DOI:
10.1039/C7RA09154K
(Paper)
RSC Adv., 2017,
7, 45022-45028
Facile, simple, and inexpensive ionic liquid, 1-phenyl-3-methylimidazole diphenyl phosphate, as an efficient phosphorus-based ligand for copper-catalyzed reverse atom transfer radical polymerization of methyl methacrylate
Received
18th August 2017
, Accepted 14th September 2017
First published on 21st September 2017
Abstract
Ligands play a vital role in atom transfer radical polymerization (ATRP) in solubilizing the transition-metal salt and adjusting the redox potential of the metal center. In general, nitrogen ligands work particularly well for copper-mediated ATRP, while phosphorus-based ligands are rarely used due to less effectiveness. Therefore, this work aims to explore for the first time a facile, simple, and inexpensive ionic liquid (IL), 1-phenyl-3-methylimidazole diphenyl phosphate ([Phmim][Ph2PO4]), as an efficient phosphorus ligand for CuBr2-mediated reverse ATRP. The key to success is ascribed to stronger complexation of the IL ligand with the catalyst and higher solubility of the resulting complex. The polymerizations proceeded in a controlled/“living” fashion, as evidenced by first-order kinetics, linear evolution of molecular weights with monomer conversion, and narrow molecular weight distributions. Effects of various experimental parameters—solvent, reaction temperature, IL, and molar ratio of CuBr2/[Phmim][Ph2PO4]—on the polymerization were investigated in detail. Furthermore, 1H NMR analysis confirmed the halogen-containing chain-end functionality of the resultant polymer.
Introduction
Atom transfer radical polymerization (ATRP) has attracted considerable interest over the past twenty years because it can easily synthesize vinyl polymers with well-controlled molecular weights, narrow molecular weight distributions, and functional end-functionalities.1–5 Normal ATRP proceeds via a redox process where an alkyl halide is activated by a transition-metal catalyst, generating the corresponding alkyl radical and the transition-metal complex in its higher oxidation state.6–9 One serious disadvantage of ATRP is the air sensitivity of transition-metal complexes in their lower oxidation states.10 Reverse ATRP was developed to overcome the above drawback. It has two advantages in comparison with normal ATRP: a conventional radical initiator is used instead of an organic halide, which is generally toxic and expensive, and the transition-metal complex in its higher oxidation state is air-stable.11
Generally, ligand plays key role in solubilizing the transition-metal complex and in adjusting the redox potential of the metal center for appropriate reactivity in a ATRP process.12 Generally, nitrogen ligands work particularly well for copper-mediated ATRP but phosphorus-based ligands are rarely used ATRP due to less effectiveness. Ionic liquids (ILs) have drawn significant attention in the past few years. They have unique properties, such as low vapor pressure, non-flammability, and good solubility to transition-metal catalyst. Particularly, they are used as alternative for reaction media, extraction solvent for separation and recovery, and particularly used as ligand for ATRP.13–16
To date, there have many studies on ILs as reaction media or ligand for ATRP.17–26 The use of 1-butyl-3-methylimidazolium hexafluorophosphate ([C4mim][PF6]) as solvent for copper(I)-mediated ATRP of methyl methacrylate (MMA) was first reported by Carmichael et al.27 This report opened an avenue to the preparation of well-defined polymers by ATRP with IL as ligand. Particularly, IL as ligand instead of expensive nitrogen compounds for copper-catalyzed ATRP systems is possible. Matyjaszewski's group investigated copper(I) and iron(II)-catalyzed normal ATRP of MMA with various ILs as ligands.28 These ILs with 1-alkyl-3-methylimidazolium ([Rmim], R represents an alkyl group) as cations contained different anions such as Cl−, Br−, CO3−, AlCl4−, [(C4H9)2PO4]−, and etc. Furthermore, Lai's group explored the [Hmim][RCOO] used as ligand, ethyl 2-bromoisobutyrate/CuBr as the initiating system for normal ATRP of MMA.29,30 However, all of above mentioned copper-IL catalyzed systems were used for normal ATRP. To the best of our knowledge, only Zhou's group31,32 and Chen's group33 had used IL as solvent for copper(II)-catalyzed reverse ATRP. The ILs in these polymerization systems generally used expensive anion, such as PF6−, BF4−, and an organic ligand was usually required. Thus, it is curious to us why copper(II)-catalyzed reverse ATRP with IL as ligand was rarely reported.
The aim of this work is to prove the feasibility of the application of a novel IL, 1-phenyl-3-methylimidazole diphenyl phosphate ([Phmim][Ph2PO4]) as efficient phosphorus ligand for copper(II)-catalyzed reverse ATRP of MMA. Controlled/living polymerization characteristic were confirmed by kinetic studies as well as GPC and 1H NMR analyses.
Experimental
Materials
MMA (99%) was washed with 5% NaOH and distilled water several times to remove the inhibitor, and freshly distilled under reduced pressure after drying over Na2SO4. 2,2-Azobisisobutyronitrile (AIBN) was recrystallized twice from ethanol prior to use. Dimethyl sulfoxide (DMSO, 99%), toluene (99%), and tetrahydrofuran (THF, 99%) were dried over Na2SO4 before use. Cupric bromide (CuBr2, 99%, Aladdin), copper sulfate pentahydrate (CuSO4·5H2O, 99%), cupric nitrate trihydrate (Cu(NO3)2·3H2O, 99%), cupric acetate monohydrate (Cu(CH3COO)2·H2O, 99%), 1-methylimidazole (MIM, 99%, Aladdin), and triphenyl phosphate (Ph3PO4, 99%) were used as received without further purification.
Synthesis of [Phmim][Ph2PO4]
Triphenyl phosphate (32.63 g, 0.1 mol) and 1-methylimidazole (8.24 g, 0.1 mol) was added into a flask in sequence. Then the mixture was charged with nitrogen before sealed, and placed in an oil bath at 150 °C for 10 h. Finally, the obtained mixture was washed with petroleum ether three times to remove the unreacted triphenyl phosphate. The synthetic route of [Phmim][Ph2PO4] is shown in Scheme 1. Yield: 39.78 g (90 wt%). Element analysis: C 64.70%, H 5.18%, O 15.67% (calculated); C 58.76%, H 5.58%, O 14.02% (found). In addition, the 1H NMR spectra of [Phmim][Ph2PO4] is presented in Fig. 1. The signal at 3.56–3.95 ppm is attributed to the protons of methylene group (CH2, peak a) in imidazole, and the signals at 6.72–7.65 ppm are attributed to the protons of benzene (CH, peaks b–f).
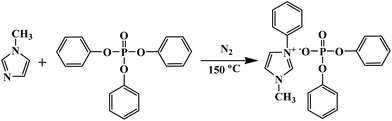 |
| Scheme 1 Synthetic route of [Phmim][Ph2PO4]. | |
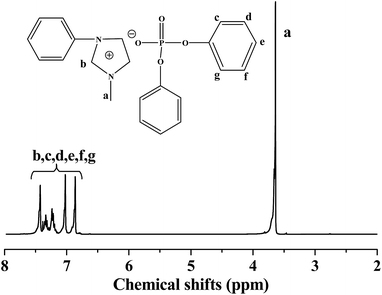 |
| Fig. 1 1H NMR spectrum of [Phmim][Ph2PO4]. Reaction conditions: [MIM]0/[Ph3PO4]0 = 1 : 1, reaction time = 10 h, T = 150 °C. | |
Polymerization
A typical polymerization procedure is as follows. MMA, CuBr2, AIBN, [Phmim][Ph2PO4], and THF with a predetermined molar ratio were added into a dry Schlenk tube. Then the tube was immersed in liquid nitrogen for one minute. Subsequently, the mixture was subject to three freeze–pump–thaw cycles to remove oxygen, charged with nitrogen, and sealed. The tube with the reaction mixture was placed in an oil bath at the predetermined reaction temperature. After a desired polymerization time, the polymerization mixture was cooled by ice water, exposed to air, and the content was then diluted with THF to achieve a homogenous solution. The polymer was then precipitated into a large excess of distilled water. The final polymer was separated by filtration, and dried under vacuum at 60 °C until a constant weight. The monomer conversion was determined gravimetrically.
Characterization
1H NMR spectra of PMMA samples were recorded on a Bruker 300 MHz nuclear magnetic resonance (NMR) instrument using CDCl3 as the solvent and tetramethylsilane (TMS) as the internal standard at ambient temperature. The number-average molecular weight (Mn,GPC) and PDI values of poly(methyl methacrylate) (PMMA) samples were determined by gel permeation chromatography (GPC). The GPC system contains a Waters 510 HPLC pump and Waters 2414 RI detector using three Waters Ultrastyragel columns (500, 103, and 105) in THF at a flow rate of 1.0 mL min−1. The columns were calibrated by PMMA narrow standards. Elemental analysis was performed on an elemental Vario EL cube spectrophotometer with an 80 bit autosampler and a “purge-trap” adsorption analytical column.
Results and discussion
Copper-catalyzed reverse ATRP of MMA utilizing 1-phenyl-3-methylimidazole diphenyl phosphate ([Phmim][Ph2PO4]) as the ligand
As mentioned previously, ILs are not efficient ligands for copper(II)-catalyzed reverse ATRP of MMA. Therefore, we first reasoned the feasibility of IL as phosphorus-based ligands ligand for Cu(II)-catalyzed reverse ATRP. Two phosphate-anionic IL ([Bmim][DBP] and [Phmim][Ph2PO4]) and a common halogen IL ([Bmim][Br]) were examined, and the results are presented in Table 1.
Table 1 Effect of ILs on reverse ATRP of MMAa
Entry |
ILs |
Conv. (%) |
Mn,th (Da) |
Mn,GPC (Da) |
PDI |
[MMA]0/[AIBN]0/[CuBr2]0/[IL]0 = 200 : 1 : 2 : 4, [MMA]0 = 9.4 mol L−1, time = 25 h, MMA/THF = 1 : 1 (v/v), T = 70 °C. |
1 |
[Bmim]Br |
50 |
5300 |
20 500 |
1.79 |
2 |
[Bmim][DBP] |
85 |
9100 |
16 100 |
1.39 |
3 |
[Phmim][Ph2PO4] |
95 |
10 100 |
15 800 |
1.27 |
Obviously, with a common and simple IL 1-butyl-3-methylimidazole bromine ([Bmim][Br]), the polymerization only gives 50% monomer conversion within 25 h (entry 1). Besides, the measured molecular weight value (Mn,GPC = 20
500) of the resultant PMMA is much larger than the theoretical one (Mn,th = 5300), and the PDI value is as high as 1.79. These results indicate that the polymerizations have not any differences in molecular dimensions compared with the conventional radical polymerization except that it is retarded by CuBr2.34 Instead, with phosphate-anionic IL, i.e., [Bmim][DBP] and [Phmim][Ph2PO4], the rate of polymerization enhanced greatly (entries 2 and 3). At the same time, the difference between measured molecular weight values and theoretical ones decreased considerably. The decreasing rule is also reflected in the PDI results. Particularly, [Phmim][Ph2PO4] provided the fastest rate of polymerization (95 monomer conversions after 25 h) and the highest control of the radical polymerization of MMA with the measured Mn,GPC very close to theoretical Mn,th and low PDI of 1.27 (entry 3). This may be ascribed to the fact that there exists much stronger complexation of [Phmim][Ph2PO4] with Cu(II) compound compared with [Bmim][Br], followed by [Bmim][DBP], and thus the increased solubility of catalytic system will avail the function of reverse ATRP process. As a result, [Phmim][Ph2PO4] is the optimal ligand for reverse ATRP under investigated conditions.
The polymerization catalyzed with CuBr2/[Phmim][Ph2PO4] in presence of AIBN as the radical source was investigated in detail to follow the evolutions of molecular weight and PDI values. The CuBr2–[Phmim][Ph2PO4] catalyzed reverse ATRP of MMA was first performed with [MMA]0/[AIBN]0/[CuBr2]0/[IL]0 = 200
:
1
:
2
:
4 in THF at 70 °C. The monomer conversion and ln([M]0/[M]) versus reaction time are plotted in Fig. 2. Clearly, a quasi-linear semilogarithmic kinetics is observed, suggesting that the propagating radical concentrations remained almost unchanged during the polymerization process. Additionally, there is a certain deviation from linearity, suggesting the presence of unavoidable termination or transfer reactions for radical polymerization. It is also noted that there is an induction period of 8.5 h at the beginning of polymerization.35 This indicates that the polymerization needs some time to decompose AIBN and establish a dynamic equilibrium between the Cu(I) and Cu(II) species.36,37 Besides, the inhibition caused by a high concentration of high valence copper at the initial stage or the incomplete degassing of oxygen may also lead to a lengthened induction period.
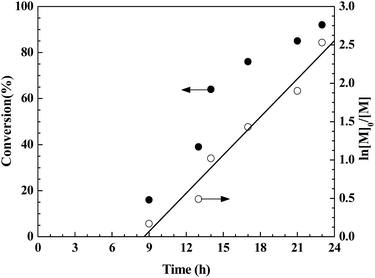 |
| Fig. 2 Kinetic plots of ln([M]0/[M]) versus reaction time for reverse ATRP of MMA in THF. Reaction conditions: [MMA]0/[AIBN]0/[CuBr2]0/[Phmim][Ph2PO4]0 = 200 : 1 : 2 : 4, [MMA]0 = 9.4 mol L−1, MMA/THF = 1 : 1 (v/v), T = 70 °C. | |
The Mn,th of PMMA obtained from reverse ATRP can be calculated based on the following equation:38
where [MMA]
0 and [AIBN]
0 correspond to the initial concentrations of MMA and AIBN, respectively. Conv. is the monomer conversion.
MMMA is the molecular weight of MMA. The value of
kd refers to decomposition rate at 70 °C, and
t corresponds to the reaction time.
Fig. 3 illustrates that the molecular weight (
Mn,GPC) values of the polymers increased linearly with monomer conversions. Meanwhile, the PDI values (1.25–1.46) of the obtained PMMA samples remained low throughout the polymerization process. However, a certain deviation from linearity is observed, implying that there were unavoidable termination or transfer reactions. The result can be clearly seen in the corresponding GPC curve of the polymers (
Fig. 4). The curves are nearly narrow and symmetrical. Moreover, the traces of the obtained typical polymers shift toward shorter elution time, indicating the increase of
Mn,GPC with monomer conversion. Nevertheless, the GPC traces are not symmetrical at higher yields, and the molecular weight distribution curves and PDI values do not gradually decrease, indicating a partial loss of control over the polymerization. Therefore the results suggest that the CuBr
2–[Phmim][Ph
2PO
4]-catalyzed reverse ATRP of MMA possess the controlled/“living” radical polymerization characteristics of ATRP.
37
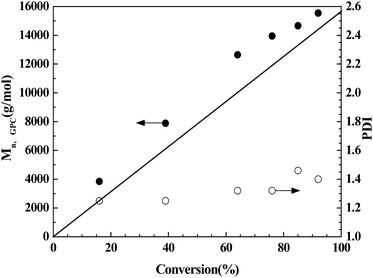 |
| Fig. 3 Evolution of Mn (— theoretical) and PDI with monomer conversion for reverse ATRP of MMA in THF. Reaction conditions: [MMA]0/[AIBN]0/[CuBr2]0/[Phmim][Ph2PO4]0 = 200 : 1 : 2 : 4, [MMA]0 = 9.4 mol L−1, MMA/THF = 1 : 1 (v/v), T = 70 °C. | |
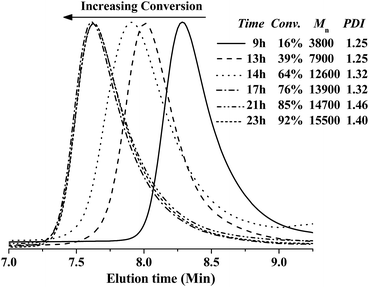 |
| Fig. 4 Evolution of GPC traces for reverse ATRP of MMA in THF at different monomer conversions: 16%, 39%, 64%, 76%, 85%, and 92%. Reaction conditions: [MMA]0/[AIBN]0/[CuBr2]0/[Phmim][Ph2PO4]0 = 200 : 1 : 2 : 4, [MMA]0 = 9.4 mol L−1, MMA/THF = 1 : 1 (v/v), T = 70 °C. | |
Effect of solvent
The effect of various solvent on CuBr2–[Phmim][Ph2PO4] catalyzed reverse ATRP of MMA was studied. As shown in Table 2, for the polymerization conducted in bulk, the monomer conversion reached 95% within 16 h (entry 1). The measured molecular weight value (Mn,GPC = 13
000) is very close to the theoretical one (Mn,th = 11
400). However, the measured PDI value is as high as 1.42. For the polymerization conducted in THF, the polymerization yielding 95% monomer conversion after 25 h (entry 2). The measured Mn,GPC = 15
800 is slightly higher than the theoretical Mn,th = 10
100. Particularly, the measured PDI value (PDI = 1.27) is the lowest among the investigated reaction medias. For the polymerization conducted in toluene, the polymerization achieved 96% monomer conversion within the same time (entry 3). Meanwhile, the measured molecular weight value Mn,GPC = 13
000 match with theoretical one Mn,th = 10
200. However, the measured PDI value is slightly high (i.e., 1.42). For the polymerization conducted in DMSO, the monomer conversion only reach 26% within 25 h, and both the measured Mn,GPC = 2600 was low (entry 4), but the measured PDI value (PDI = 1.40) is relatively high. To sum up, though bulk and toluene systems can yield fast rates of polymerizations (95% and 96% monomer conversions) and the measured PDI values reached up to 1.42. The obvious difference of the polymerization results may be attributed to the disproportionation reaction of the generated activator Cu+ for DMSO.39 For toluene, a nonpolar nature did not exhibit excellent solubility for the catalyst system. Therefore, THF is the optimal solvent for the investigated systems, as evidenced from the results that the corresponding polymerization obtained 95% monomer conversion, and produced well-defined PMMA with controlled Mn,GPC (15
800) close to Mn,th (10
100) as well as the lowest PDI value (1.27).
Table 2 Effect of solvent on reverse ATRP of MMAa
Entry |
Solvent |
Conv. (%) |
Mn,th (Da) |
Mn,GPC (Da) |
PDI |
[MMA]0/[AIBN]0/[CuBr2]0/[Phmim][Ph2PO4]0 = 200 : 1 : 2 : 4, [MMA]0 = 9.4 mol L−1, time = 25 h, MMA/solvent = 1 : 1 (v/v), T = 70 °C. Reaction time = 16 h. |
1b |
Bulk |
95 |
11 400 |
13 000 |
1.42 |
2 |
THF |
95 |
10 100 |
15 800 |
1.27 |
3 |
Toluene |
96 |
10 200 |
13 000 |
1.42 |
4 |
DMSO |
26 |
2800 |
2600 |
1.40 |
Effect of [AIBN]0/[CuBr2]0/[IL]0
For an ideal reverse ATRP, it is necessary to use an appropriate molar ratio of catalyst/ligand to obtain a high control of the polymerization.40 For the sake of comparison, the control experiment, the conventional free radical polymerization of MMA in absence of the CuBr2 or IL was first carried out at 70 °C. The polymerization results are summarized in entries 1–3 of Table 3. For the conventional radical polymerization in absence of CuBr2 and IL, the monomer conversion reached 91% within 10 h (entry 1). The measured molecular weight value Mn,GPC = 35
300 is much larger than theoretical one Mn,th = 13
500, and the PDI value is as high as 1.56. Likewise, for the polymerization system free of CuBr2, the monomer conversion reached 88% within 10 h, and the measured Mn,GPC = 33
400 is much larger than theoretical Mn,th = 13
100, and the PDI value is 1.87 (entry 2). Finally, the polymerization with only CuBr2 is greatly retarded of inhibited, yielding a low monomer conversion of 35% within 31 h as well as a low polymer molecular weight of 5600 and a high PDI value of 1.72 (entry 3). Therefore, the blank conventional radical polymerizations in presence of CuBr2 or IL cannot proceed via reverse ATRP process and were out of control under the investigated reaction conditions.
Table 3 Effect of molar ratio of CuBr2 to IL on reverse ATRP of MMAa
Entry |
[MMA]0/[AIBN]0/[CuBr2]0/[IL]0 |
Time (h) |
Conv. (%) |
Mn,th (Da) |
Mn,GPC (Da) |
PDI |
IL: [Phmim][Ph2PO4], [MMA]0 = 9.4 mol L−1, MMA/THF = 1 : 1 (v/v), T = 70 °C. |
1 |
200/1/0/0 |
10 |
91 |
13 500 |
35 300 |
1.56 |
2 |
200/1/0/4 |
10 |
88 |
13 100 |
33 400 |
1.87 |
3 |
200/1/2/0 |
31 |
35 |
3600 |
5600 |
1.72 |
4 |
200/1/0.5/1 |
25 |
88 |
9400 |
19 100 |
1.32 |
5 |
200/1/1/2 |
25 |
91 |
9700 |
16 300 |
1.27 |
6 |
200/1/2/4 |
25 |
95 |
10 100 |
15 800 |
1.27 |
7 |
200/1/2/6 |
25 |
94 |
10 000 |
21 600 |
1.39 |
8 |
200/1/2/8 |
25 |
92 |
9800 |
17 100 |
1.37 |
9 |
200/1/4/8 |
25 |
98 |
10 400 |
25 200 |
1.38 |
Alternatively, effect of [CuBr2]0/[IL]0 on the polymerization was investigated under similar reaction conditions. For entries 4–6, and 9, the molar ratio of [CuBr2]0/[IL]0 is 1
:
2. The polymerization reached 88% and 91% monomer conversion within 25 h for [AIBN]0/[CuBr2]0/[IL]0 = 1
:
0.5
:
1 and 1
:
1
:
2, respectively (entries 4–5). For [AIBN]0/[CuBr2]0/[IL]0 = 1
:
2
:
4, the polymerization gived 95% monomer conversion within 25 h (entry 6). Decreasing [AIBN]0/[CuBr2]0/[IL]0 down to 1
:
4
:
8, the polymerization yield 98% conversion within 25 h (entry 9). Thus lowering the molar ratio of [AIBN]0/[CuBr2]0/[IL]0 can slightly enhance the monomer conversion. The same rule can be seen from the evolution of the molecular weight and PDI values with different [AIBN]0/[CuBr2]0/[IL]0 values, as shown in Table 3, Fig. 5 and 6. With a high [AIBN]0/[CuBr2]0/[IL]0 value of 1
:
0.5
:
1, the corresponding PDI value is 1.32. With the decrease of [AIBN]0/[CuBr2]0/[IL]0 down to 1
:
1
:
2 and 1
:
2
:
4, the corresponding PDI values remain 1.27. Further decreasing the molar ratio of [AIBN]0/[CuBr2]0/[IL]0 to 1
:
4
:
8, the corresponding PDI value increased up to 1.38. In case of molecular weight, with the [AIBN]0/[CuBr2]0/[IL]0 of 1
:
2
:
4, the measured Mn,GPC = 15
800 is the closest to theoretical Mn,th = 10
100 among the four catalytic systems i.e. the initiator efficiency is the highest (Fig. 6). For entries 6–8, the monomer conversion decrease with increase of the amount of IL ([CuBr2]0/[IL]0 = 2
:
4, 2
:
6, and 2
:
8). With a molar ratio of [CuBr2]0/[IL]0 = 2
:
4, the measured Mn,GPC is close to theoretical one, and the PDI value (1.27) is the lowest among three polymerizations. Therefore, all these results indicate that selecting a suitable molar ratio of [CuBr2]0/[IL]0 = 2
:
4 is extremely important for reverse ATRP of MMA.
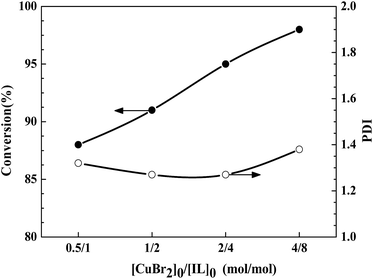 |
| Fig. 5 Dependence of conversion and PDI on the molar ratio of [CuBr2]0/[Phmim][Ph2PO4]0 for reverse ATRP of MMA in THF. Reaction conditions: [MMA]0/[AIBN]0 = 200 : 1, MMA/THF = 1 : 1 (v/v), [MMA]0 = 9.4 mol L−1, T = 70 °C. | |
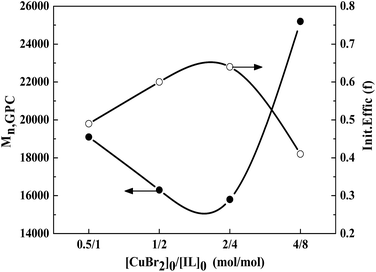 |
| Fig. 6 Dependence of Mn,GPC and initiator efficiency (f) on the molar ratio of [CuBr2]0/[Phmim][Ph2PO4]0 for reverse ATRP of MMA in THF. Reaction conditions: [MMA]0/[AIBN]0 = 200 : 1, MMA/THF = 1 : 1 (v/v), [MMA]0 = 9.4 mol L−1, T = 70 °C. | |
Effect of reaction temperature
Reaction temperature was also investigated in order to reduce polymerization duration and improve the control of molecular weight and PDI. For the temperature of 60–80 °C, the polymerizations yielded higher monomer conversions than 90 °C after 25 h (entries 1–3 in Table 4). However, when the temperature increased up to 90 °C, a lower monomer conversion of 75% within 25 h is observed (entry 4). The decrease in rate of polymerization is attributed to the high primary radical concentration at the start of the polymerization and the accompanying relatively fast rate of biradical termination, leading to the insufficient supply of reactive radicals at the later stage of polymerization.41 Concerning the control over the molecular weights at four investigated temperature, the polymers of higher molecular weight higher than 14
000 were all obtained, and the difference between measured molecular weight value and theoretical one tended to enlarge with the increase of reaction temperature. Nevertheless, more attention should be paid to the influence of reaction temperature on the PDI. Clearly, a lower temperature of 60 °C or higher temperatures of 80 and 90 °C will increase the PDI results. Comparatively speaking, 70 °C is an optimal temperature to provide a low PDI value of 1.27 together with a higher monomer conversion and controlled molecular weight of polymer.
Table 4 Effect of temperature on reverse ATRP of MMAa
Entry |
Temperature (°C) |
Conv. (%) |
Mn,th (Da) |
Mn,GPC (Da) |
PDI |
[MMA]0/[AIBN]0/[CuBr2]0/[Phmim][Ph2PO4]0 = 200 : 1 : 2 : 4, [MMA]0 = 9.4 mol L−1, time = 25 h, MMA/THF = 1 : 1 (v/v). |
1 |
60 |
94 |
9400 |
14 300 |
1.38 |
2 |
70 |
95 |
10 100 |
15 800 |
1.27 |
3 |
80 |
95 |
9500 |
26 900 |
1.32 |
4 |
90 |
75 |
7500 |
10 700 |
1.31 |
Chain-end functionality
The structure of PMMA obtained at 16% monomer conversion (Mn,GPC = 3800, PDI = 1.25) via CuBr2–[Phmim][Ph2PO4] catalyzed reverse ATRP with [MMA]0/[AIBN]0/[CuBr2]0/[Phmim][Ph2PO4]0 = 200
:
1
:
2
:
4 is characterized by 1H NMR technique. As exhibited in Fig. 7, the chemical shift at 0.65–1.25 ppm was reasonably assigned to the protons of methyl protons (CH3, peak b). The chemical shift at 1.33–2.10 ppm was attributed to methylene protons (CH2, peak a) in the main chain. The chemical shift at 3.48–3.71 ppm was attributed to methoxy protons (OCH3, peak c). Moreover, the chemical shift at 3.74–3.84 ppm corresponded to the protons of OCH3 adjacent to the bromine atom (peak d), proving the presence of the containing-halogen end group, CH2CCl(CH3)(COOCH3), which is consistent with the literature reports.42 The Mn,NMR (3100) value can be calculated from the ratio of the methylene protons (peak a) to the terminal ones (peak d), which is very close to the Mn,GPC (3800), indicating that the PMMA is end-capped by Br with high fidelity. Thus the radical polymerization of MMA mediated by CuBr2–[Phmim][Ph2PO4] follows the reverse ATRP mechanism.
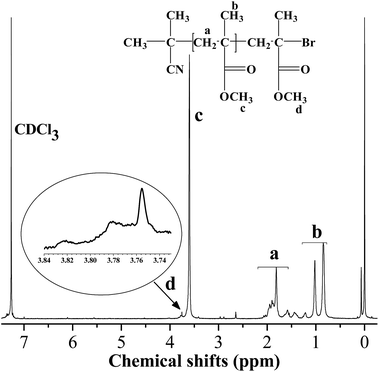 |
| Fig. 7 1H NMR spectrum of PMMA obtained from reverse ATRP of MMA in THF. Reaction conditions: [MMA]0/[AIBN]0/[CuBr2]0/[Phmim][Ph2PO4]0 = 200 : 1 : 2 : 4, [MMA]0 = 9.4 mol L−1, MMA/THF = 1 : 1 (v/v), reaction time = 9 h, monomer conversion = 16%, T = 70 °C. | |
Conclusions
Facile, simple, and inexpensive ionic liquid (IL), 1-phenyl-3-methylimidazole diphenyl phosphate ([Phmim][Ph2PO4]), has been successfully used as efficient phosphorus ligand for CuBr2-mediated reverse ATRP. The results show that IL provided the optimal controlled/living nature, as evidenced from ideal rate of polymerization, well control of molecular weight and PDI values, and bromine-containing chain-end fidelity confirmed by 1H NMR spectroscopy. Varied experimental parameters including solvent, reaction temperature, IL, and molar ratio of CuBr2/[Phmim][Ph2PO4] were tested to improve the control of the polymerization. Thus we believe that the facile, inexpensive, and simple catalytic system, the combination of phosphate IL and high-valence copper compound, provides a simple and efficient approach to reverse ATRP reactions of various vinyl monomers.
Conflicts of interest
There are no conflicts to declare.
Acknowledgements
The authors are grateful for subsidy provided by the National Natural Science Foundation of China (No. 21074127).
Notes and references
- P. Krys, H. Schroeder, J. Buback, M. Buback and K. Matyjaszewski, Macromolecules, 2016, 49, 7793–7803 CrossRef CAS.
- P. Leophairatana, S. Samanta, C. C. De Silva and J. T. Koberstein, J. Am. Chem. Soc., 2017, 139, 3756–3766 CrossRef CAS PubMed.
- T. G. Ribelli, S. Wahidur Rahaman, J. C. Daran, P. Krys, K. Matyjaszewski and R. Poli, Macromolecules, 2016, 49, 7749–7757 CrossRef CAS.
- G. Wang, M. Schmitt, Z. Wang, B. Lee, X. Pan, L. Fu, J. Yan, S. Li, G. Xie and M. R. Bockstaller, Macromolecules, 2016, 49, 8605–8615 CrossRef CAS.
- Q. Yang, J. Lalevée and J. Poly, Macromolecules, 2016, 49, 7653–7666 CrossRef CAS.
- K. Matyjaszewski, Macromolecules, 2012, 45, 4015–4039 CrossRef CAS.
- J. C. Theriot, C. H. Lim, H. Yang, M. D. Ryan, C. B. Musgrave and G. M. Miyake, Science, 2016, 352, 1082–1086 CrossRef CAS PubMed.
- J. Ran, L. Wu, Z. Zhang and T. Xu, Prog. Polym. Sci., 2014, 39, 124–144 CrossRef CAS.
- X. H. Liu, F. J. Zhang, H. N. Li, X. H. Hu, W. L. Di and S. Zhao, J. Appl. Polym. Sci., 2015, 132, 2410–2415 Search PubMed.
- J. S. Wang and K. Matyjaszewski, J. Am. Chem. Soc., 1995, 117, 5614–5615 CrossRef CAS.
- J. Xia and K. Matyjaszewski, Macromolecules, 1997, 30, 7692–7696 CrossRef CAS.
- A. Anastasaki, C. Waldron, P. Wilson, R. McHale and D. M. Haddleton, Polym. Chem., 2013, 4, 2672–2675 RSC.
- Y. H. Yu, X. H. Liu, D. Jia, B. W. Cheng, F. J. Zhang, H. N. Li, P. Chen and S. Xie, J. Polym. Sci., Part A: Polym. Chem., 2013, 51, 1468–1474 CrossRef CAS.
- X. H. Liu, J. Wang, J. S. Yang, S. L. An, Y. L. Ren, Y. H. Yu and P. Chen, J. Polym. Sci., Part A: Polym. Chem., 2012, 50, 1933–1940 CrossRef CAS.
- W. Tang and K. Matyjaszewski, Macromol. Theory Simul., 2008, 17, 359–375 CrossRef CAS.
- C. Boyer, A. H. Soeriyadi, P. B. Zetterlund and M. R. Whittaker, Macromolecules, 2016, 44, 8028–8033 CrossRef.
- A. Anastasaki, V. Nikolaou, G. Nurumbetov, N. P. Truong, G. S. Pappas, N. G. Engelis, J. F. Quinn, M. R. Whittaker, T. P. Davis and D. M. Haddleton, Macromolecules, 2015, 48, 5140–5147 CrossRef CAS.
- Y. Zhou, L. Qiu, Z. Deng, J. Texter and F. Yan, Macromolecules, 2011, 44, 7948–7955 CrossRef CAS.
- S. G. N. Brusseau, O. Boyron, C. Schikaneder, C. C. Santini and B. Charleux, Macromolecules, 2010, 44, 215–220 CrossRef.
- C. Hou, R. Qu, C. Ji, C. Sun and C. Wang, J. Polym. Sci., Part A: Polym. Chem., 2008, 46, 2701–2707 CrossRef CAS.
- Y. Liang, H. Chen and W. Zhou, J. Macromol. Sci., Part A: Pure Appl.Chem., 2009, 46, 759–764 CrossRef CAS.
- H. Chen, Y. Meng, Y. Liang, Z. Lu and P. Lv, J. Mater. Res., 2009, 24, 1880–1885 CrossRef CAS.
- J. Ma, H. Chen, M. Zhang and M. Yu, J. Polym. Sci., Part A: Polym. Chem., 2015, 50, 609–613 CrossRef.
- G. Wang, M. Lu, M. Zhong and H. Wu, J. Polym. Res., 2012, 19, 1–6 CrossRef CAS.
- X. Du, J. Pan, M. Chen, L. Zhang, Z. Cheng and X. Zhu, Chem. Commun., 2014, 50, 9266–9269 RSC.
- Z. Deng, L. Qiu, L. Bai, Y. Zhou, B. Lin, J. Zhao, Z. Cheng, X. Zhu and F. Yan, J. Polym. Sci., Part A: Polym. Chem., 2012, 50, 1605–1610 CrossRef CAS.
- A. J. Carmichael, D. M. Haddleton, S. A. Bon and K. R. Seddon, Chem. Commun., 2000, 1237–1238 RSC.
- T. Sarbu and K. Matyjaszewski, Macromol. Chem. Phys., 2001, 202, 3379–3391 CrossRef CAS.
- G. Q. Lai, F. M. Ma, Z. Q. Hu, H. Y. Qiu, J. X. Jiang, J. R. Wu, L. M. Chen and L. B. Wu, Chin. Chem. Lett., 2007, 18, 601–604 CrossRef CAS.
- F. Ma, W. Li, G. Lai, J. Guo, M. Ruan and W. Qin, J. Test. Eval., 2011, 39, 967–973 CAS.
- H. Ma, X. Wan, X. Chen and Q. F. Zhou, J. Polym. Sci., Part A: Polym. Chem., 2003, 41, 143–151 CrossRef CAS.
- H. Ma, X. Wan, X. Chen and Q. F. Zhou, Polymer, 2003, 44, 5311–5316 CrossRef CAS.
- H. Chen, Y. Liang, M. Wang, P. Lv and Y. Xuan, Chem. Eng. J., 2009, 147, 297–301 CrossRef CAS.
- K. Matyjaszewski, A. K. Nanda and W. Tang, Macromolecules, 2005, 38, 2015–2018 CrossRef CAS.
- X. H. Liu, H. N. Li, F. J. Zhang, S. Xie, Z. J. Liu and Y. G. Li, Chin. J. Polym. Sci., 2015, 33, 362–370 CrossRef CAS.
- Y. H. Yu, X. H. Liu, D. Jia, B. W. Cheng, Y. L. Ren, F. J. Zhang, H. N. Li, P. Chen and S. Xie, J. Polym. Sci., Part A: Polym. Chem., 2013, 51, 1690–1694 CrossRef CAS.
- Y. H. Yu, X. H. Liu, D. Jia, B. W. Cheng, F. J. Zhang, P. Chen and S. Xie, Polymer, 2013, 54, 148–154 CrossRef CAS.
- J. S. Wang and K. Matyjaszewski, Macromolecules, 1995, 28, 7572–7573 CrossRef CAS.
- Y. H. Yu, X. H. Liu, D. Jia, B. W. Cheng, F. J. Zhang, P. Chen and S. Xie, J. Polym. Sci., Part A: Polym. Chem., 2013, 51, 1559–1564 CrossRef.
- X. Liu, Q. Zhu, Q. Zhang, Y. Zhang and C. Ding, RSC Adv., 2017, 7, 6972–6980 RSC.
- T. G. Ribelli, D. Konkolewicz, X. Pan and K. Matyjaszewski, Macromolecules, 2014, 47, 6316–6321 CrossRef CAS.
- W. Zhang, X. Zhu, J. Zhu and Z. Cheng, Macromol. Chem. Phys., 2004, 205, 806–813 CrossRef CAS.
|
This journal is © The Royal Society of Chemistry 2017 |