DOI:
10.1039/C7RA10248H
(Paper)
RSC Adv., 2017,
7, 56655-56661
Fish oil affects the metabolic process of trimethylamine N-oxide precursor through trimethylamine production and flavin-containing monooxygenase activity in male C57BL/6 mice†
Received
15th September 2017
, Accepted 20th November 2017
First published on 18th December 2017
Abstract
High trimethylamine N-oxide (TMAO) circulation levels in vivo are closely related to atherosclerosis, chronic heart failure and chronic kidney disease. Fish oil as a commercial functional food has various positive effects on human health. The aim of the present study was to investigate the effects of long-term supplementation with fish oil on the metabolic process of TMAO after administrating a high dose of TMAO precursors. Male C57BL/6 mice were fed a diet containing 1.5% fish oil for 8 weeks. Plasma choline, trimethylamine (TMA), and TMAO pharmacokinetics were measured after treatment with choline chloride through oral gavage or trimethylammonium chloride by intraperitoneal injection. The mRNA expressions and activities of hepatic flavin-containing monooxygenases (FMOs) were also determined. Results showed that fish oil increased choline circulation levels in plasma, while suppressing the production of TMA and raising the concentration–time curve (AUC) of TMAO according to pharmacokinetic parameters. Further analysis indicated that up-regulated FMO gene expression levels and activities accelerated the metabolic process of TMAO when administrating plenty of TMAO precursors. The above results demonstrated that long-term supplementation with DHA-enriched fish oil could regulate the metabolic process of TMAO in vivo, which provides references for the development of nutritional supplements.
Introduction
Trimethylamine N-oxide (TMAO) is an oxidation product of trimethylamine (TMA) with the formula (CH3)3NO. In recent years, the TMAO level has been proved to be closely related to atherosclerosis, chronic heart failure and chronic kidney disease, and has attracted many researchers' attention.1–3 TMAO is produced in the metabolic process of dietary precursors mainly through the action of gut bacteria and the catalysis of hepatic FMOs.4 Dietary precursors such as choline are converted to TMA by anaerobic microorganisms in the colon.5 Then, TMA is rapidly further oxidized by hepatic FMOs to form TMAO after intestinal absorption.6
A number of experimental studies indicated that the levels of TMAO and its metabolic precursors could be affected by food components. Resveratrol as a dietary intervention has the ability to inhibit TMAO synthesis via remodeling microbiota, and consequently decreases the risk of developing cardiovascular diseases.7 Allicin was also capable of preventing atherosclerosis through reducing carnitine transformation to TMA by gut microbiota.8 Meldonium and D plus B vitamins as anti-atherosclerotic drug and dietary components, respectively, just regulated plasma TMAO circulation levels without dynamic changes of TMAO precursor.9,10 Additionally, our laboratory research has proved that the metabolism of TMAO might be affected by different phosphatidylcholine sources, especially phospholipid (PC) from squid rich in docosahexaenoic acid (DHA) could significantly counter-regulate the TMAO generation.11 It is known that fish oil with high content of n-3 polyunsaturated fatty acids (PUFAs) has beneficial effects on health, such as reducing the risk of cardiovascular disease, ameliorating TMAO induced glucose intolerance.12,13 Nevertheless, to best of our knowledge, little information is available about the effect of fish oil on metabolic process of TMAO after administrating dietary precursors.
Hence, male C57BL/6 mice were fed with diet containing 1.5% fish oil for 8 weeks to investigate metabolic process of TMAO. Plasma choline, TMA, and TMAO levels were measured following the oral gavage of choline chloride or injection of TMA hydrochloride. The FMOs gene expressions and enzyme activities involved were also detected.
Experimental section
Chemicals
The choline chloride, TMA hydrochloride, and TMAO dihydrate were purchased from Sigma (St. Louis, MO, USA). The methanol was purchased from Fisher Scientific (Pittsburgh, PA, USA). Fish oil (triglyceride phenotype, DHA > 70%) was purchased from Sinomega Biotech Engineering Co. Ltd (Zhoushan, Zhejiang, China).
Animals and diets
Male C57BL/6 mice aged 6 weeks were provided by Vital River Laboratories (Beijing, China). All these mice were maintained under pathogen-free conditions at constant humidity of 65 ± 15% and a temperature of 23 ± 2 °C with a 12 h light/dark cycle. After one week adaptation period, all mice were randomly divided into two treatment series containing two groups (n = 6 in each group) in each series, and two groups in the same series were fed with chow diet and fish oil diet, respectively. The fatty acid composition of fish oil was shown in Table S1.† Experimental diets were modified based on AIN-93G, the ingredients and fatty acid compositions of diets were shown in Tables S2 and S3.† After 8 weeks of feeding, choline chloride (340 μg g−1 body weight) was orally gavage to each group in one series followed with a 12 h fasting, and the mice in the other series were not suffered painful experiments. A 10 μL volume of caudal vein blood was collected at 0, 2, 3.5, 4, 4.5, 5, 5.5, 6, 12 h following gavage. One week later, each group was treated with TMA hydrochloride (32.3 μg g−1 body weight) by intraperitoneal injection (i.p). A 10 μL volume of caudal vein blood was collected at 0, 0.5, 1, 1.5, 2, 3 h after the injection. All mice in two series were sacrificed at the last time point. Liver was quickly excised and weighted. Plasma was extracted from the blood sample by centrifuging at 7500× rpm for 15 min. All the samples were frozen in liquid nitrogen and stored at −80 °C until analysis. All animal procedures were performed in accordance with the Guideline for Care and Use of Laboratory Animals of Ocean University of China approved by the Animal Ethics Committee of experimental animal care at Ocean University of China (Qingdao, China).
The measurement of choline, TMA and TMAO in plasma
Liquid chromatography with tandem mass spectrometry (LC-MS/MS) was used to quantity choline, TMA and TMAO according to the previous methods.1 Plasma proteins were precipitated with four volumes of ice-cold methanol, and supernatants were analyzed with a phenyl column (4.6 × 250 mm, 5 mm SB-Phenyl; Agilent Technologies) at a flow rate of 0.8 mL min−1 performed on an Agilent G6410 Triple Quad (QQQ) tandem mass spectrometer (Agilent Technologies) in positive ESI ion mode. The mobile phase consisted of 10 mM ammoniumformate (A) and the mixture of 5 mM ammoniumformate, 25% methanol with 0.1% formic acid (B). Gradient elution was as follows: 0–0.5 min (A); 0.5–3.5 min (B); 3.5–11.5 min (B); 11.5–14.5 min (A). Compounds were monitored in multiple reaction monitoring (MRM) mode with the following transitions: choline: m/z 104 → 60.2; TMA: m/z 60.2 → 44.1; TMAO: m/z 76 → 58.2. Different concentrations of mix standards were used to prepare the calibration curves.
RNA extraction and quantitative RT-PCR
The gene expression levels of mRNA were measured by real time-PCR (RT-PCR). Total RNA was extracted from liver using a Trizol Reagent (Invitrogen, Japan). One μg of total RNA from each sample were reverse-transcribed to cDNA using random primers and Moloney murine leukemia virus (MMLV) reverse transcriptase (Madison, WI). Selected genes were amplified using SYBR Green I Master Mix (Roche, Germany) in an iQ5 real-time detection system (Bio-Rad, USA) with 0.3 mM of both forward and reverse primers. Relative gene expression levels were quantified by the standard curve method. Target gene mRNA concentration was normalized to the mRNA concentration of the housekeeping gene β-actin. The prime sequences for analyzed genes were shown in Table S4.†
The determination of FMO activity and hepatic TMAO level
The liver tissue was homogenized in cold methanol and the supernatant was collected. After filtering through a 3k cutoff spin filter, the hepatic TMAO level was detected by HPLC-MS/MS. The enzymatic activity of FMOs was measured according to the modified method.14 The final reaction system contained 1 mg liver protein homogenate, 300 μM TMA and 100 μM NADPH in 10 mM HEPES (4-(2-hydroxyethyl)-1-piperazineethanesulfonic acid). The reaction mixture was incubated at 37 °C, pH 7.4 for 10 min and stopped by 0.2 N cold formic acid followed by filtering through a 3k cutoff spin filter. The baseline and final concentration of TMAO were detected by HPLC-MS/MS as described above.
Statistical analysis
All results were expressed as mean ± SD (standard deviation). Statistical analyses were performed with SPSS 18.0. Differences between chow diet and fish oil diet groups were evaluated by one-way ANOVA followed by Tukey's post hoc test. The difference was considered significant when p < 0.05. The area under the concentration–time curve (AUC) was calculated using the trapezoidal rule in GraphPad Prism 5.
Results and discussion
Effects of fish oil on plasma concentrations of choline, TMA, and TMAO in mice after choline chloride gavage
Choline is a trimethylamine-containing compound, which is part of the head group of phosphatidylcholine. It is an essential nutrient contributing to cell membrane function, widely present in a variety of foodstuffs. The serum concentration–time profiles showed that the peak value of choline reached at 1 h in two groups. Interestingly, the AUC and maximum concentration (Cmax) in fish oil diet group were significantly higher than chow diet group (Fig. 1a and Table 1). Previous reports showed that abundant long-chain PUFAs particularly DHA (22
:
6) increased the amount of PC via increasing the synthesis of phosphatidylethanolamine (PE) and promoting phosphatidylethanolamine N-methyltransferase (PEMT) pathway.15,16 Enhanced concentration of PC would be expected to inhibit the cytidine diphosphate choline (CDP-choline) pathway as well as facilitate the hydrolysis of PEMT.17 Accordingly, increased plasma choline levels might be achieved by adjusting the synthesis of lecithin in CDP-choline and PEMT pathway. West et al.18 also found that consumption of eggs enriched with n-3 PUFAs increased circulating concentrations of free choline compared with the egg-free control.
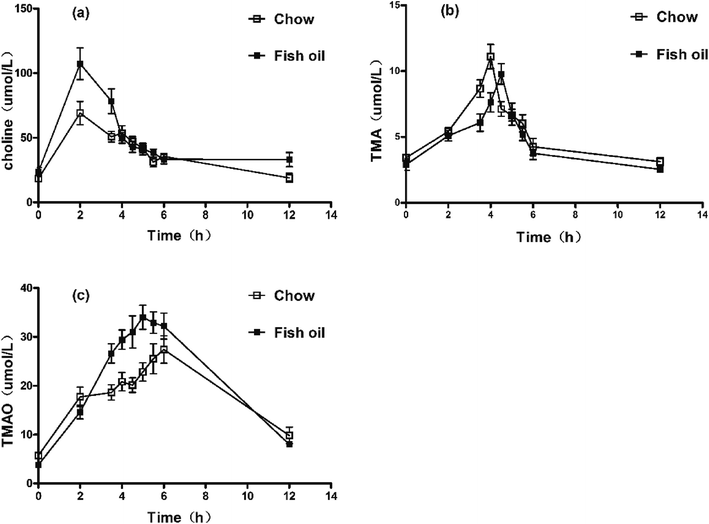 |
| Fig. 1 Concentration–time curves of serum choline, TMA, and TMAO after choline chloride oral administration. (a) Concentration–time curves of plasma choline. (b) Concentration–time curves of plasma TMA. (c) Concentration–time curves of plasma TMAO. | |
Table 1 Pharmacokinetic parameters of choline, TMA, TMAO plasma concentrations after choline chloride oral administrationa
|
Parameters |
Chow diet |
Fish oil |
Notes: data are presented as mean ± SD; significant difference compared to the chow diet group determined by one-way ANOVA. *p < 0.05; **p < 0.01; ***p < 0.001. |
Choline |
AUC (μmol L−1 h−1) |
450.0 ± 62.7 |
583.6 ± 74.9*** |
Tmax (h) |
2 |
2 |
Cmax (μmol L−1) |
69.1 ± 9.0 |
107.3 ± 12.2*** |
TMA |
AUC (μmol L−1 h−1) |
58.0 ± 5.4 |
54.7 ± 4.1 |
Tmax (h) |
4 |
4.5 |
Cmax (μmol L−1) |
11.1 ± 0.9 |
9.8 ± 0.8* |
TMAO |
AUC (μmol L−1 h−1) |
217.8 ± 18.1 |
248.4 ± 20.3* |
Tmax (h) |
6 |
5 |
Cmax (μmol L−1) |
27.4 ± 2.8 |
34.0 ± 2.5** |
It is well known that TMA is normally formed in higher organisms mainly from dietary choline, which is widely present in eggs, soybean and so on.19 TMA production was related to composition and abundance of individual gut microbiome.20 The serum concentration–time profile of TMA in mice after oral administration was illustrated in Fig. 1b and Table 1. Results showed the Cmax of TMA was significantly lower in fish oil diet group compared to chow diet group. In addition, the Tmax of TMA in fish oil diet group was later than that of fish oil-free control. Interestingly, more TMAO was produced in plasma of fish oil diet group in comparison with chow diet group (Fig. 1c and Table 1). Previous results showed that long-term diet was able to alter intestinal microflora. The reduction of L-carnitine ingestion by vegans and vegetarians might contribute to reducing TMA and TMAO levels compared to the omnivore.21 Dietary 3,3-dimethyl-1-butanol (DMB) decreased proportions of Clostridiales meanwhile increased the Bacteroidetes, which exhibited significantly inverse associations with TMA levels thereby reducing plasma TMAO.22 Fish oil as functional foods with a high content of n-3 PUFAs also could suppress the growth of Clostridiales and increase the abundance of Bacteroidetes.23 Above results indirectly implied that the low production of TMA in vivo might be attributed to the different intestinal flora resulting from the fish oil with high content of DHA.
Effects of fish oil on plasma concentrations of TMA and TMAO in mice after intraperitoneal injection
Food components affect TMAO metabolic process mainly through two aspects. One is that long-term dietary habits modulate the composition of the intestinal microbes.24 The other is to influence hepatic FMOs expression. According to our above results of pharmacokinetics, we hypothesized that the rise of TMAO might be related to hepatic FMOs activities. Therefore, the TMA hydrochloride was administered to caudal vein to eliminate the disturbance of intestinal flora in the metabolic process of TMAO. Results showed that the concentrations of TMA in blood of both groups reached to the maximum values at 0.5 h, respectively. Interestingly, no significant differences were observed in both groups although the Cmax and AUC of TMA in fish oil diet group were slightly lower than chow diet group after i.p injection (Fig. 2a and Table 2). In addition, the AUC of TMAO in fish oil diet group was significantly higher than the chow diet group. Importantly, the TMAO level at the last point in plasma of fish oil diet group was statistically lower in comparison with that of chow diet group (Fig. 2b and Table 2). The above results indicated that the hepatic FMOs activities might be changed after supplementation with fish oil, and subsequently TMAO could rapidly be metabolized in vivo.
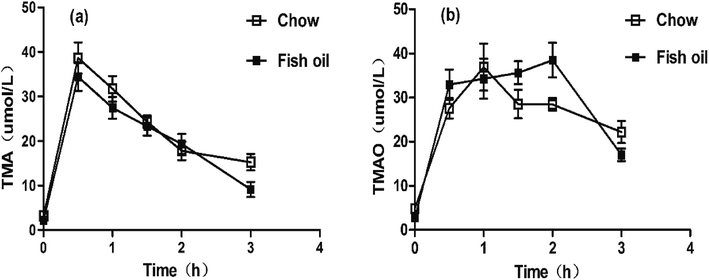 |
| Fig. 2 Concentration–time curves of serum TMA and TMAO after i.p administration. (a) Concentration–time curves of plasma TMA. (b) Concentration–time curves of plasma TMAO. | |
Table 2 Pharmacokinetic parameters of TMA and TMAO plasma concentrations after intraperitoneal injection administrationa
|
Parameters |
Chow diet |
Fish oil |
Notes: data are presented as mean ± SD; significant difference compared to the chow diet group determined by one-way ANOVA. *p < 0.05. |
TMA |
AUC (μmol L−1 h−1) |
68.9 ± 5.1 |
62.0 ± 4.5 |
Tmax (h) |
0.5 |
0.5 |
Cmax (μmol L−1) |
38.6 ± 3.5 |
34.4 ± 3.2 |
TMAO |
AUC (μmol L−1 h−1) |
80.1 ± 6.5 |
89.4 ± 7.1* |
Tmax (h) |
1 |
2 |
Cmax (μmol L−1) |
36.8 ± 5.3 |
38.4 ± 3.8 |
Effects of fish oil on FMOs gene expressions and enzyme activities in liver
FMO is the NADPH-dependent enzyme which catalyze the oxidative metabolism involved in a wide array of foreign chemicals including therapeutic drugs dietary-derived compounds.24 The activity of FMO in the organization is affected by many factors including diets, hormones, etc.25,26 TMAO can be produced by FMO family including FMO1, FMO2, FMO3 and FMO5 in liver, in which FMO3 is the most important TMA N-oxygenase.27 Our findings suggested that FMO3 mRNA level in fish oil diet group was significantly higher than that of chow diet group (Fig. 3), which was consistent with previous study about the effect of dietary ingredients on FMOs expression levels. Baneshi et al. found that lecithin (i.p) could significantly increase the mRNA expression of FMO3 in the liver of mice.28
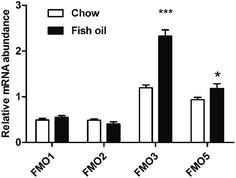 |
| Fig. 3 Hepatic FMO1, FMO2, FMO3, and FMO5 gene expressions in mice at 3 h after treatment with trimethylammonium chloride as a single dose intraperitoneal injection. Real time PCR analyses of FMO1, FMO2, FMO3, and FMO5 genes were normalized with 18S. Date expressed as mean ± SD (n = 5) *p < 0.05; ***p < 0.001. | |
The enzyme activities of FMOs family were also determined, and results showed that long-term supplementation with fish oil diet containing high content of DHA could increase hepatic FMOs activities (Fig. 4a). Previous study suggested the intake of fish oil at a high dose up-regulated CYP3A2 gene expression and CYP3A activity in liver.29 Fish oil was also proved to increase the activities of CYP1A1 and CYP3A1 containing drug-metabolizing capacity.30 FMO was similar to cytochrome P450 (CYP) in the aspects including the location, co-factor requirement and activity toward xenobiotics. In addition, FMO is involved in a wide range of substrates including TMA, benzydamine compared with CYP.31 A number of studies have suggested that FMO was beneficial ranging from drug metabolism to human disease. FMO as crucial hepatic metabolic enzymes generally converted the parent amines to compounds with polarity, which are readily excreted.32 For example, if MPTP is not oxygenated by FMO, it could undergo other oxidase-catalyzed metabolism to the potent neurotoxins MPDP+ and MPP+, and result in parkinsonism.33 Moreover, elevated FMO enzyme activity can rapidly metabolize a large number of TMA to TMAO, then prevent trimethylamine rising and escaping via the breath and sweat, which indicates that FMO-mediated N-oxygenation of tertiary amines exhibits important significance for human heath.34,35 Mutations in the human FMO3 gene are responsible for trimethylamine urine.36 Polymorphisms in FMO3 increased efficacy to prevent polyposis in familial adenomatous polyposis.37
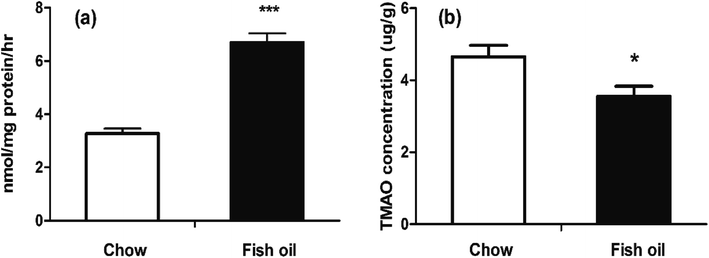 |
| Fig. 4 FMOs activity and TMAO levels in liver. (a) Liver FMOs activity in liver between chow diet and fish oil. (b) TMAO levels in liver at the sacrificed point time. The results represent mean value ± SD. (n = 5) *p < 0.05; ***p < 0.001. | |
Some researches suggested that high TMAO circulation level was a risk factor for atherosclerosis, but meanwhile a number of studies also provided evidence for its important biological functions in numerous organisms, ranging from bacteria to mammals.38 For example, increased TMAO levels might be beneficial on relieving vascular injury in hemodialysis patients and implied the therapeutic potential in epidermolysis bullosa.39,40 Our results also indicated that fish oil diet group had lower TMAO accumulative levels in liver than chow diet group at the sacrificed time (Fig. 4b). In plasma, TMAO levels were briefly elevated after a large amount of TMAO precursors administration then rapidly declined. In terms of FMO's drug metabolism, the temporary elevation of TMAO levels in fish oil might imply that fish oil consumption was beneficial for the metabolism of exogenous substances. Hence, we speculated that the accelerated metabolism of TMAO by elevated FMO enzyme activities in fish oil diet group could contribute to the excretion of exogenous harmful substances. In our study, we believed that plasma TMAO level was transient higher in fish oil group was not necessarily pernicious.
Previous studies have demonstrated that choline could be metabolized to TMA by gut microbiota. Then, TMA was absorbed into the circulation and further metabolized by FMOs in the host liver to generate TMAO. Therefore, the levels of intestinal flora and FMOs in vivo played important roles in the content of TMAO in plasma. The present work proved that the rapid metabolism of TMAO might be attributed to the intestinal flora inducing low production of TMA and the increase of FMOs activities following long-term fish oil supplementation (Fig. 5).
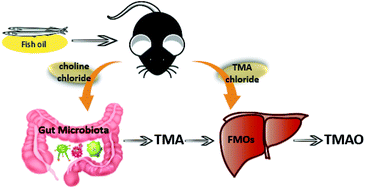 |
| Fig. 5 Schematic pathways of proposed hypothesis that fish oil could affect TMAO metabolic process through gut microbiota and FMOs. | |
Conclusions
In conclusion, this study first evaluated the effects of supplementation with fish oil on TMAO metabolism by mouse model. Results showed that fish oil increased choline circulation levels and suppressed the production of TMA after choline chloride oral gavage. Additionally, fish oil accelerated TMAO metabolism via enhancing FMOs activities involved in fatty acid oxidation and drug detoxification. Consequently, fish oil supplementary can make it more easily to metabolize exogenous toxic substances, which is beneficial to human health.
Conflicts of interest
There are no conflicts to declare.
Acknowledgements
This work was funded by the National Natural Science Foundation of China (No. 31371757), State Key Program of National Natural Science of China (No. 31330060), Fundamental Research Funds for the Central Universities (No. 201762028) and National Natural Science Foundation of China-Shandong Joint Fund for Marine Science Research Centers (U1606403).
References
- Z. Wang, E. Klipfell, B. J. Bennett, R. Koeth, B. S. Levison, B. Dugar, A. E. Feldstein, E. B. Britt, X. Fu, Y. M. Chung, Y. Wu, P. Schauer, J. D. Smith, H. Allayee, W. H. Tang, J. A. DiDonato, A. J. Lusis and S. L. Hazen, Nature, 2011, 472, 57–63 CrossRef CAS PubMed.
- C. Missailidis, J. Hallqvist, A. R. Qureshi, P. Barany, O. Heimburger, B. Lindholm, P. Stenvinkel and P. Bergman, PLoS One, 2016, 11, e0141738 Search PubMed.
- R. Xu, Q. Q. Wang and L. Li, BMC Genomics, 2015, 16, S4 CrossRef PubMed.
- D. Fennema, I. R. Phillips and E. A. Shephard, Drug Metab. Dispos., 2016, 44, 1839–1850 CrossRef CAS PubMed.
- S. Craciun and E. P. Balskus, Proc. Natl. Acad. Sci. U. S. A., 2012, 109, 21307–21312 CrossRef CAS PubMed.
- K. A. Romano, E. I. Vivas, D. Amador-Noguez and F. E. Rey, mBio, 2015, 6, e02481 CrossRef PubMed.
- M. L. Chen, L. Yi, Y. Zhang, X. Zhou, L. Ran, J. Yang, J. D. Zhu, Q. Y. Zhang and M. T. Mi, mBio, 2016, 7, e02210 CAS.
- W. K. Wu, S. Panyod, C. T. Ho, C. H. Kuo, M. S. Wu and L. Y. Sheen, J. Funct. Foods, 2015, 15, 408–417 CrossRef CAS.
- M. Dambrova, E. Skapare-Makarova, I. Konrade, O. Pugovics, S. Grinberga, D. Tirzite, R. Petrovska, I. Kalvins and E. Liepins, J. Clin. Pharmacol., 2013, 53, 1095–1098 CrossRef CAS PubMed.
- R. Obeid, H. M. Awwad, S. H. Kirsch, C. Waldura, W. Herrmann, S. Graeber and J. Geisel, Mol. Nutr. Food Res., 2017, 61, 2 Search PubMed.
- X. Gao, C. Jiang, J. Xu, T. Yanagita, C. Xue and Y. Wang, Biosci., Biotechnol., Biochem., 2016, 80, 1 Search PubMed.
- C. Wang, W. S. Harris, M. Chung, A. H. Lichtenstein, E. M. Balk, B. Kupelnick, H. S. Jordan and J. Lau, Am. J. Clin. Nutr., 2006, 84, 5 CAS.
- X. Gao, J. Xu, C. Jiang, Y. Zhang, Y. Xue, Z. Li, J. Wang, C. Xue and Y. Wang, Food Funct., 2015, 6, 1117–1125 CAS.
- D. M. Shih, Z. Wang, R. Lee, Y. Meng, N. Che, S. Charugundla, H. Qi, J. Wu, C. Pan and J. M. Brown, J. Lipid Res., 2015, 56, 22–37 CrossRef CAS PubMed.
- U. I. Richardson and R. J. Wurtman, Biochim. Biophys. Acta, 2007, 1771, 558–563 CrossRef CAS PubMed.
- C. J. Pynn, N. G. Henderson, H. Clark, G. Koster, W. Bernhard and A. D. Postle, J. Lipid Res., 2011, 52, 399–407 CrossRef CAS PubMed.
- Z. Li and D. E. Vance, J. Lipid Res., 2008, 49, 1187–1194 CrossRef CAS PubMed.
- A. A. West, Y. Shih, W. Wang, K. Oda, K. Jaceldo-Siegl, J. Sabate, E. Haddad, S. Rajaram, M. A. Caudill and B. Burns-Whitmore, J. Acad. Nutr. Diet., 2014, 114, 1594–1600 CrossRef PubMed.
- H. Wolrath, B. Ståhlbom, A. Hallén and U. Forsum, APMIS, 2010, 113, 513–516 CrossRef PubMed.
- C. E. Cho, S. Taesuwan, O. V. Malysheva, E. Bender, N. F. Tulchinsky, J. Yan, J. L. Sutter and M. A. Caudill, Mol. Nutr. Food Res., 2017, 61, 1 Search PubMed.
- R. A. Koeth, Z. Wang, B. S. Levison, J. A. Buffa, E. Org, B. T. Sheehy, E. B. Britt, X. Fu, Y. Wu, L. Li, J. D. Smith, J. A. DiDonato, J. Chen, H. Li, G. D. Wu, J. D. Lewis, M. Warrier, J. M. Brown, R. M. Krauss, W. H. Tang, F. D. Bushman, A. J. Lusis and S. L. Hazen, Nat. Med., 2013, 19, 576–585 CrossRef CAS PubMed.
- Z. Wang, A. B. Roberts, J. A. Buffa, B. S. Levison, W. Zhu, E. Org, X. Gu, Y. Huang, M. Zamanian-Daryoush, M. K. Culley, A. J. DiDonato, X. Fu, J. E. Hazen, D. Krajcik, J. A. DiDonato, A. J. Lusis and S. L. Hazen, Cell, 2015, 163, 1585–1595 CrossRef CAS PubMed.
- H. N. Yu, J. Zhu, W. S. Pan, S. R. Shen, W. G. Shan and U. N. Das, Arch. Med. Res., 2014, 45, 195–202 CrossRef CAS PubMed.
- V. Tremaroli and F. Backhed, Nature, 2012, 489, 242–249 CrossRef CAS PubMed.
- J. R. Cashman, Y. Xiong, J. Lin, H. Verhagen, P. G. Van, P. J. van Bladeren, S. Larsensu and D. E. Williams, Biochem. Pharmacol., 1999, 58, 1047 CrossRef CAS PubMed.
- S. Coecke, G. Debast, I. R. Phillips, A. Vercruysse, E. A. Shephard and V. Rogiers, Biochem. Pharmacol., 1998, 56, 1047–1051 CrossRef CAS PubMed.
- D. H. Lang, C. K. Yeung, R. M. Peter, C. Ibarra, R. Gasser, K. Itagaki, R. M. Philpot and A. E. Rettie, Biochem. Pharmacol., 1998, 56, 1005–1012 CrossRef CAS PubMed.
- A. Mohammadi, M. Baneshi, Z. Vahabzadeh and T. Khalili, Biosci., Biotechnol. Res. Asia, 2016, 13, 1797–1803 CrossRef.
- T. Yamazaki, T. Ohki, H. Taguchi, A. Yamamoto, M. Okazaki, T. Sakamoto, A. Mitsumoto, Y. Kawashima and N. Kudo, Fundam. Toxicol. Sci., 2015, 2, 127–135 CrossRef CAS.
- H. W. Chen, C. W. Tsai, J. J. Yang, C. T. Liu, W. W. Kuo and C. K. Lii, Br. J. Nutr., 2003, 89, 189–200 CrossRef CAS PubMed.
- E. Störmer, I. Roots and J. Brockmöller, Br. J. Clin. Pharmacol., 2000, 50, 553 CrossRef.
- J. R. Cashman, Biochem. Biophys. Res. Commun., 2005, 338, 599–604 CrossRef CAS PubMed.
- T. Mushiroda, N. Ariyoshi, T. Yokoi, E. Takahara, O. Nagata, H. Kato and T. Kamataki, Chem. Res. Toxicol., 2001, 14, 228–232 CrossRef CAS PubMed.
- S. C. Mitchell and R. L. Smith, Chem. Senses, 2016, 41, 275–279 CrossRef PubMed.
- S. K. Krueger and D. E. Williams, Pharmacol. Ther., 2005, 106, 357–387 CrossRef CAS PubMed.
- J. Zhang, Q. Tran, V. Lattard and J. R. Cashman, Pharmacogenetics, 2003, 13, 495–500 CrossRef CAS PubMed.
- I. M. Hisamuddin, M. A. Wehbi, A. Chao, H. W. Wyre, L. M. Hylind, F. M. Giardiello and V. W. Yang, Clin. Cancer Res., 2004, 10, 8357–8362 CrossRef CAS PubMed.
- M. Ufnal, A. Zadlo and R. Ostaszewski, Nutrition, 2015, 31, 1317–1323 CrossRef CAS PubMed.
- K. Fukami, S. Yamagishi, K. Sakai, Y. Kaida, M. Yokoro, S. Ueda, Y. Wada, M. Takeuchi, M. Shimizu, H. Yamazaki and S. Okuda, J. Cardiovasc. Pharmacol., 2015, 65, 289–295 CrossRef CAS PubMed.
- J. C. Chamcheu, H. Navsaria, I. Pihllundin, M. Liovic, A. Vahlquist and H. Törmä, J. Invest. Dermatol., 2011, 131, 1684 CrossRef CAS PubMed.
Footnote |
† Electronic supplementary information (ESI) available. See DOI: 10.1039/c7ra10248h |
|
This journal is © The Royal Society of Chemistry 2017 |