DOI:
10.1039/C7RA09722K
(Paper)
RSC Adv., 2017,
7, 49858-49862
Investigation of membrane condensation induced by CaCO3 nanoparticles and its effect on membrane protein function†
Received
1st September 2017
, Accepted 17th October 2017
First published on 26th October 2017
Abstract
The membrane dynamics both across the lipid bilayer and in the lateral lipid domains play an important role in a number of key cellular processes including the normal functions of membrane proteins. Herein, we report an electrical method to monitor the changes in membrane dynamics with respect to the interactions between the lipid bilayer and CaCO3 nanoparticles (nano CaCO3). Through transmembrane capacitance measurements, the electrostatic interaction between lipid molecules and Ca2+ ions dissolved from nano CaCO3 turned out to be responsible for the membrane condensation, whereas nano CaCO3 itself didn't show any notable interaction with the lipid bilayer. Moreover, the activities of gramicidin represented by the channel forming rate and lifetime of the channel were shown to be greatly influenced by nano CaCO3. These findings demonstrate that a potential cytotoxicity of nano CaCO3 could be derived from the dissolved Ca2+ ions, but not from nano CaCO3 itself, affecting cellular membrane dynamics by lipid condensation.
Introduction
Calcium is one of the essential minerals that play an important role in bodily functions, including the formation of bones and teeth, muscle contractions, signal transductions, acting as a cofactor for a range of enzymatic reactions, etc. Calcium carbonate (CaCO3) is a naturally occurring calcium salt that is commonly used as a source of calcium supplement.1 Since CaCO3 has low solubility under physiological conditions, many efforts, for example, on the production of CaCO3 in nanoparticulate form (nano CaCO3) are carried out to increase its bioavailability when consumed as a calcium supplement. Due to the great biostability and non-toxic nature of CaCO3, nano CaCO3 has also emerged as promising drug carrier targeting tumour tissues and cells.2 On the other hand, there has been growing concern toward potential health effect of nanoparticles over the past decades,3 and most nanoparticles with the size in the range of 1 to 100 nm have been found to alter signalling processes essential for normal cell functions and, in some cases, lead to cell death.4 Moreover, recent observation in cytotoxicity assay demonstrated that Ca2+ ions from nano CaCO3 could cause membrane damage through oxidative stress.5 However, the mechanism of the interaction between nano CaCO3 and lipid membrane is not fully understood yet.
Planar lipid bilayer (PLB) is a two-dimensional structure presenting a great deal of advantages as a model system mimicking the cell membrane. Upon integration with a proper electrical setup, PLB can be used as a platform for investigating a number of membrane associated ion channels and nanopore based DNA sequencing technique.6,7 In principle, lipid bilayers are a thin insulating membrane that acts as a capacitor when applying a potential bias across the membrane,8 so that the changes of lipid bilayer structure in molecular level can be directly monitored by measuring the membrane capacitance.9 Recently, the interaction between surface-modified gold nanoparticles and PLB was successfully investigated based on this principle, in which the adsorption of the nanoparticles to lipid membrane was quantitatively monitored by monitoring the changes in capacitance of the membranes.10 In addition, gramicidin channel activity can be employed as an excellent reporter to study the effect of membrane-incorporated compound on the lipid bilayer membrane together with prediction of how such a given compound on the lipid bilayer dynamics as well as the membrane protein functions under physiological conditions.11–13 Gramicidin is a cation-selective membrane channel protein whose activities, such as channel forming rate and its lifetime, are closely associated with the status of membrane dynamics.
In this study, we investigated the effect of nano CaCO3 on the dynamics of lipid bilayer by monitoring the modulated capacitance of the lipid membrane. Subsequently, we employed gramicidin as a molecular force transducer to directly probe the mechanical properties of the bilayer membrane in the presence of nano CaCO3, assuming that there would be a correlation between the changes in membrane capacitance and gramicidin-mediated ions transport activity. Finally, we used this platform to evaluate the effect of nano CaCO3 on the membrane dynamics, which would be of importance to elucidate the mechanisms of potential toxicity of nano CaCO3.
Experimental
Chemicals and reagents
Phosphate buffered saline (PBS) was purchased from Noblebio Co. Ltd. (Suwon, Korea). 1,2-Diphytanoyl-sn-glycero-3-phosphocholine (DPhPC) was purchased from Avanti polar lipid (Alabaster, USA). n-Decane was purchased from Fisher Scientific. Bulk calcium carbonate (CaCO3) and nano CaCO3 were provided from Apexel Ltd. (Daejeon, Korea). Calcium chlorides (CaCl2) was purchased from Duchefa biochemie (Haarlem, Netherlands) and sodium hydroxide (NaOH) was purchased from Daejung (Siheung, Korea). Gramicidin, potassium chloride (KCl) and Quantofix-calcium test kit were purchased from Sigma-Aldrich (St. Louis, MO, U.S.A).
Bilayer preparation and electrical measurement
The painting method was employed to prepare a planar lipid bilayer membrane.14 An aperture of diameter around 150 μm was made on 50 μm-thick Teflon film (HANAROTR Corp, Suwon, Korea) using a spark generator (Daedalon, Salem, MA, U.S.A). For lipid stock, DPhPC was dissolved in chloroform and dried in a gentle stream of nitrogen gas to remove the solvent. The lipids were redissolved in n-decane to a final concentration of 3% (wt/v) and stored at −80 °C until use. The lipid/decane stock was prepainted on both sides of the aperture using a 200 μl plastic pipette tip and the Teflon film was placed horizontally between two custom-built Teflon chambers. The setup was then dried in vacuum chamber for 15 minutes to remove the excess solvent before introducing 2 ml of 1× PBS (pH 7.4) solution to both cis and trans chamber. Ag/AgCl electrodes were immersed in the trans and cis chambers to measure the capacitance and current flow across the membrane. The electrical data were collected using the Axon Digidata 1440A data digitizer and Axopatch 200b patch clamp (Molecular Devices, Sunnyvale, CA, U.S.A) at a sampling rate of 100 kHz, and then analyzed using the Clampfit 10.2 software. The output signal was filtered with a low pass Bessel filter at 300 Hz for membrane capacitance and a Gaussian filter at 10 kHz for gramicidin-mediated transmembrane current measurement.
In order to measure the changes in membrane capacitance, we applied a triangle-waveform voltage that was cyclically ramped between −10 and 10 mV. The formation of a lipid bilayer was confirmed by the characteristic signal of a capacitive square waveform. In our system, the membrane with a capacitance value greater than 100 pF was regarded as a bilayer.10 After the capacitance of the lipid bilayer remained constant for 10 min, two types of CaCO3, bulk CaCO3 and nano CaCO3, were introduced to the chamber. The concentrations of CaCO3 stock solution were increased from 0.2 to 5 mg ml−1 with a definitive time interval of 10 min. The transmembrane capacitances were measured three times per every 10 min.
For gramicidin experiment, 1 M KCl (pH 7.4) was used as a supporting electrolyte to measure the current flow through the gramicidin channels formed in lipid bilayer. After the capacitance value of membrane remained constant for 10 min at bias voltage of 200 mV, gramicidin (final conc. 2.7 nM) was introduced to the chamber. Once the first gramicidin-mediated current flow was observed, the solutions in both chambers were replaced with a fresh electrolyte to cease the incorporation of excess gramicidin into the bilayer membrane. To prevent disruption of the bilayer, buffer exchanges should be performed with an extra care by using micropipette. All experiments were performed in a Faraday cage (Warner Instruments) with a floating air table.
Quantification of Ca2+ ions
The amount of Ca2+ ions dissolved from varying concentrations of CaCl2, bulk CaCO3, and nano CaCO3 in 1× PBS solution were measured by using Quantofix-calcium test kit (Sigma) that can quantify the concentration of Ca2+ ions by using the indicator strips. After reaction, the indicator strips were dried under a gentle stream of nitrogen gas. In order to minimize the effect of the undesirable oxidation, the dried indicator strips were immediately transferred to HP scanner device (Officejet Pro 8660) to obtain the image of the strips. ImageJ software was used to convert the color of indicator strips into grayscale value to quantify the concentration of Ca2+ ions.
Results and discussion
The schematic illustration of electrical measurement system is shown in Fig. 1a. In this system, PLB that separated cis and trans chamber was considered as a planar capacitor exhibiting a resistance (R) of approximately 108 ohm cm2.15 The PLB we used to insulate these two chamber is made by diphytanoyl phosphatidylcholine (DPhPC) that has been historically known for its high mechanical stability, and the capacitance of DPhPC-based PLB is about 0.412 μF cm−2.16 When applying a triangular voltage wave, the capacitive current across the bilayer is given by: |
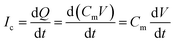 | (1) |
where Ic is the current across the capacitor, Q is the charge on the surface of this capacitor, V is the applied potential, Cm is the membrane capacitance (F). If the specific Cm and thickness of PLB is known, the surface area of the bilayer can be calculated with |
 | (2) |
where ε0 is the absolute dielectric constant, 8.854 × 10−12 (F m−1), and εm is the relative dielectric constant of the membrane, which is taken to be close to 2.2 for DPhPC.17 Here the medium is the mixture of the hydrophobic core of PLB and the solvent, decane. A is the surface area of the PLB (m2), and d is the thickness of the dielectric hydrophobic core of the PLB, which is 4.5 nm for DPhPC.16 When applying a triangular voltage wave (±10 mV) across the PLB, we observed the square-wave form current which is a measure of bilayer capacity (Fig. 1a). As each lipid molecule has an ability to hold a certain amount of charge, the capacitance can be considered as a parameter showing structural changes of lipid molecule assuming that the area of PLB are constant.10 Accordingly, we speculate that the changes in capacitance of the lipid membrane can be utilized as a measure to predict the effect of nano CaCO3 on the density of lipid molecules in the lipid membrane (Fig. 1b).
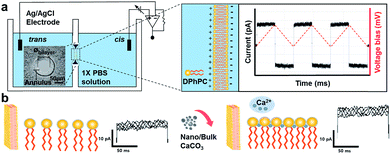 |
| Fig. 1 Schematic illustration showing electrical setup for the measurement of membrane capacitance. The insert shows the diameter of planar lipid bilayer (øbilayer) prepared on the Teflon aperture. (a) A lipid bilayer separating cis and trans chambers filled with 1× PBS (pH 7.4) was constructed on Teflon film. The magnitude of the square-wave current represents the membrane capacitance at an applied triangular wave voltage of ±10 mV (dotted red line). (b) Electrostatic interaction between lipid head and Ca2+ ions after addition of nano or bulk CaCO3 to the chamber and the subsequent changes in membrane capacitance. | |
In this study, we investigated the effect of two types of calcium carbonate particles, nano CaCO3 in a size of about 100 nm and bulk CaCO3 of size about 1–3 μm (Fig. 2a), on the dynamics of PLB. Here the bulk CaCO3 is used as a control to determine the size effect of nano CaCO3 in terms of its effect on membrane capacitance. In CaCO3-free condition, the PLB exhibited a structural stability with a constant capacity more than 10 min at the given potential. On the other hand, nano CaCO3 caused notable changes in membrane capacitance, which is much greater than those of bulk CaCO3 (Fig. 2b), indicating the increased lipid density of the bilayer in the presence of nano CaCO3. Nano CaCO3 did not show pore forming activity or adsorption to the membrane according to the very stable current trace over the course of tests. Thus, we propose that changes of membrane capacitance were due primarily to the interactions between divalent calcium ions dissolved from nano CaCO3 and the phosphate group in lipid head group (Fig. 1b). Calcium ions have been known to cluster phospholipid molecules via ion-bridge,18 so that lipid molecules are attracted to each other, resulting in the increase of lipid density of the membrane.19,20 Moreover, there was a gradual increase in the membrane capacitance upon increasing the concentration of nano CaCO3 ranging from 0.2 mg ml−1 to 5 mg ml−1, while bulk CaCO3 showed a negligible change in membrane capacitance regardless of its concentration. This might be due to the higher solubility of nano CaCO3, which agrees well with grayscale value analysis for the dissolved calcium ions from nano CaCO3 and bulk CaCO3 (ESI Fig. S1†). On the other hand, we found that the addition of nano CaCO3 caused a slight increase of pH compared with that of the bulk CaCO3 (Fig. 3a). The effects of pH on the membrane capacitance were therefore taken into account in this study. We examined the effect of pH on membrane capacitance, and found that the membrane capacitances increased slightly in respond to the increase of pH values (Fig. 3b). This might be due to the increase in the concentration of free OH−, some of which can enhance the negativity of polar head groups of lipid molecule, leading to the increases in the membrane capacitance.21 However, the change of capacitance was insignificant (<0.5%) upon increasing the concentration of NaOH up to 0.4 μM, which brought about the pH value corresponding to that obtained from the highest concentration of nano CaCO3 we tested (Fig. 3a). In addition, we observed a concentration-dependent increase in the capacitance when the lipid bilayer was exposed to CaCl2 that even lowered the pH value of the solution (ESI Fig. S2†). These results demonstrate that the cause of the capacitance change is more likely due to the increased lipid density of the membrane by the cation–lipid interactions rather than the increased pH in the electrolyte solution.
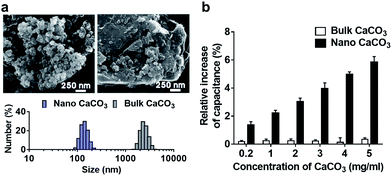 |
| Fig. 2 (a) Scanning electron microscope image of nano CaCO3 and bulk CaCO3. Histogram of the size distribution corresponding to nano CaCO3 and bulk CaCO3 were shown below. (b) Relative increase of membrane capacitance in response to the varying concentration of nano CaCO3 and bulk CaCO3. | |
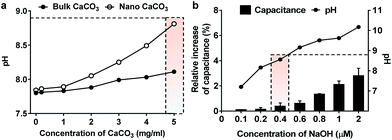 |
| Fig. 3 pH effect on membrane dynamics. (a) pH of the electrolyte solution in response to the addition of varying concentration of nano CaCO3 and bulk CaCO3. (b) Relative increase of membrane capacitance as a function of NaOH concentration. The pH of electrolyte solution with a given concentration of NaOH was plotted in solid line. The capacitance change induced by the pH corresponding to that obtained from the highest concentration (5 mg ml−1) of nano CaCO3 was indicated by dotted box. | |
Subsequently, the gramicidin-mediated ions transport experiments were carried out to evaluate the effect of nano CaCO3 on mechanical properties of the lipid bilayer of which its experimental setup is shown in Fig. 4a. Considering that gramicidin can spontaneously incorporate into the membrane, a given concentration of gramicidin (2.7 nM) was added to the electrolyte solutions in both cis and trans chambers. Upon observation of the occurrence of the first leakage conductance, indicating the formation of gramicidin channel in lipid bilayer, the electrolyte solutions were replaced with fresh one without gramicidin to cease excessive incorporation of gramicidin to the membrane. In this study, the channel forming rates of gramicidin, however, was not considered as a parameter representing the effect of nano CaCO3 on the channel function due to the uncontrollable movement of gramicidin from the solvent annulus to the bilayer.12 Instead, the lifetime of gramicidin channels was only taken into account to analyze the changes of the channel behavior in the presence of nano CaCO3. In control experiment, without nano CaCO3, gramicidin channels showed a wide range of lifetime distribution (Fig. 4b). Interestingly, the channel lifetimes were notably decreased as a function of the concentration of nano CaCO3, indicating that the membrane condensation provided a driving force for the channel dissociation. These results indicate that membrane condensation induced by Ca2+ ions that were dissolved from nano CaCO3 could alter the dynamics of membrane protein, gramicidin, by shortening the lifetime of the channels, in agreement with that observed from the DOPS bilayers.22 It might be due to Ca2+-mediated changes in the electrostatic interaction among lipid head groups,23 which caused lipid packing and also affected the bending elasticity of bilayer.24 From the results, we assume that the changes in mechanical and electrical properties of the membranes could influence the monolayer equilibrium curvature, leading to a change in the curvature stress in the bilayer,25 consequently increasing the disjoining force (Fdis) that influence the functions of gramicidin channels (Fig. 4c).26
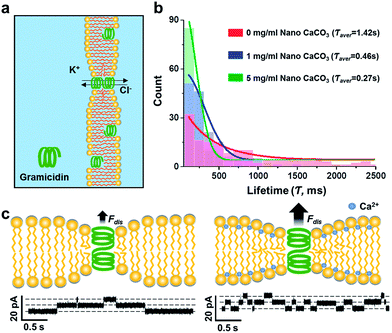 |
| Fig. 4 Channel forming activities of gramicidin upon varying concentration of nano CaCO3. (a) Experimental setup. Gramicidin was introduced to both trans and cis chambers. (b) Histogram of lifetime distribution of gramicidin channel depending on the concentration of nano CaCO3. The average lifetime (Τaver) of gramicidin channel at different concentration of nano CaCO3 were indicated in the top-right corner. (c) Effect of Ca2+ on curvature stress of lipid bilayer, altering the channel function of gramicidin. Single-channel conductance traces in the absence (left) and presence (right) of nano CaCO3. | |
Conclusions
Although nano CaCO3 did not exhibit any notable physical effect on model cell membrane, the divalent calcium ions dissolved from nano CaCO3 were shown to induce the condensation of lipid membrane, which was reflected by the increased membrane capacitance in concentration dependent manner. Comparing to bulk CaCO3, nano CaCO3 showed significantly higher condensation effect on lipid membrane mainly due to its higher solubility in aqueous environment. The changes in the density and bending elasticity of lipid membrane by nano CaCO3 also altered the channel function of gramicidin embedded in lipid bilayer, implying that the excess divalent calcium ions dissolved from nano CaCO3 may pose cytotoxic effect on cell membrane through altering the nature of membrane dynamics. We believe that the developed platform will be of powerful tool to study membrane dynamics in association with potential cytotoxic components in molecular level.
Conflicts of interest
The author declares no conflicts of interest.
Acknowledgements
This research was supported by the Pioneer Research Center Program through the National Research Foundation of Korea funded by the Ministry of Science, ICT & Future Planning (2012-0009575 & 2012-0009563), and the National Research Foundation of Korea (NRF-2012M3A7B4049864).
Notes and references
- I. R. Reid, R. W. Ames, M. C. Evans, G. D. Gamble and S. J. Sharpe, N. Engl. J. Med., 1993, 328, 460–464 CrossRef CAS PubMed.
- S. Maleki Dizaj, M. Barzegar-Jalali, M. H. Zarrintan, K. Adibkia and F. Lotfipour, Expert Opin. Drug Delivery, 2015, 12, 1649–1660 CrossRef PubMed.
- A. Elsaesser and C. V. Howard, Adv. Drug Delivery Rev., 2012, 64, 129–137 CrossRef CAS PubMed.
- S. R. Datta, A. Brunet and M. E. Greenberg, Genes Dev., 1999, 13, 2905–2927 CrossRef CAS PubMed.
- M.-K. Kim, J.-A. Lee, M.-R. Jo, M.-K. Kim, H.-M. Kim, J.-M. Oh, N. W. Song and S.-J. Choi, Nanomaterials, 2015, 5, 1938–1954 CrossRef CAS PubMed.
- G. Maglia, M. R. Restrepo, E. Mikhailova and H. Bayley, Proc. Natl. Acad. Sci. U. S. A., 2008, 105, 19720–19725 CrossRef CAS PubMed.
- S. Ladha, A. Mackie, L. Harvey, D. Clark, E. Lea, M. Brullemans and H. Duclohier, Biophys. J., 1996, 71, 1364 CrossRef CAS PubMed.
- W. Hanke and W. Schulue, Planar Lipid Bilayers: Methods and Applications, Academic Press, 2012 Search PubMed.
- L. C. Gross, A. J. Heron, S. C. Baca and M. I. Wallace, Langmuir, 2011, 27, 14335–14342 CrossRef CAS PubMed.
- R. P. Carney, Y. Astier, T. M. Carney, K. Voïtchovsky, P. H. Jacob Silva and F. Stellacci, ACS Nano, 2013, 7, 932–942 CrossRef CAS PubMed.
- O. S. Andersen and R. E. Koeppe, Annu. Rev. Biophys. Biomol. Struct., 2007, 36, 107–130 CrossRef CAS PubMed.
- H. Ryu, H. Lee, S. Iwata, S. Choi, M. K. Kim, Y.-R. Kim, S. Maruta, S. M. Kim and T.-J. Jeon, Sci. Rep., 2015, 5, 11935 CrossRef PubMed.
- J. A. Lundbaek and O. S. Andersen, J. Gen. Physiol., 1994, 104, 645–673 CrossRef CAS PubMed.
- P. Mueller, D. O. Rudin, H. Ti Tien and W. C. Wescott, Nature, 1962, 194, 979–980 CrossRef CAS PubMed.
- G. Wiegand, N. Arribas-Layton, H. Hillebrandt, E. Sackmann and P. Wagner, J. Phys. Chem. B, 2002, 106, 4245–4254 CrossRef CAS.
- J. Stern, H.-J. Freisleben, S. Janku and K. Ring, Biochim. Biophys. Acta, Lipids Lipid Metab., 1992, 1128, 227–236 CrossRef CAS.
- G. Valincius, F. Heinrich, R. Budvytyte, D. J. Vanderah, D. J. McGillivray, Y. Sokolov, J. E. Hall and M. Lösche, Biophys. J., 2008, 95, 4845–4861 CrossRef CAS PubMed.
- J. A. Lundbaek, S. A. Collingwood, H. I. Ingolfsson, R. Kapoor and O. S. Andersen, J. R. Soc., Interface, 2010, 7, 373–395 CrossRef CAS PubMed.
- A. A. Gurtovenko and I. Vattulainen, J. Phys. Chem. B, 2008, 112, 1953–1962 CrossRef CAS PubMed.
- R. Kagawa, Y. Hirano, M. Taiji, K. Yasuoka and M. Yasui, J. Membr. Sci., 2013, 435, 130–136 CrossRef CAS.
- S. Ohki, Biophys. J., 1969, 9, 1195 CrossRef CAS PubMed.
- J. A. Lundbæk, A. M. Maer and O. S. Andersen, Biochemistry, 1997, 36, 5695–5701 CrossRef PubMed.
- H. I. Petrache, T. Zemb, L. Belloni and V. A. Parsegian, Proc. Natl. Acad. Sci. U. S. A., 2006, 103, 7982–7987 CrossRef CAS PubMed.
- Y. Zhou and R. M. Raphael, Biophys. J., 2007, 92, 2451–2462 CrossRef CAS PubMed.
- G. L. Kirk, S. M. Gruner and D. Stein, Biochemistry, 1984, 23, 1093–1102 CrossRef CAS.
- J. A. Lundbæk, S. A. Collingwood, H. I. Ingólfsson, R. Kapoor and O. S. Andersen, J. R. Soc., Interface, 2010, 7, 373–395 CrossRef PubMed.
Footnotes |
† Electronic supplementary information (ESI) available: Calcium ions analysis, effect of CaCl2 on membrane capacitance details. See DOI: 10.1039/c7ra09722k |
‡ These authors contributed equally to this work. |
|
This journal is © The Royal Society of Chemistry 2017 |