DOI:
10.1039/C7RA09226A
(Paper)
RSC Adv., 2017,
7, 47143-47147
Rapid and label-free strategy for the sensitive detection of Hg2+ based on target-triggered exponential strand displacement amplification†
Received
21st August 2017
, Accepted 29th September 2017
First published on 6th October 2017
Abstract
We herein describe a rapid and label-free strategy for the sensitive detection of Hg2+ based on target-triggered exponential strand displacement amplification (eSDA). The developed strategy utilizes specially designed DNA probes that form mismatched thymine–thymine (T–T) base pairs at their 3′-ends. In the absence of Hg2+, the mismatched T–T base pairs prohibit the DNA polymerase-mediated extension and the subsequent eSDA does not occur. On the other hand, the presence of Hg2+ that mediates the formation of stable T–Hg2+–T base pairs, enables the extension of DNA probes by DNA polymerase. As a result, a large number of duplexes are produced through the eSDA, which is simply monitored in real-time by the gradual fluorescence enhancement from SYBR green I. By employing this strategy, we detected Hg2+ down to 2.95 pM within 30 min, and the practical applicability of this method was successfully demonstrated by detecting Hg2+ in tap water.
Introduction
As one of the most dangerous heavy metal ions, Hg2+ has been a serious threat to human health because it can be accumulated in human bodies and lead to damage in the brain and the nervous, kidney, and endocrine systems.1,2 To prevent its deleterious effects, the accurate detection of Hg2+ has long been required and numerous methods have been developed based on the specific interaction between Hg2+ and a mismatched thymine–thymine (T–T) base pair.3 The examples of this type are found in different systems which rely on electrochemistry,4,5 colorimetry,6–8 and fluorometry.9–19
Notably, the fluorometric methods which operate in a reliable and simple manner, have been extensively investigated. For instance, Deng et al. utilized fluorescent silver nanoclusters for the detection of Hg2+ and Qi et al. developed a fluorescent method for Hg2+ based on the target-induced activation of deoxyribozyme activity.11,12 In addition, several sensing strategies were also made in combination with isothermal signal amplification methods including HCR, hyperbranched RCA, and EXPAR.15,16,19 Although promising, they not only exhibit the poor sensitivity, but also require the labeling with fluorophore and quencher, preparation and functionalization of nanomaterials, and long detection time, hampering their practical use (Table S1†). Therefore, it is highly necessary to develop a new detection strategy for Hg2+ that overcomes the drawbacks of the previous systems including high-cost, poor sensitivity, and complicated and long procedures.
Towards this goal, we herein developed a rapid and label-free strategy for the sensitive detection of Hg2+ based on target-triggered exponential strand displacement amplification (eSDA), an isothermal nucleic acid amplification method that rapidly produces a large number of duplexes through the cooperative actions of DNA polymerase and nicking endonuclease.20–22 In principle, DNA probes that form the mismatched T–T base pairs at their 3′-ends, are stabilized by the presence of Hg2+ through the formation of stable T–Hg2+–T base pairs, which enables the extension of DNA probes by DNA polymerase. As a result, eSDA is initiated to produce a large number of duplex products, which is simply monitored in real-time by the gradual fluorescence enhancement from a double-stranded (ds) DNA specific fluorescent dye, SYBR green I. Based on this novel strategy, Hg2+ was rapidly analyzed with the high sensitivity and selectivity and its practical applicability was demonstrated by accurately detecting Hg2+ present in tap water.
Experimental
Materials
All DNA strands used in the study were synthesized by Genotech Co. (Daejeon, South Korea) and purified by desalting, except for TP (purified by PAGE). The sequences of all DNAs are listed in Table S2.† Klenow fragment (3′ → 5′ exo-) (KF) and Nt.AlwI were purchased from New England Biolabs Inc. (Beverly, MA, USA). The metal salts and SYBR green I were purchased from Sigma-Aldrich (St. Louis, MO, USA). All other chemicals were of analytical grade and used without further purification. Ultrapure DNase/RNase-free distilled water (DW) purchased from Bioneer® (Daejeon, Korea) was used in all the experiments.
Hg2+ detection procedure
28.5 μL of DW, 2 μL of TP (2.5 μM), 2 μL of FP-T12 (2.5 μM), 2 μL of RP (2.5 μM), 4 μL of dNTP (2.5 mM), 2.5 μL of SYBR green I (20×), 1 μL of KF (5 U μL−1), 1 μL of Nt.AlwI (10 U μL−1), 2 μL of Hg(NO3)2 at varying concentrations and 5 μL of NEBuffer 4 (20 mM Tris-acetate, 50 mM potassium acetate, 10 mM magnesium acetate, 1 mM DTT, pH 7.9 at 1× concentration) were mixed to prepare the reaction solutions of 50 μL and their fluorescence was measured in real-time at every 30 s at 42.5 °C during eSDA on C1000™ thermal cycler (Bio-Rad, CA, USA).23
Detection of Hg2+ in tap water (4%)
The tap water was first confirmed to be free of Hg2+ with the standard method, inductively coupled plasma-mass spectrometry (ICP-MS) (Fig. S1†). Then, Hg2+ at varying concentrations was spiked into the tap water, which was subsequently analyzed using the same procedure to detect Hg2+ as described above.
Results and discussion
The basic principle of the new strategy to detect Hg2+ is illustrated in Fig. 1, which relies on target-triggered eSDA. The developed strategy utilizes the specially designed DNA probes composed of template, forward primer and reverse primer. Forward primer (FP) and template (TP) are designed to form the mismatched T–T base pairs at their 3′-ends. In addition, FP and reverse primer (RP) bear the sequence that contains a single-stranded (ss) recognition site for nicking endonuclease (Nt.AlwI) at their 5′-ends. In the absence of Hg2+, FP, TP, and RP coexist separately due to the mismatched T–T base pairs and the subsequent eSDA does not take place. On the contrary, the presence of Hg2+ enables FP and TP to hybridize at their 3′-ends through the formation of stable T–Hg2+–T base pairs, which promotes the extension from their 3′-ends by DNA polymerase (Klenow fragment (KF)) so that the double-stranded (ds) recognition site for nicking endonuclease is formed (Fig. S2†).16,24 Thus, the nicking endonuclease cleaves FP at the fourth base downstream from the recognition site and generates a new initiation site for the next round of extension, from which ss extension products (TP1) are displaced by DNA polymerase. This cycle termed SDA I consisting of cleavage, extension, and displacement continuously produces a lot of ssDNAs (TP1). Importantly, Hg2+ is released in the extension accompanying the displacement during SDA I and recycled to promote another cycle of SDA I.19,25 In addition, TP1 generated in SDA I, contains the sequence complementary to the 3′-end of RP and thus forms the hybridization complex with RP, which is extended by DNA polymerase from its 3′-ends to generate the ds recognition site for nicking endonuclease. In the same manner to SDA I, a large number of ss extension products (TP2) containing T bases are produced through SDA II, which induces the formation of the hybridization complex between TP2 and FP and promotes SDA I to produce TP3 continuously. It is fed back to SDA II through which a large number of TP2s are generated again. Likewise, SDA I and II are cooperatively repeated to promote the exponential amplification (eSDA) generating a large number of duplex products, which is simply monitored in real-time by the gradual fluorescence enhancement from SYBR green I.
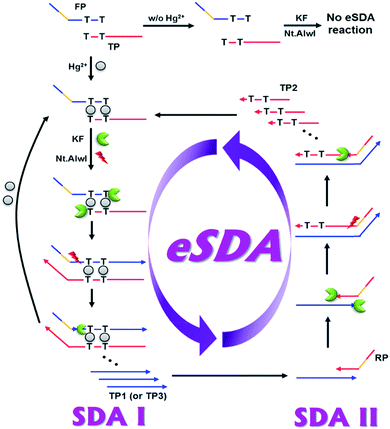 |
| Fig. 1 Schematic illustration of the Hg2+ detection method based on target-triggered eSDA. TP and TP1 are only involved in the first cycle of SDA I and SDA II, respectively. The orange color in FP and RP indicates the single-stranded recognition sequence for nicking endonuclease (Nt.AlwI) and the arrow shows the 3′-end of all DNA probes. | |
Considering that the number of mismatched T–T base pairs, reaction temperature, and concentration of primers would have significant effects on the detection of Hg2+, these factors were first optimized by examining the time-dependent fluorescence enhancement from SYBR green I. The results of experiments in which the number of mismatched T–T base pairs, reaction temperature, and concentration of primers were varied, showed that 12 of mismatched T–T base pairs, 42.5 °C of reaction temperature, and 100 nM of primers were ideal to achieve the best detection performance. Thus, these optimal conditions were used for further experiments (Fig. 2). It should be noted that the nonspecific background signal was inevitably produced in the absence of Hg2+, which might be due to the ab initio DNA synthesis, the template DNA-independent polymerization of free nucleotides by DNA polymerase.26,27
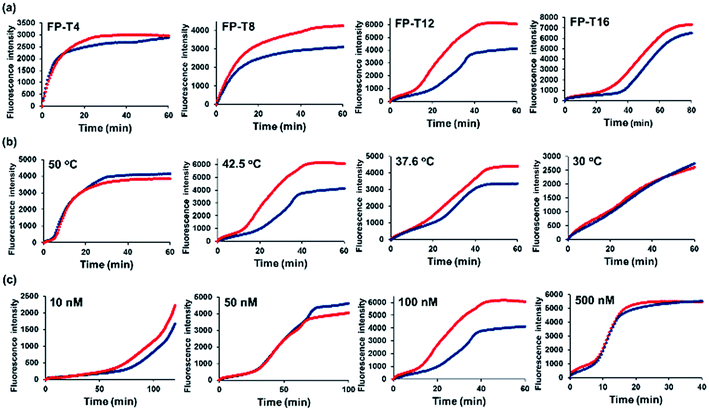 |
| Fig. 2 Optimization of the reaction conditions for the Hg2+ detection method. Fluorescence intensities from SYBR green I are plotted as a function of time during target-triggered eSDA in the absence (blue) and presence (red) of Hg2+ (10 nM). The corresponding reaction conditions are indicated at the left upper corner of each graph. (a) Optimization of the number of mismatched T–T base pairs (Table S2†). The reaction temperature is 42.5 °C and the concentration of primers is 100 nM. (b) Optimization of the reaction temperature. The number of mismatched T–T base pairs is 12 and the concentration of primers is 100 nM. (c) Optimization of the concentration of primers. The number of mismatched T–T base pairs is 12 and the reaction temperature is 42.5 °C. | |
Under the optimal conditions, we measured the time-dependent fluorescence enhancement from SYBR green I at varying concentrations of Hg2+ (Fig. 3(a)). For the accurate quantification of Hg2+, threshold time (Tt), the time to reach the threshold fluorescence intensity, was defined. The results in Fig. 3(a) showed that Tt decreased with increasing concentrations of Hg2+, and the entire assay was completed less than 30 min. In addition, the plot of Tt vs. logarithm of Hg2+ concentration showed that an excellent linear relationship (Tt = −3.7044 × log10(CHg2+/pM) + 31.631, R2 = 0.9911) existed in the range of 10 pM to 10 nM and the limit of detection (LOD) was calculated to be 2.95 pM based on 3 σ/S, where σ is the standard deviation (SD) of blank and S is the slope of the linear relationship (Fig. 3(b)).28,29 This LOD value is lower than those from the previous reports to detect Hg2+ (Table S1†).11–16,18 The relative standard deviations (RSDs) were also calculated at varying concentrations of Hg2+. The results in Table S3† showed that they were less than 10%, proving the good reproducibility of this method.30 These results indicate that target-triggered eSDA with the efficient amplification ability could be exploited to sensitively and reliably analyze Hg2+.
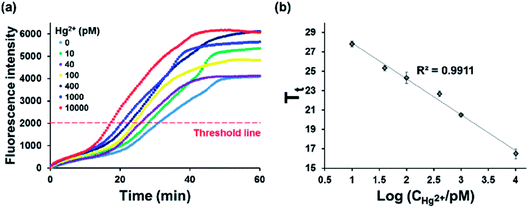 |
| Fig. 3 Sensitivity of the Hg2+ detection method. (a) Fluorescence intensities from SYBR green I are plotted as a function of time during target-triggered eSDA in the presence of varying concentrations of Hg2+. The threshold fluorescence intensity was set for 2000. (b) Linear relationship between Tt and logarithm of Hg2+ concentration (10 pM to 10 nM). | |
To evaluate the specificity of the present strategy towards Hg2+, the abilities of other metal ions (Ca2+, Fe3+, Co2+, Ni2+, Cu2+, Zn2+, Ag+, Cd2+, and Pb2+) to induce the decrease of Tt, were examined and compared with that from Hg2+. As shown in Fig. 4, the significant decrease of Tt was observed in the presence of Hg2+ while the effects of other metal ions on Tt were negligible even though they were applied at a hundred times higher concentration than that of Hg2+. These results clearly demonstrate that this system is highly selective to Hg2+, indicating that target-triggered eSDA is exclusively initiated by the formation of specific and stable T–Hg2+–T base pairs.
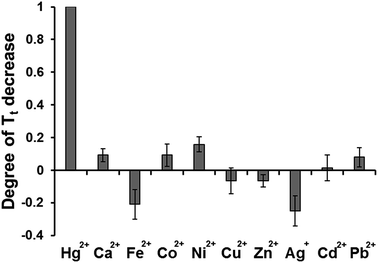 |
| Fig. 4 Specificity of the Hg2+ detection method. Degrees of Tt decrease in the presence of Hg2+ (10 nM) and different metal ions (1 μM). Degree of Tt decrease is defined as (Tt,blank − Tt,M)/(Tt,blank − Tt,Hg2+), where Tt,blank, Tt,M, and Tt,Hg2+ are the threshold times in the presence of blank, metal ions, and Hg2+, respectively. | |
Finally, the practical applicability of the proposed method was verified by determining Hg2+ spiked in tap water. The results in Fig. S3(a)† confirmed that Tt value obtained from the tap water was the same with that from the buffer solution, indicating that the developed system is insensitive to interfering substances present in the tap water. Therefore, the concentrations of Hg2+ were determined based on the standard curve established by the detection of Hg2+ in the buffer solution (Fig. 3(b)). As presented in Table 1 and Fig. S3(b),† Hg2+ concentrations were successfully determined with the excellent precision, as evidenced by the recovery rates (89.3% and 104.1%). These results clearly confirm that the developed strategy can be used to determine Hg2+ in real samples.
Table 1 Determination of Hg2+ in the tap watera
Added Hg2+ (nM) |
Measured Hg2+b (nM) |
Recoveryc (%) |
The concentrations of Hg2+ were determined based on the standard curve established by the detection of Hg2+ in the buffer solution (Fig. 3(b)). Based on this calibration curve, Tt values from unknown samples were used to determine the concentration of Hg2+ in the tap water. Mean of three measurements. Measured value/added value × 100. |
0 |
Not detected |
— |
0.5 |
0.45 |
89.3 |
5 |
5.21 |
104.1 |
Conclusions
In summary, we developed a rapid and label-free method for the sensitive detection of Hg2+ based on target-triggered eSDA. By taking advantage of the efficient amplification ability of eSDA and real-time signal acquisition, the present strategy could detect sHg2+ down to 2.95 pM within 30 min, which outperforms the previous fluorometric methods. In addition, its practical applicability was successfully demonstrated by its use to determine Hg2+ in the tap water. Importantly, this strategy does not require the labeling with fluorophore and quencher and preparation and functionalization of nanomaterials, eliminating the drawbacks of previous fluorometric systems including complexity and high-cost. To the best of our knowledge, this is the first report to exploit the eSDA for the sensitive determination of metal ions, which would broaden the application scope of eSDA as a novel analytical tool.
Conflicts of interest
There are no conflicts to declare.
Acknowledgements
This work was supported by the Mid-career Researcher Support Program through the National Research Foundation (NRF) funded by the Ministry of Science, ICT & Future planning (MSIP) of Korea (No. 2015R1A2A1A01005393) and by the Center for BioNano Health-Guard funded by the MSIP of Korea as Global Frontier Project (Grant H-GUARD_2013M3A6B2078964). Financial support was also provided by the NRF grant funded by the MSIP of Korea (No. 2017R1C1B5017724).
Notes and references
- P. B. Tchounwou, W. K. Ayensu, N. Ninashvili and D. Sutton, Environ. Toxicol., 2003, 18, 149–175 CrossRef CAS PubMed.
- F. Zahir, S. J. Rizwi, S. K. Haq and R. H. Khan, Environ. Toxicol. Pharmacol., 2005, 20, 351–360 CrossRef CAS PubMed.
- Y. Miyake, H. Togashi, M. Tashiro, H. Yamaguchi, S. Oda, M. Kudo, Y. Tanaka, Y. Kondo, R. Sawa and T. Fujimoto, J. Am. Chem. Soc., 2006, 128, 2172–2173 CrossRef CAS PubMed.
- C. Tortolini, P. Bollella, M. L. Antonelli, R. Antiochia, F. Mazzei and G. Favero, Biosens. Bioelectron., 2015, 67, 524–531 CrossRef CAS PubMed.
- G. Wang, H. Huang, X. Zhang and L. Wang, Biosens. Bioelectron., 2012, 35, 108–114 CrossRef CAS PubMed.
- Q. Zhang, Y. Cai, H. Li, D.-M. Kong and H.-X. Shen, Biosens. Bioelectron., 2012, 38, 331–336 CrossRef CAS PubMed.
- W. Ren, Y. Zhang, W. T. Huang, N. B. Li and H. Q. Luo, Biosens. Bioelectron., 2015, 68, 266–271 CrossRef CAS PubMed.
- S. Liu, X. Leng, X. Wang, Q. Pei, X. Cui, Y. Wang and J. Huang, Microchim. Acta, 2017, 184, 1969–1976 CrossRef CAS.
- J. Ge, Y.-H. Du, J.-J. Chen, L. Zhang, D.-M. Bai, D.-Y. Ji, Y.-L. Hu and Z.-H. Li, Sens. Actuators, B, 2017, 249, 189–194 CrossRef CAS.
- J. Ge, X.-P. Li, J.-H. Jiang and R.-Q. Yu, Talanta, 2014, 122, 85–90 CrossRef CAS PubMed.
- L. Deng, Z. Zhou, J. Li, T. Li and S. Dong, Chem. Commun., 2011, 47, 11065–11067 RSC.
- L. Qi, Y. Zhao, H. Yuan, K. Bai, Y. Zhao, F. Chen, Y. Dong and Y. Wu, Analyst, 2012, 137, 2799–2805 RSC.
- D. Huang, C. Niu, X. Wang, X. Lv and G. Zeng, Anal. Chem., 2013, 85, 1164–1170 CrossRef CAS PubMed.
- G. Wang, G. Xu, Y. Zhu and X. Zhang, Chem. Commun., 2014, 50, 747–750 RSC.
- J. Huang, X. Gao, J. Jia, J.-K. Kim and Z. Li, Anal. Chem., 2014, 86, 3209–3215 CrossRef CAS PubMed.
- H. Jia, Z. Wang, C. Wang, L. Chang and Z. Li, RSC Adv., 2014, 4, 9439–9444 RSC.
- G. Zhu, Y. Li and C.-y. Zhang, Chem. Commun., 2014, 50, 572–574 RSC.
- X. Zhu, X. Zhou and D. Xing, Biosens. Bioelectron., 2011, 26, 2666–2669 CrossRef CAS PubMed.
- J. Chen, P. Tong, Y. Lin, W. Lu, Y. He, M. Lu, L. Zhang and G. Chen, Analyst, 2015, 140, 907–911 RSC.
- G. T. Walker, M. S. Fraiser, J. L. Schram, M. C. Little, J. G. Nadeau and D. P. Malinowski, Nucleic Acids Res., 1992, 20, 1691–1696 CrossRef CAS PubMed.
- C. Shi, Q. Liu, M. Zhou, H. Zhao, T. Yang and C. Ma, Sens. Actuators, B, 2016, 222, 221–225 CrossRef CAS.
- C. Shi, Q. Liu, C. Ma and W. Zhong, Anal. Chem., 2013, 86, 336–339 CrossRef PubMed.
- K. S. Park, C. Y. Lee and H. G. Park, Chem. Commun., 2015, 51, 9942–9945 RSC.
- K. S. Park, C. Jung and H. G. Park, Angew. Chem., Int. Ed., 2010, 49, 9757–9760 CrossRef CAS PubMed.
- X. Zhou, Q. Su and D. Xing, Anal. Chim. Acta, 2012, 713, 45–49 CrossRef CAS PubMed.
- N. V. Zyrina, V. N. Antipova and L. A. Zheleznaya, FEMS Microbiol. Lett., 2014, 351, 1–6 CrossRef CAS.
- E. Tan, B. Erwin, S. Dames, T. Ferguson, M. Buechel, B. Irvine, K. Voelkerding and A. Niemz, Biochemistry, 2008, 47, 9987–9999 CrossRef CAS PubMed.
- Y. L. Huang, Z. F. Gao, H. Q. Luo and N. B. Li, Sens. Actuators, B, 2017, 238, 1017–1023 CrossRef CAS.
- K. Wang, J. Liao, X. Yang, M. Zhao, M. Chen, W. Yao, W. Tan and X. Lan, Biosens. Bioelectron., 2015, 63, 172–177 CrossRef CAS PubMed.
- M. Mei, X. Huang, K. Liao and D. Yuan, Anal. Chim. Acta, 2015, 860, 29–36 CrossRef CAS PubMed.
Footnote |
† Electronic supplementary information (ESI) available. See DOI: 10.1039/c7ra09226a |
|
This journal is © The Royal Society of Chemistry 2017 |
Click here to see how this site uses Cookies. View our privacy policy here.