DOI:
10.1039/C7RA10103A
(Paper)
RSC Adv., 2017,
7, 55131-55140
Visible-light-mediated antifungal bamboo based on Fe-doped TiO2 thin films
Received
11th September 2017
, Accepted 23rd November 2017
First published on 5th December 2017
Abstract
TiO2-based photocatalytic disinfection has been proved as one of the feasible approaches for the control and inhibition of the growth of mould fungi on woody materials. However, the wide band gap of TiO2 (3.2 eV) limits the efficient absorption of sunlight in the visible region. In this study, visible-light-mediated antifungal bamboo based on Fe-doped TiO2 thin films was successfully fabricated through a facile one-step homogeneous precipitation method. XRD studies confirmed that all of the as-prepared TiO2 nanoparticles on the bamboo surface were anatase phase. Fe-doped TiO2 thin films were found to grow on the bamboo surface by the self-aggregation of nanoparticles with an average diameter of about 9.7 nm and a surface area of about 90.96 m2 g−1. The Fe-doped TiO2/bamboo samples looked identical to original bamboo as there was no essential effect on the optical properties of the bamboo surface. Moreover, the antifungal activity of the as-prepared samples against mould fungi was investigated under natural weather conditions. Compared with original bamboo and TiO2/bamboo, the Fe-doped TiO2/bamboo exhibited much higher inhibition ability to mould fungi under the natural environment, which is due to the fact of the red shift of the absorption edge of the Fe-doped TiO2 nanostructures on the bamboo surface. UV-vis DRS also revealed that the band gap of Fe-doped TiO2 nanostructures was obviously decreased, extending the light response of TiO2 from ultraviolet to the visible light region. The research result may provide a general and effective approach to prepare visible-light-driven photocatalysts used for inhibition of the growth of mould fungi on the surface of bamboo.
1. Introduction
Titanium dioxide (TiO2) has been widely applied in various fields such as photocatalytic systems, antimicrobial materials, self-cleaning coatings, hydrogen production, and solar cells due to its remarkable chemical and physical properties.1–5 Over the past several years, TiO2 as an important semiconductor material and photocatalyst has received a lot of attention because of its biological and chemical inertness, nontoxicity, relatively low cost, long-term stability, and high photocatalytic efficiency. The US Food and Drug Administration (FDA) also approved its use in human foods, drugs, cosmetics, and use in the food industry, as a nontoxic material.6 Since the early research of Matsunaga et al.7 reported the first application of pure TiO2 for the photocatalytic disinfection of microorganisms, research work on TiO2-based photocatalysts for various microorganism disinfections, including viruses,8 bacteria,9 fungi,10 algae,11 and protozoa12 has been carried out. It is widely considered that photocatalytic microorganism disinfection depends on the interaction between microorganisms and reactive oxygen species (ROS) generated from photocatalysts under light illumination, such as ˙OH and ˙O2−, which can kill the microorganisms.13 At present, this capability is very desirable for the inhibition to the growth of mould fungi on bamboo surface. Bamboo as a rapidly renewable sustainable resource, can be available for building, flooring, roofing, fabrics and cloth, pulp and paper, and charcoal. The traditional markets for bamboo products were dominated by the handicrafts, chopsticks, bamboo blinds, and daily necessities. With the development of modern processing techniques, bamboo can be transformed into many new products such as laminated furniture, flooring, and decoration constructions that can compete directly with wood products either in quality or in price.14 However, bamboo is more susceptible to mould growth than wood, which allow them to adapt a wide range of humidity and temperature.15 As known, TiO2 is only active under UV light irradiation because of their wide band gap energy (3.2 eV), which only about 5% of solar light can be utilized. Thus, it is high time to develop novel TiO2-based photocatalysts that can yield high photocatalytic activity under visible light illumination so that a greater portion of the solar spectrum could be utilized.
Numerous efforts have been carried out in the past decades to develop the visible-light-driven TiO2-based photocatalysts by impurity doping, metallization, sensitization and coating, etc.16,17 Among these techniques, metal ion doped TiO2 has been extensively studied due to not only the expanded spectral response but also the improved photocatalytic activity.18–20 Choi et al.21 demonstrated that metal ions (Fe3+, Ag+, Co2+, V3+, Rb+, Ni2+, Cu2+, Ru3+, etc.) doping results in a red-shift of the photophysical response of TiO2. Among various dopants, Fe3+ is the suitable candidate because of their band-gap (∼2.6 eV) and similar size to that of Ti4+, and Fe3+-doped nanostructured TiO2 also showed an antibacterial behavior.22 Its unique half-filled electronic configuration, which might narrow the energy gap through the formation of new intermediate energy levels and also diminish recombination of electrons and holes by capturing photogenerated carriers.23 Up to now, Fe3+ doped nanostructured TiO2 has rarely been used for photocatalytic disinfection of specific microorganisms, let alone use for disinfection the mould fungi of bamboo surface. Meanwhile, the antimicrobial activities of Fe-doped TiO2 thin films have been studied by a few groups of researchers.24–27 However, due to its much larger size and thicker cell wall/membrane, the mould fungi usually have stronger resistance to ROS attack than bacteria or viruses. Thus, effective alternatives to chemical fungicides are difficult to develop. Because of the demonstrated strong photocatalytic disinfection effect under visible light illumination and environmental friendliness of Fe-doped nanostructured TiO2, they might provide an efficient fungicidal agent for the inhibition to the growth of mould fungi on woody materials.
To the best of our knowledge, it has been found that no reported studies are available on the application of Fe-doped TiO2 nanoparticles to inhibit fungal growth on the surface of bamboo in the presence of solar light. Herein, we present a facile method to anchor the Fe-doped TiO2 thin films on the surface of bamboo. The effect of Fe3+ dopants on the morphology, structure, composition, surface area, and band gap of TiO2 nanoparticles on the surface of bamboo was also investigated. The visible-light-mediated photocatalytic inhibition of mould fungi using the Fe-doped TiO2 nanoparticles has been verified in the absence and presence of solar light.
2. Experimental
2.1. Materials
All the chemical agents were analytical grade and used without further purification. Deionized (DI) water was used in the entire experiment. Moso bamboo specimens with the sizes of 50 mm × 20 mm × 6 mm were ultrasonically cleaned in DI water for 30 min, and then dried at 60 °C for 24 h.
2.2. Synthesis of Fe-doped TiO2 thin films on bamboo surface
Ammonium fluotitanate ((NH4)2TiF6) was used as the titanium source. Urea (CO(NH2)2) which releases ammonia via forced hydrolysis in DI water, was used to control the precipitation reaction. Iron nitrate nonahydrate (Fe(NO3)3·9H2O) was used as a source for Fe3+ doping. In a typical synthesis process, (NH4)2TiF6 (1.5 g), CO(NH2)2 (15.0 g) and Fe(NO3)3·9H2O (0.0606 g) were dissolved in 500 mL of DI water under vigorous magnetic stirring. The nFe/nTi molar ratio is 2.0 at%. After stirring for 5 min at room temperature (RT), bamboo samples were subsequently placed into the reaction solution. The final solution was heated to 90 °C under magnetic stirring for 2 h. After cooling naturally to RT, the bamboo specimens were taken out and cleaned in DI water by an ultrasonic bath for 3 min. The resultant particles in reaction solution were recovered via centrifugation, and washed repeatedly with DI water. At last, all the samples were dried at 80 °C for 24 h. The pure TiO2 nanoparticles were prepared on the surface of bamboo with the same procedure described above but without the addition of Fe(NO3)3·9H2O. In this paper, the undoped TiO2/bamboo was labeled as TiO2B. The Fe-doped TiO2/bamboo was labeled as FeTiO2B and pure Fe-doped TiO2 particle was labeled as FeTiO2.
2.3. Characterization
The X-ray diffraction (XRD) analysis was performed on a Rigaku D/Max 2500 X-ray diffractometer with Cu Kα radiation (λ = 1.5418 Å) at a generator voltage of 40 kV and a generator current of 30 mA with a scanning speed of 4° min−1 from 10° to 90°. The compositions of the products were inferred from the X-ray photoelectron spectroscopy (XPS, PHI Quantera XPS) on a PHI-5000C ESCA system with Al Kα X-rays as the excitation source. The surface morphologies of the samples were observed by scanning electron microscopy (SEM, JEOL JSM-7610F, Japan). Specific surface areas of the as-prepared samples were measured based on the linear portion of the Brunauer–Emmett–Teller (BET, Quantachrome of Micromeritics ASAP 2020 instruments, USA). The UV-vis absorption spectra of the samples were recorded using a UV-vis spectrophotometer (UV-vis, Hitachi U-3900, Japan).
2.4. Mould resistance test
The field test was performed in Hangzhou city, China (N 30°17′25′′, E 120°6′53′′, 10 m above sea level). In order to keep the same environment, all specimens were placed horizontally, 1.2 m above the ground, in an outdoor test field fully exposed to sun, rain and wind (Fig. 1a). The bamboo samples were exposed outdoors from Jun 4th to Jul 3rd (2017), which are easily to mould in the rainy season. Temperature, relative humidity, and precipitation at the test site were recorded as shown in Fig. 1b–d. It is noteworthy that there is no sunny day in this period of time and the ultraviolet index is quite low at daytime. The control group was carried out in the same condition without natural light. The mould coverage on bamboo substrate was evaluated according to EN 927-3 (2000).28
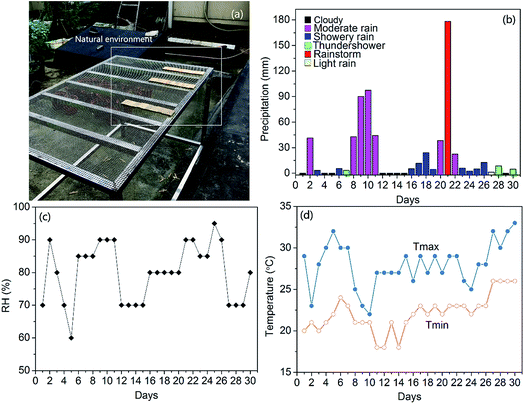 |
| Fig. 1 (a) Field test. (b) Precipitation, (c) relative humidity, and (d) temperature at the test site from Jun 4th until Jul 3rd. | |
3. Results and discussion
Mildew is an urgent problem in the bamboo processing industry. Especially in outdoor applications, bamboo is very easy to mould in the rainy season. The effective fungicidal methods used for bamboo rely heavily on synthetic chemical pesticide, such as sodium pentachlorophenate.29 However, it is usually hazardous to animals, humans, and the environment. Furthermore, mould fungi have developed resistance to some current fungicides, which is always hard to inhibit fungal growth of bamboo. Thus, alternative antifungal techniques used for bamboo need to be developed, which should overcome the fungicide resistance for a better fungicidal effect. Since the photocatalytic disinfection of microorganism using TiO2 was first successful reported by Matsunaga et al.7 Considerable studies have been conducted on TiO2-based photocatalysts for various microorganism disinfections. However, the large band gap of TiO2 and low quantum efficiency restrict its wide application. Thus, it is high time to develop novel photocatalysts that can yield high photocatalytic activity under visible light so that a greater portion of the solar spectrum could be utilized. As depicted in Fig. 2, the Fe-doped TiO2 nanoparticles were successfully fabricated on the surface of bamboo. By doping the Fe ions in TiO2 matrix results in significantly extending for its optical responses from UV to visible region. The resulted Fe-doped TiO2/bamboo samples exhibit powerful ability to against mould fungi under natural environment than the original bamboo and TiO2/bamboo, which will be demonstrated by fungi inhibition tests in subsequent paragraphs.
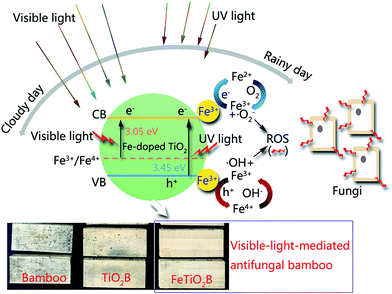 |
| Fig. 2 Bamboo decorated with Fe-doped TiO2 thin films for fungi inhibition. | |
3.1. Phase structures
XRD spectra of the as-prepared pure TiO2 nanoparticles and FeTiO2 nanoparticles were presented in Fig. 3. It is observed that the diffraction peaks of two samples are ascribed to the peaks of anatase TiO2 phase (JCPDS card no. 71-1167). It should be noted that no Fe2O3 or FexTiOy peak could be found in the XRD spectra. This result indicates that there has been virtually no phase change in TiO2 during the doping process. The crystal sizes of TiO2 nanoparticles and FeTiO2 nanoparticles were estimated using the Scherrer equation:30 |
 | (1) |
where β is the half-height width of the diffraction peak of anatase, K = 0.89 is a coefficient, θ is the diffraction angle, and λ is the X-ray wavelength corresponding to the Cu-Kα irradiation (λ = 1.5406 Å). By using the Scherrer equation, the crystallite sizes of TiO2 and FeTiO2 are calculated to be 12.5 and 9.7 nm, respectively. The average crystallite size of FeTiO2 products is lower than that of pure TiO2, which indicates the occurrence of a slight lattice distortion in the TiO2 structure. The dimension decrease in crystallite size may be caused by a number of defects in the anatase crystallites produced by the substitution of part of the Ti(IV) site by Fe(III) cations.31 In addition, the spacing between the (101) plane fringes of both samples was measured to be approximately 0.35 nm. No distinct spacing change was observed in FeTiO2, suggesting that Fe was incorporated into the anatase crystal structure without changing the average dimension of the unit cell.32
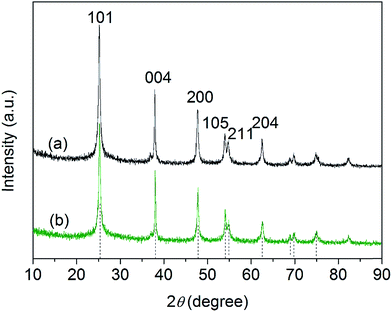 |
| Fig. 3 XRD patterns of (a) pure TiO2 and (b) FeTiO2 nanoparticles. | |
3.2. XPS analysis
In order to investigate the chemical composition of Fe-doped TiO2 on the surface of bamboo and determine the chemical status of Fe elements in the doped products, the as-prepared samples were measured by XPS. Fig. 4a presents the XPS survey spectra of the pristine bamboo, TiO2B, and FeTiO2B. It can be observed that the original bamboo contains C, O, and N elements. After decorating with pure TiO2 nanoparticles, two new peaks of Ti and F elements were appeared on the surface of bamboo. It should be noted that the doped TiO2 sample not only contains Ti, F, C, N and O elements, but also a small amount of Fe elements, which come from the Fe doping process. The C element can be ascribed to the bamboo substrate or the adventitious carbon based contaminant.
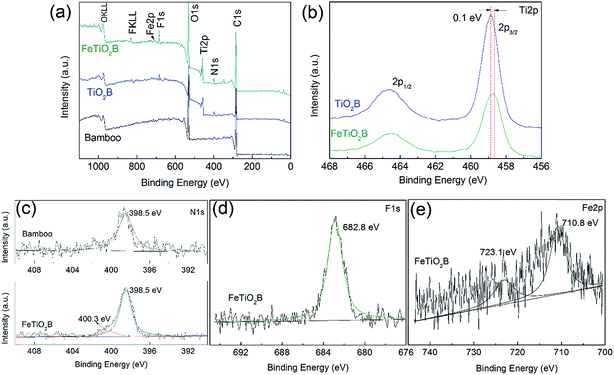 |
| Fig. 4 (a) XPS survey spectra of the original bamboo, TiO2B, and FeTiO2B; high-resolution XPS spectra of (b) Ti 2p region of TiO2B and FeTiO2B, (c) N 1s region of original bamboo and FeTiO2B, and (d) F 1s and (e) Fe 2p region of FeTiO2B, respectively. | |
The high-resolution XPS spectra of the Ti 2p of TiO2B and FeTiO2B samples are presented in Fig. 4b. The binding energy difference of 5.7 eV for the Ti 2p3/2 peak and Ti 2p1/2 peak of both products revealed a valence state of +4 for Ti, indicating that Ti was mostly Ti4+. It should be noted that Ti 2p peak at 458.7 eV of FeTiO2B shifted positively by 0.1 eV in comparison with that of the Ti 2p peak in TiO2B sample (458.8 eV). The shift of Eb could be ascribed to the interaction between host ion Ti4+ and foreign Fe-ions and the formation of the Ti–O–Fe bond in FeTiO2B sample. Fig. 4c displays the XPS spectra of N 1s for original bamboo and FeTiO2B. We can see that the peak at approximately 398.5 eV was assigned to the nitrogen in C–N or O–N in original bamboo substrate. For FeTiO2B sample, a N 1s peak of weak intensity is observed at about 400.3 eV, which can be assigned to the surface adsorbed ammonium ions.33 To investigate whether F atoms were incorporated in the titania lattice or not, the high-resolutions XPS spectrum of F 1s for FeTiO2B sample was recorded in Fig. 4d. Only one peak was detected in FeTiO2B sample. The peak at 682.8 eV is due to the adsorbed F− ions on the surface of TiO2. The large amount of surficial F− ions may occur not only by electrostatic adsorption but also by nucleophilic substitution reaction of F− ions and surficial hydroxyl groups.34 The high-resolution XPS spectrum of the Fe 2p is presented in Fig. 4e. The peaks of Fe 2p3/2 and Fe 2p1/2 shown in Fig. 4e are located at 710.8 and 723.1 eV, respectively, corresponding well to the binding energy of Fe3+. The low intensity of the Fe 2p peaks suggests that the incorporated Fe3+ ions do not accumulate at the nanoparticles surface layers but uniformly distribute in the titania matrix.
3.3. Morphological structures
The SEM images of the original bamboo, TiO2B, and FeTiO2B are shown in Fig. 5. As shown in Fig. 5a, a typical SEM image of original bamboo surface, on which the microstructure of a longitudinal section of original bamboo whose vascular bundles were embedded in the matrix of ground parenchyma. A corresponding EDS spectrum for original bamboo was presented in Fig. 5a (inset). Only C, O, and Au elements could be detected from the spectra of the original bamboo. Au element originated from the coating layer used during the SEM observation, whereas C and O elements were from the bamboo. For TiO2B sample (Fig. 5b), numerous TiO2 nanoparticles can be observed on the surface of bamboo after precipitation process. This illustrates TiO2 nanoparticles were successfully attached on the surface of bamboo via the facile one-step homogeneous precipitation method. In the corresponding EDS spectrum of the inset in Fig. 5b, three new signals from N, F, and Ti were detected in the spectrum of the TiO2B sample. The N and F elements may be originated from the precursor (NH4)2TiF6, and no other elements were detected, implying that the thin films were primarily TiO2. Fig. 5c shows the SEM image of FeTiO2B at low magnification. More small nanoparticles are well-dispersed on bamboo surface. The bamboo surface is covered by a dense and even hierarchical film of FeTiO2 nanoparticles. From the corresponding high-magnification SEM image in Fig. 5d, the nanoparticles with the sizes ranging from 20 to 100 nm are clearly visible. However, by using the Scherrer equation (Table 1), the average crystallite sizes of FeTiO2 are calculated to be about 9.7 nm. Obviously, these nanoparticles were aggregated into larger particles on the surface of bamboo. As shown in Fig. 5d (inset), a signal from Fe was present in the spectrum of FeTiO2B sample after Fe doping, which further confirmed that the bamboo substrate was successfully modified by Fe-doped TiO2. Furthermore, the Fe-doped TiO2/bamboo sample looks identical to the original bamboo as there is no essential effect on the optical properties of the bamboo surface (Fig. 5, insets).
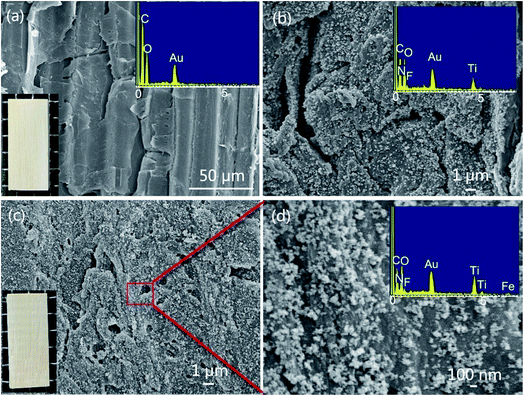 |
| Fig. 5 SEM images of the (a) original bamboo, (b) TiO2B, (c, d) FeTiO2B at different magnifications. The inset is the corresponding physical image and EDS spectra of bamboo samples. | |
Table 1 Physicochemical properties of the samples
Samples |
Crystallite size (nm) |
Plane fringes (101) (nm) |
BET surface area (m2 g−1) |
Average pore size (nm) |
Pore volume (m3 g−1) |
Band gap (eV) |
TiO2 |
12.5 |
0.35 |
73.64 |
11.99 |
0.217 |
3.20 |
FeTiO2 |
9.7 |
0.35 |
90.96 |
11.20 |
0.237 |
3.05 |
3.4. BET surface areas and pore distributions
The specific surface area of the as-prepared samples is measured using the BET method by N2 adsorption and desorption at 77 K. Fig. 6 displays the N2 adsorption–desorption isotherms of TiO2 and FeTiO2 products. Their pore size distributions are presented in the inset of Fig. 6. Both samples present a type-IV isotherm with an H3 type hysteresis loop with distinct hysteresis loops observed in the relative pressure range of 0.6–1.0, which indicated that the nanoparticles were mesoporous materials. The BET surface area of the FeTiO2 nanoparticles is calculated to be 90.96 m2 g−1. Moreover, the pore diameter distribution of the FeTiO2 indicates that the average pore diameter is about 11.20 nm. Furthermore, the pure TiO2 sample has similar isotherm and pore diameter distribution and BET surface area, which is listed in Table 1. The formation of nanoporous structure in the products may be attributed to the aggregation of TiO2 nanoparticles. Such nanoporous structures and large BET surface areas were crucial for photocatalysts, since they could supply transport channels for the molecules involved in the reaction and effectively separate photo-excited electron–hole pairs, reducing the recombination of electron–hole pairs.35
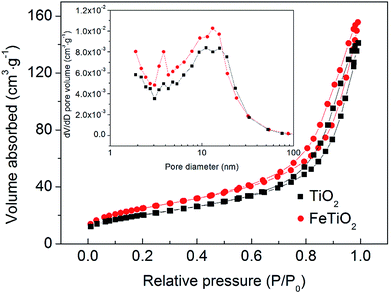 |
| Fig. 6 N2 adsorption–desorption isotherms and corresponding pore diameter distribution curves of the TiO2 and FeTiO2 samples. | |
3.5. UV-vis spectroscopy
UV-vis diffuse reflectance spectra of the pure TiO2 and FeTiO2 products were shown in Fig. 7. As shown in Fig. 7a, the pure TiO2 nanoparticles only exhibit adsorption in UV-light region. Obviously, the diffuse reflectance spectra of FeTiO2 presents a red shift and increases absorption in the visible-light range when Fe was doped into TiO2 lattice. Compared with the pure TiO2, these FeTiO2 products exhibit absorption in both UV and visible-light regions. The direct band gap energy can be estimated from a plot of (αhν)2 versus photon energy (hν).20 The absorption coefficient α and direct band gap Eg are related through the Kubelka–Munk function equation:36,37where ν is the frequency and h is Plank's constant. The band gap energies of the TiO2 and FeTiO2 products are shown in Table 1. The band gap energy of FeTiO2 is 3.05 eV, which is lower than that of pure TiO2 prepared by the same method (3.20 eV). The results indicated Fe doping can decrease the band gap energy of TiO2, extending the utilization of the visible light. This is due to the fact that the doping Fe ions could create a donor level above the original valence band of TiO2 to narrow the TiO2 band gap.38 This narrow band gap facilitated excitation of electrons from valence band to conduction band in FeTiO2 under visible light, resulting in enhanced photoactivity.
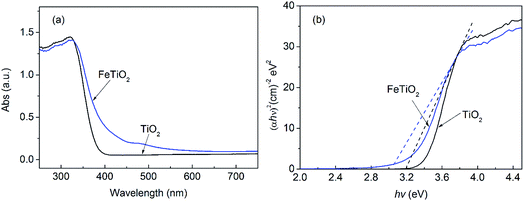 |
| Fig. 7 (a) UV-vis diffuse reflectance spectra and (b) plots of (αhν)2 vs. hν of the as-prepared samples. | |
3.6. Antifungal activity
Bamboo products without protection are very easy deteriorated by the complex interplay of microorganism and environment when exposed to the weather. Previous studies have shown that bamboo can allow mould fungi to adapt and survive over a wide range of humidity (63%∼) and temperature (5–30 °C).15 When the temperature and humidity were appropriate, spores of mould fungi on bamboo surface germinated and grew rapidly. As shown in Fig. 1, we can see that the humidity and temperature are suitable for growing mould fungi on the surface of bamboo in Hangzhou, especially in the rainy seasons. As expected, all model fungi grew differently according to the infected bamboo samples (Fig. 8). The biological colonization percentages by mould fungi on different bamboo samples are presented in Fig. 9. After two weeks, the original bamboo displayed poor resistance to mould fungi exposure and became rapidly covered with mould fungi (Fig. 8a). The mould fungi had created abundant mycelia on the sample surface and showed a fast propagation reaching a level of colonization of around 100%. In a similar manner, the mould fungi also attacked the TiO2B samples, but colonization evolves in a slower manner than the original bamboo (final degree of colonization round 75% for TiO2B samples). This is due to the fact that in the presence of TiO2 and UV light (solar light), ROS (such as hydroxyl radicals and superoxide radical anions) generated on the surface is considered responsible for the inactivation of the mould fungi. By contrast, the FeTiO2B samples showed efficiently resistance to mould growth. There is no mould fungi grown on the surface of bamboo (Fig. 8a). After one-month exposure under natural environment, we also saw no visible growth of FeTiO2B surface. The area of mould infection of FeTiO2B samples was zero (Fig. 9). However, we found that the area of mould infection of TiO2B sample was reached 100% at the end of a month (Fig. 9). Furthermore, the photographs of the bottom surfaces of bamboo samples after two weeks exposure to the outdoor test field are shown in Fig. 8b. We can see that the original bamboo and TiO2B samples were both infected by the mould fungi. Compared with the upper surfaces of TiO2B samples, there are more mould fungi covered on the bottom surfaces of TiO2B samples. The area of mould infection was reached 100% after two weeks. Our previous study have illuminated that TiO2-coated film on bamboo surface in the presence of solar light exhibited weak antifungal capability.39 Though the weather in the test period was cloudy or rainy, a small amount of ultraviolet light could still shine on the surface of bamboo through the clouds. Therefore, the surface of TiO2B sample, which is exposed to solar light, exhibits little higher antifungal activity than the shady face of sample. In contrast, FeTiO2B is different from the original bamboo and TiO2B, the bottom surfaces of FeTiO2B still displayed an excellent antifungal activity without UV light (solar light) (Fig. 8b). The similar experimental results were presented in Fig. 10. The end face and side face of FeTiO2B both remained the high photocatalytic disinfection efficiency of mould fungi than the original bamboo and TiO2B even under the natural environment. This high efficiency of mould fungi disinfection by the Fe-doped TiO2 thin films was a result of the Fe doping, lead to the significantly extending of the optical responses from UV to visible region.
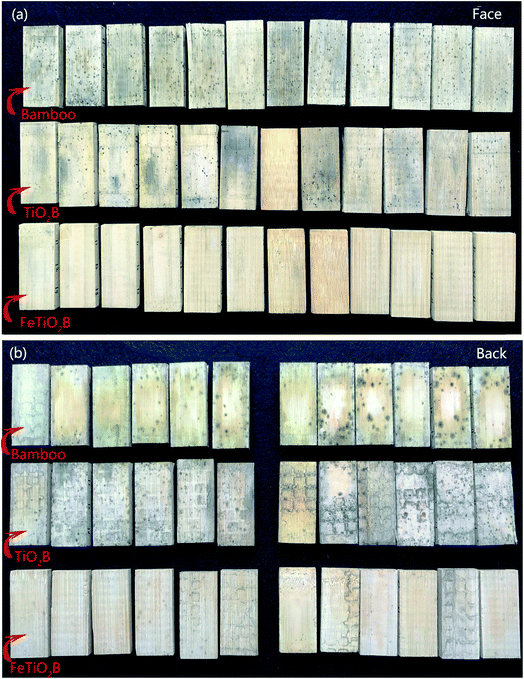 |
| Fig. 8 Digital photograph of the as-prepared samples after two weeks exposure to the outdoor test field. (a) Face to the sun; (b) back to the sun. | |
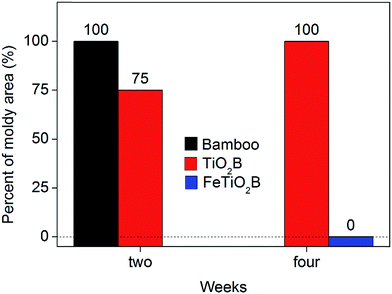 |
| Fig. 9 Antifungal activity of the as-prepared samples after one-month exposure. | |
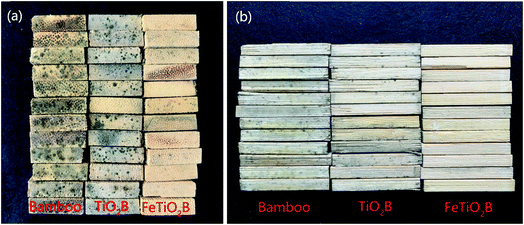 |
| Fig. 10 Digital photograph of the as-prepared samples after two weeks exposure to the outdoor test field. (a) End face; (b) side face. | |
Based on the above investigation of the experimental parameters for TiO2B and FeTiO2B, we concluded that Fe doping can decrease the band gap energy of TiO2, extending the utilization of the visible light. As shown in Fig. 2, even without UV light, the Fe-doped TiO2 still presented higher visible light photocatalytic activity than that of pure TiO2. Fe in FeTiO2B acted as an intermediate agent for the transfer of photogenerated electrons from the valence band to the conduction band of TiO2. Therefore, Fe could contribute to the electron–hole pair separation efficiency and to inhibiting recombination of the electrons and holes, leading to an increasing lifetime of generated electrons. According to the XPS results, Fe defected into the TiO2 lattice produced Ti3+ ions could increase the electron–hole separation capacity of the photocatalyst, which is due to the production of oxygen vacancy (eqn (3)). Additionally, Fe3+ ions came into react with Ti3+ ions (eqn (4)) to promote the proceed of eqn (3) and produce more oxygen vacancy. That is, the presence of oxygen vacancy could reduce the band gap of TiO2, and thus the electron–hole pairs could be easily generated when FeTiO2B was irradiated by even visible light. The photoinduced electron–hole pairs then reacted with water and molecular oxygen absorbed on the photocatalyst surface, and produced hydroxyl radicals (˙OH) and superoxide radical anions (˙O2−) (eqn (5)–(7)).13,40 Moreover, Fe3+ ions would also react with the oxygen species and hydroxyl species adsorbed on the surfaces to form hydroxyl radicals (˙OH) and superoxide radical anions (˙O2−) (eqn (8)–(10)). Finally, these oxygen radicals attacked organic components of mould fungi resulting in its death (eqn (11)).41
|
TiO2 + O2− → 2Ti4+ + 2Ti3+ + 0.5O2
| (3) |
|
Fe3+ + Ti3+ → Ti4+ + Fe2+
| (4) |
|
Fe3+ + O− → Fe4+ + O2−
| (8) |
|
Fe4+ + OH− → Fe3+ + ˙OH (ROS)
| (9) |
|
Fe2+ + O2 → Fe3+ + ˙O2− (ROS)
| (10) |
|
ROS + organic compounds → CO2 + H2O
| (11) |
To further verify the excellent antifungal activity of FeTiO2B caused by visible light, the original bamboo and FeTiO2B were placed in a natural environment without any light. Fig. 11 shows the mould fungi growth on the surfaces of original bamboo and FeTiO2B after being cultured in the dark environment conditions for one week. As shown in Fig. 11, the original bamboo and the FeTiO2B were both infected with mould fungi on the surfaces. Without light illumination, the mould fungi grown on FeTiO2B surfaces, indicating that Fe-doped TiO2 nanoparticles were not toxic to the mould fungi by themselves. The above results demonstrated that Fe-doped TiO2 is crucial for inhibition growth of mould fungi on the surface of bamboo under solar light, which can be more effective in the protection and maintenance of bamboo surfaces than pure TiO2, thus reducing the biodeterioration processes.
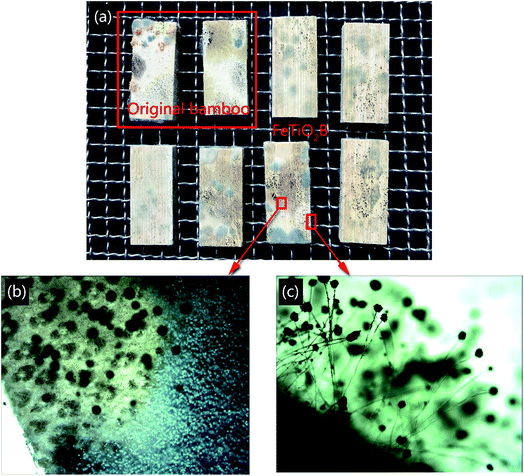 |
| Fig. 11 (a) Digital photograph of original bamboo and FeTiO2B after one-week exposure in dark environments. (b, c) Optical microscope pictures of FeTiO2B sample colonized by mould fungi. | |
4. Conclusions
In summary, visible-light-mediated antifungal bamboo based on Fe-doped TiO2 thin films was successfully fabricated via a facile one-step homogeneous precipitation method. Fe doping exhibits a significant influence on the crystallinity, crystallite size, and photocatalytic activity of the TiO2 products. Fe doped in the TiO2 lattice also shifted the optical absorption edge to the visible region. Compared with TiO2/bamboo, the Fe-doped TiO2/bamboo exhibited much higher photocatalytic disinfection activity to mould fungi under natural environment. Fe dopants increased the electron–hole pair separation efficiency, inhibited their recombination leading to a lifetime increase of the generated electrons, and thus improved antifungal activity even under nature light. Therefore, Fe-doped TiO2 photocatalyst has a great potential as an environmentally-friendly alternative method to inhibit mould fungi under solar irradiation for protecting bamboo products.
Conflicts of interest
There are no conflicts to declare.
Acknowledgements
The work was financially supported by the Fundamental Research Funds for the Central Non-profit Research Institution of CAF (CAFYBB2017MA023) and Key Laboratory of High Efficient Processing of Bamboo of Zhejiang Province (2014F10047).
References
- F. N. Chen, X. D. Yang and Q. Wu, Environ. Sci. Technol., 2009, 43, 4606–4611 CrossRef CAS PubMed.
- W. Wang, G. Li, D. Xia, T. An, H. Zhao and P. K. Wong, Environ. Sci.: Nano, 2017, 4, 782–799 RSC.
- J. Meng, P. Zhang, F. Zhang, H. Liu, J. Fan, X. Liu, G. Yang, L. Jiang and S. Wang, ACS Nano, 2015, 9, 9284–9291 CrossRef CAS PubMed.
- T. Leshuk, R. Parviz, P. Everett, H. Krishnakumar, R. A. Varin and F. Gu, ACS Appl. Mater. Interfaces, 2013, 5, 1892–1895 CAS.
- I. G. Yu, J. K. Yong, H. J. Kim, C. Lee and I. L. Wan, J. Mater. Chem., 2010, 21, 532–538 RSC.
- H. Bodaghi, Y. Mostofi, A. Oromiehie, Z. Zamani, B. Ghanbarzadeh, C. Costa, A. Conte and M. A. D. Nobile, LWT--Food Sci. Technol., 2013, 50, 702–706 CrossRef CAS.
- T. Matsunaga, R. Tomoda, T. Nakajima and H. Wake, FEMS Microbiol. Lett., 1985, 29, 211–214 CrossRef CAS.
- R. Nakano, H. Ishiguro, Y. Yao, J. Kajioka, A. Fujishima, K. Sunada, M. Minoshima, K. Hashimoto and Y. Kubota, Photochem. Photobiol. Sci., 2012, 11, 1293–1298 CAS.
- L. Rizzo, J. Hazard. Mater., 2009, 165, 48–51 CrossRef CAS PubMed.
- F. Chen, X. Yang and Q. Wu, Build. Environ., 2009, 44, 1088–1093 CrossRef.
- C. A. Linkous, G. J. Carter, D. B. Locuson, A. J. Ouellette, D. K. Slattery and L. A. Smitha, Environ. Sci. Technol., 2000, 34, 4754–4758 CrossRef CAS.
- J. Lonnen, S. Kilvington, S. C. Kehoe, F. Altouati and K. G. Mcguigan, Water Res., 2005, 39, 877–883 CrossRef CAS PubMed.
- J. Zhang, Y. Liu, Q. Li, X. Zhang and J. K. Shang, ACS Appl. Mater. Interfaces, 2013, 5, 10953–10959 CAS.
- A. Flynn, K. W. Chan, Z. H. Zhu and L. Yu, J. Rural Stud., 2017, 49, 128–139 CrossRef.
- H. Chi, T. Nguyen, D. Stanley, A. Persily and R. L. Corsi, Build. Environ., 2014, 81, 226–233 CrossRef.
- N. C. Birben, C. S. Uyguner-Demirel, S. S. Kavurmaci, Y. Y. Gürkan, N. Turkten, Z. Cinar and M. Bekbolet, Catal. Today, 2017, 281, 78–84 CrossRef CAS.
- E. Bae, W. Choi, J. Park, H. S. Shin, S. B. Kim and J. S. Lee, J. Phys. Chem. B, 2014, 108, 14093–14101 CrossRef.
- Z. Zhao, W. Zhang, X. Lv, Y. Sun, F. Dong and Y. Zhang, Environ. Sci.: Nano, 2016, 3, 1306–1317 RSC.
- L. Wang, J. Fan, Z. Cao, Y. Zheng, Z. Yao, G. Shao and J. Hu, Chem.–Asian J., 2014, 9, 1904–1912 CrossRef CAS PubMed.
- M. Zhou, J. Yu and B. Cheng, J. Hazard. Mater., 2006, 137, 1838–1847 CrossRef CAS PubMed.
- J. Choi, H. Park and M. R. Hoffmann, J. Phys. Chem. C, 2010, 114, 783–792 CAS.
- A. J. Fonseca, F. Pina, M. F. Macedo, N. Leal, A. Romanowska-Deskins, L. Laiz, A. Gomez-Bolea and C. Saiz-Jimenez, Int. Biodeterior. Biodegrad., 2010, 64, 388–396 CrossRef CAS.
- J. Li, J. Xu, W. L. Dai, H. Li and K. Fan, Appl. Catal., B, 2009, 85, 162–170 CrossRef CAS.
- J. C. Yu, W. Ho, J. Lin, H. Yip and P. K. Wong, Environ. Sci. Technol., 2003, 37, 2296–2301 CrossRef CAS PubMed.
- W. Zhang, Y. Chen, S. Yu, S. Chen and Y. Yin, Thin Solid Films, 2008, 516, 4690–4694 CrossRef CAS.
- M. F. L. Russa, A. Macchia, S. A. Ruffolo, F. D. Leo, M. Barberio, P. Barone, G. M. Crisci and C. Urzì, Int. Biodeterior. Biodegrad., 2014, 96, 87–96 CrossRef.
- C. C. Trapalis, P. Keivanidis, G. Kordas, M. Zaharescu, M. Crisan, A. Szatvanyi and M. Gartner, Thin Solid Films, 2003, 433, 186–190 CrossRef CAS.
- EN927-3 Paints and varnishes, Coating Materials and Coating Systems for Exterior Wood – Part 3 Natural Weathering Test, European Committee for Standardization, 2000 Search PubMed.
- F. Sun, B. Bao, L. Ma, A. Chen and X. Duan, J. Wood Sci., 2012, 58, 51–56 CrossRef CAS.
- J. Lin, Y. Lin, P. Liu, M. J. Meziani, L. F. Allard and Y. Sun, J. Am. Chem. Soc., 2002, 124, 11514–11518 CrossRef CAS PubMed.
- Y. Niu, M. Xing, J. Zhang and B. Tian, Catal. Today, 2013, 201, 159–166 CrossRef CAS.
- M. Xing, Y. Wu, J. Zhang and F. Chen, Nanoscale, 2010, 2, 1233–1239 RSC.
- M. Zhou and J. Yu, J. Hazard. Mater., 2008, 152, 1229–1236 CrossRef CAS PubMed.
- S. Liu, X. Sun, J. G. Li, X. Li, Z. Xiu, H. Yang and X. Xue, Langmuir, 2010, 26, 4546–4553 CrossRef CAS PubMed.
- H. Song, Y. Li, Z. Lou, M. Xiao, L. Hu, Z. Ye and L. Zhu, Appl. Catal., B, 2015, 166–167, 112–120 CrossRef CAS.
- M. Arienzo, R. Scotti, L. Wahba, C. Battocchio, E. Bemporad, A. Nale and F. Morazzoni, Appl. Catal., B, 2009, 93, 149–155 CrossRef.
- J. Ng, S. Xu, X. Zhang, Y. Y. Hui and D. D. Sun, Adv. Funct. Mater., 2011, 20, 4287–4294 CrossRef.
- H. Yu, H. Irie, Y. Shimodaira, Y. Hosogi, Y. Kuroda, M. Miyauchi and K. Hashimoto, J. Phys. Chem. C, 2010, 114, 16481–16487 CAS.
- J. Li, H. Yu, Z. Wu, J. Wang, S. He, J. Ji, N. Li, Y. Bao, C. Huang and Z. Chen, Colloids Surf., A, 2016, 508, 117–123 CrossRef CAS.
- T. Tong, J. Zhang, B. Tian, F. Chen and D. He, J. Hazard. Mater., 2008, 155, 572–579 CrossRef CAS PubMed.
- C. Sichel, M. D. Cara, J. Tello, J. Blanco and P. Fernández-Ibáñez, Appl. Catal., B, 2007, 74, 152–160 CrossRef CAS.
|
This journal is © The Royal Society of Chemistry 2017 |