRef CAS.
- J. M. Simmons, et al., J. Phys. Chem. B, 2006, 110, 113–118 CrossRef PubMed.
- J. L. Bahr, et al., J. Am. Chem. Soc., 2001, 123, 6536–6542 CrossRef CAS PubMed.
- J. L. Bahr and J. M. Tour, Chem. Mater., 2001, 13, 3823–3824 CrossRef CAS.
- C. A. Dyke and J. M. Tour, J. Am. Chem. Soc., 2003, 125, 1156–1157 CrossRef CAS PubMed.
- Y. Chen, et al., J. Mater. Res., 1198, 13, 2423–2431 CrossRef.
- M. Holzinger, et al., Angew. Chem., Int. Ed., 2001, 40, 4002–4005 CrossRef CAS PubMed.
- K. A. Worsley, K. R. Moonoosawmy and P. Kruse, Nano Lett., 2004, 4, 1541–1546 CrossRef CAS.
- M. J. Moghaddam, et al., Nano Lett., 2004, 4, 89–93 CrossRef CAS.
- G. Viswanathan, et al., J. Am. Chem. Soc., 2003, 125, 9258–9259 CrossRef CAS PubMed.
- S. Pekker, J. P. Salvetat, E. Jakab, J. M. Bonard and L. Forro, J. Phys. Chem. B, 2001, 105, 7938–7943 CrossRef CAS.
- N. Tagmatarchis, V. Georgakilas, M. Prato and H. Shinohara, Chem. Commun., 2002, 2010–2011 RSC.
- N. Karousis and N. Tagmatarchis, Chem. Rev., 2010, 110, 5366–5397 CrossRef CAS PubMed.
- P. C. Ma, J.-K. Kim and B. Z. Tang, Carbon, 2006, 44, 3232–3238 CrossRef CAS.
- J.-P. Tessonnier, A. Villa, O. Majouler, S. S. Su and R. Schlogl, Angew. Chem., 2009, 48, 6543–6546 CrossRef CAS PubMed.
- W. F. Tuley and R. Adams, J. Am. Chem. Soc., 1925, 47, 3061–3068 CrossRef CAS.
- M. R. Johnson and B. Rickborn, J. Org. Chem., 1970, 35, 1041–1045 CrossRef CAS.
- J. K. Whitesell and M. A. Whitesell, Synthesis, 1983, 1983, 517–536 CrossRef.
- A. F. Abdel-Magid, K. G. Carson, B. D. Harris, C. A. Maryanoff and R. D. Shah, J. Org. Chem., 1996, 61, 3849–3862 CrossRef CAS PubMed.
- E. J. Corey, N. W. Gilman and B. E. Ganem, J. Am. Chem. Soc., 1968, 90, 5616–5617 CrossRef CAS.
- L. Saikia, J. M. Baruah and A. J. Thakuri, Org. Med. Chem. Lett., 2011, 1, 1–6 CrossRef PubMed.
- E. Abele, R. Abele and E. Lukevics, Chem. Heterocycl. Compd., 2004, 40, 1–15 CrossRef CAS.
- B. E. Maryanoff and A. B. Reitz, Chem. Rev., 1989, 89, 863–927 CrossRef CAS.
- J. Dambacher, et al., Tetrahedron Lett., 2005, 46, 4473–4477 CrossRef CAS.
- J. C. Gilbert and U. Weerasooriya, J. Org. Chem., 1982, 47, 1837–1845 CrossRef CAS.
- M. Davoust, J.-F. Briere, P.-A. Jaffres and P. Metzner, J. Org. Chem., 2005, 70, 4166–4169 CrossRef CAS PubMed.
- A. Piccinini, S. A. Kavanagh, P. B. Connon and S. J. Connon, Org. Lett., 2010, 12, 608–611 CrossRef CAS PubMed.
- M. K. Bayazit, A. Suri and K. S. Coleman, Carbon, 2010, 48, 3412–3419 CrossRef CAS.
- A. Suri and K. S. Coleman, J. Nanosci. Nanotechnol., 2012, 12, 2929–2933 CrossRef CAS PubMed.
- L. Moradi, N. Izadi and F. Rostami, Int. J. Biomed. Nanosci. Nanotechnol., 2015, 11, 93–99 Search PubMed.
- L. Moradi and I. Etesami, Fullerenes, Nanotubes, Carbon Nanostruct., 2016, 24, 213–218 CrossRef CAS.
- P. C. Ma, J.-K. Kim and B. Z. Tang, Carbon, 2006, 44, 3232–3238 CrossRef CAS.
- W.-X. Lou, Synth. Commun., 1992, 22, 767–772 CrossRef CAS.
- E. Santaniello and P. Ferraboschi, Communications, 1980, 646–647 CAS.
- C. Li, et al., Synlett, 2002, 12, 2041–2042 CrossRef.
- V. Rajeshkumar, Y. T. Lee and M. C. Stuparu, Eur. J. Org. Chem., 2016, 2016, 36–40 CrossRef CAS.
- Y. S. Kim, S. J. Yang, H. J. Lim, T. Kim and C. R. Park, Carbon, 2012, 50, 3315–3323 CrossRef CAS.
- A. Misra, P. K. Tyagi, M. K. Singh and D. S. Misra, Diamond Relat. Mater., 2006, 15, 385–388 CrossRef CAS.
- V. T. Le, et al., Adv. Nat. Sci.: Nanosci. Nanotechnol., 2013, 4, 035017 CrossRef.
- S. Boncel, et al., ACS Biomater. Sci. Eng., 2016, 2, 1273–1285 CrossRef CAS.
- F. Hennrich, et al., J. Phys. Chem. B, 2005, 109, 10567–10573 CrossRef CAS PubMed.
- M. S. Dresselhaus, G. Dresselhaus, A. Jorio, A. G. Souza Filho and R. Saito, Carbon, 2002, 40, 2043–2061 CrossRef CAS.
- S. Costa, E. Borowiak-Palen, M. Kruszynska, A. Bachmatiuk and R. J. Kalenczuk, Mater. Sci.-Pol., 2008, 26, 433–441 CAS.
- L. Bokobza and J. Zhang, eXPRESS Polym. Lett., 2012, 6, 601–608 CrossRef CAS.
- K. Zhang, Q. Zhang, C. Liu, N. Marzari and F. Stellacci, Adv. Funct. Mater., 2012, 22, 5216–5223 CrossRef CAS.
- A. M. Rao, P. C. Eklund, S. Bandow, A. Thess and E. E. Smalley, Nature, 1997, 388, 257–259 CrossRef CAS.
- C. N. R. Rao and R. Voggu, Mater. Today, 2010, 13, 34–40 CrossRef CAS.
- A. P. Herman and S. Boncel, RSC Adv., 2016, 6, 64129–64132 RSC.
Footnote |
† Electronic supplementary information (ESI) available. See DOI: 10.1039/c7ra10525h |
|
This journal is © The Royal Society of Chemistry 2017 |