DOI:
10.1039/C7RA08905H
(Paper)
RSC Adv., 2017,
7, 52955-52961
Novel rod-Y2O3:Eu3+@0.01YVO4:Eu3+ with open core/shell nanostructure and “off-and-on” fluorescent performance†
Received
11th August 2017
, Accepted 9th November 2017
First published on 16th November 2017
Abstract
Herein, we develop a novel sensitive fluorescent probe with an open core/shell nanostructure (OCSNS), rod-Y2O3:Eu3+@0.01YVO4:Eu3+, in which a YVO4:Eu3+ shell with a thickness of less than 5 nm partially covers an anisotropic core of rod-Y2O3:Eu3+. The photo-luminescent performance of the OCSNS is sensitive to trace ions or molecules. High-resolution transmission electron microscopy (HR-TEM) observations clearly confirm that this OCSNS has an open quantum shell of YVO4:Eu3+. In contrast to closed core Y2O3:Eu3+@shell YVO4:Eu3+, which has only one excitation band peaking at ∼308 nm, this novel nanostructure has a wide-wavelength excitation response that can be decomposed into two components centered at ∼254 and ∼280 nm, which stem from open core rod-Y2O3:Eu3+ and the YVO4:Eu3+ quantum shell, respectively. As expected, this OCSNS shows “off-and-on” fluorescence properties due to the strong surface effect of this open quantum shell: it is “off” when detecting trace Cu(II) ions and “on” when detecting trace amino acids, at levels as low as 10−8 mol L−1. Hence, rod-Y2O3:Eu3+@0.01YVO4:Eu3+ could be an excellent candidate for sensitive fluorescent probes, showing similar performance to most quantum dots for the detection of ions and molecules. Moreover, it is both easier to fabricate and more stable than quantum dots.
1. Introduction
Rare-earth-doped nano-phosphors have unique properties and applications in many fields, such as white light-emitting diodes, field emission displays, and general fluorescence lamps.1–7 They are highly popular among researchers and are gaining increasing importance in our daily lives. Y2O3:Eu3+ and YVO4:Eu3+ are well-known red-emitting phosphors. Y2O3:Eu3+ shows excellent luminescent performance with high brightness, color purity, and stability.5,8 YVO4:Eu3+ also shows prominent luminescent performance owing to the strong 5D0–7F2 transition due to the efficient energy transfer from VO43− to Eu3+, which provides a high quantum yield of photoluminescence (PL q ≈ 70%).9,10 Rare-earth-doped nanocomposites with core/shell structures have been investigated by many researchers in attempts to obtain outstanding luminescent performance: examples with close and continuous shells on their cores include Y2O3:Eu3+@SiO2,11 CePO4:Tb/LaPO4,12 SiO2@YVO4:Dy3+/Sm3+,13 γ-Fe2O3@YVO4:Eu3+,14 and Y2O3:Eu3+@SiO2@YVO4:Eu3+.15
However, these reported nanostructures had fully coated shells, and therefore showed independent shell-only or core-only PL properties on energy transfer from the shell (or core) to the core (or shell). It is reasonable to assume that their lifetimes in both the core and shell depended on the 5D0–7F2 transitions in the Eu3+ ions, giving different results for each system.15 The preparation processes for obtaining these core–shell nanostructures were complex; furthermore, their fluorescences were not significantly affected by trace surrounding molecules and ions, as shown by the limited variation in the before-and-after fluorescence intensities. Therefore, these nanomaterials generally cannot be used as fluorescent probes.
Recently, quantum dots (QDs) have drawn great attention because of their unique physical and chemical properties and potential applications.16 These nanoparticles have been employed in diverse applications such as light-absorbing components of solar cells17–19 and light-emitting components of LEDs and lasers,20 especially as fluorescent probes for trace detection of some molecules and ions.21 “Off-and-on” fluorescent probes are generally composed of many kinds of QDs and have attracted great attention because of their sensitive response to some trace ions and molecules. For example, diethyldithiocarbamate-functionalized CdSe/CdS QDs have been used as a fluorescent probe for copper ion detection,22 manganese oxide QDs have been applied as a fluorescent probe for the detection of metal ions in aqueous media,23 and carbon dots have been used as fluorescent probes for “off–on” detection of Cu2+ and L-cysteine in aqueous solutions.24 However, agglomeration of these QDs can easily occur during their preparation and preservation as well as application because of their high surface energies, making them unstable. In addition, their PLs are easily affected by many external factors. As a result, their fluorescence properties change very easily.
In this study, we develop a luminescent nanomaterial with wide-wavelength response and good stability for use as a PL probe in aqueous solutions. Specifically, a novel open core–shell nanostructure, rod-Y2O3:Eu3+@0.01YVO4:Eu3+, was designed and prepared, in which the core was partially coated with a quantum shell. In the open nanostructure proposed in this paper, the quantum shell plays a key role in the sensitivity of the material to trace ions.
2. Experimental section
2.1 Synthesis process
All the chemical reagents were analytical grade. Aqueous solutions of the precursors, Y(NO3)3 and Eu(NO3)3, were obtained by dissolving 1.061 g of Y2O3 and 0.0105 g of Eu2O3 (99.99%) in dilute HNO3, and the resulting mixtures were then heated to remove excess HNO3 solution. Next, 2 mol L−1 of NaOH solutions were used to adjust the pH of the above mixtures to 9. Finally, 0.0110 g of NH4VO3 (molar ratio nV
:
nY = 0.01) was dispersed into the above suspensions, which were then subjected to ultrasonication for 15 min. The prepared precursor solutions were then transferred to a 100 mL autoclave and heated at 180 °C for 24 h. After cooling, the sample was collected, washed several times with water and ethanol, dried at 60 °C, and then annealed at 800 °C for 3 h. The final sample was identified as the partially coated core@shell rod-Y2O3:Eu3+@0.01YVO4:Eu3+.
2.2 Fabrication and measurement of Cu2+ (off) and amino acid (on) sensor
Rod-Y2O3:Eu3+@0.01YVO4:Eu3+ (5 mL, 0.03 mg mL−1) was placed in a centrifugal tube. Then, Cu(NO3)2 was also added to the container, giving a final concentration of Cu2+ ranging from 10−4 to 10−10 mol L−1. After shaking well, the fluorescence spectra of the solutions were monitored under excitation at 280 nm.
For the detection of amino acids, 10−8 M of Cu2+ was added to rod-Y2O3:Eu3+@0.01YVO4:Eu3+ (5 mL, 0.03 mg mL−1) to form a mixed solution, which was denoted as rod-Y2O3:Eu3+@0.01YVO4:Eu3+–Cu2+ (10−8 mol L−1). Amino acid was then added, with the final concentration of amino acid ranging from 0 to 1.0 × 10−8 mol L−1. After shaking well, the fluorescence spectra of the solutions were monitored under excitation at 280 nm.
2.3 Characterization
The X-ray diffraction (XRD) patterns of the prepared samples were examined on an X-ray powder diffractometer (AXSD8-Advance, Bruker Company, Germany) with Cu Kα radiation (λ = 0.15418 nm, 40 kV, 30 mA). The Fourier transform infrared spectroscopy (FTIR) spectra were recorded on a Nicolet (Impact 410) infrared spectrophotometer using KBr pellets over the range of 400–4000 cm−1. The morphologies of the samples were observed using scanning electron microscopy (SEM) (ZEISS ULTRA 55, Carl Zeiss NTS GmbH, Germany) and high-resolution transmission electron microscopy (HR-TEM, JEOL JEM-2100HR, Japan). The excitation and emission spectra were taken on Hitachi F-4600 spectrofluorometer equipped with a 150 W xenon lamp as the excitation source. All the measurements were performed at room temperature.
3. Results and discussion
3.1 Structural and morphological properties of rod-Y2O3:Eu3+@0.01YVO4:Eu3+ nanoparticles
XRD. The XRD pattern of the partially coated rod-Y2O3:Eu3+@0.01YVO4:Eu3+ nanostructure was collected, as shown in Fig. 1, and all of the XRD diffraction peaks can be readily indexed to the cubic structure of Y2O3 and the tetragonal structure of YVO4, in accordance with the JCPDS cards 41-1105 and 17-0341. No additional diffraction peaks were observed, showing that there were no other impurities in this sample.
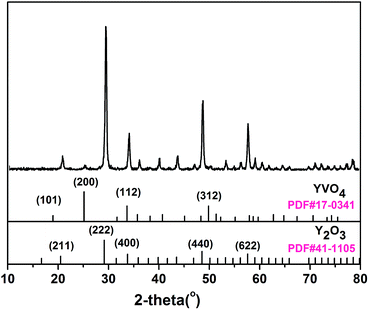 |
| Fig. 1 XRD pattern of rod-Y2O3:Eu3+@0.01YVO4:Eu3+. | |
FT-IR. The FTIR spectra of pure Y2O3:Eu3+ and rod-Y2O3:Eu3+@0.01YVO4:Eu3+ are exhibited in Fig. 2. As shown in Fig. 2a, absorption peaks at 3441, 1520, 1410, and 560 cm−1 can be clearly observed in the spectrum of Y2O3:Eu3+. A broad peak can also be seen at 3441 cm−1 due to the stretching mode of the hydroxyl groups.25,26 Meanwhile, the typical absorption peak corresponding to the Y–O stretching vibration of Y2O3 is present at 560 cm−1.27 Furthermore, a new vibration peak can be seen in Fig. 2b at 821 cm−1, which can be attributed to the V–O stretching vibration in the VO43− tetrahedron.28 The FT-IR spectra reveal clearly that Y2O3:Eu3+ and YVO4:Eu3+ co-existed in the prepared nanocomposite.
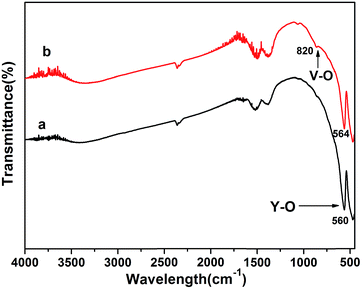 |
| Fig. 2 FT-IR spectra of pure Y2O3:Eu3+ (a) and rod-Y2O3:Eu3+@0.01YVO4:Eu3+ (b). | |
SEM, EDS, and HR-TEM. The morphology of rod-Y2O3:Eu3+@0.01YVO4:Eu3+ is exhibited in Fig. 3. The particles were uniform and monodispersed rods, with an average diameter of ∼50 nm and a length of ∼400 nm. In order to determine the chemical composition of the rod-Y2O3:Eu3+@0.01YVO4:Eu3+, EDS measurement was performed on the area of the material SEM in Fig. 4. The EDS analysis indicated that the main composition of the nanorods is yttrium and oxygen. Small amount of vanadium and europium were also detected. These results indicated that the chemical composition of the rod-Y2O3:Eu3+@0.01YVO4:Eu3+ is uniform.
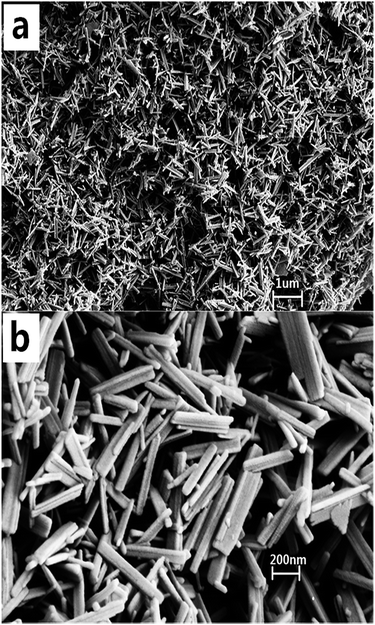 |
| Fig. 3 SEM images of rod-Y2O3:Eu3+@0.01YVO4:Eu3+. | |
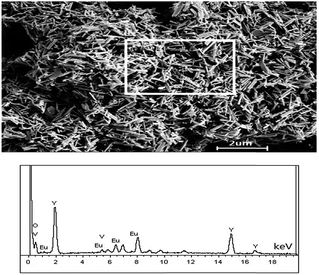 |
| Fig. 4 A SEM image of as-prepared nanorods (up) with collection area marked, and EDX signals (down, EDS spectrum). | |
Furthermore, the rod-Y2O3:Eu3+@0.01YVO4:Eu3+ was examined by TEM and HR-TEM in order to verify its partially coated structure, as displayed in Fig. 5. The composite structure of the rod-Y2O3:Eu3+@0.01YVO4:Eu3+ was investigated with a HRTEM, some breaking areas could be found on the rod and it exhibited a discontinuous interface. As shown in Fig. 5b1, the measured lattice spacing of the nanorod was 0.35 nm, indicating a (200) crystal growth direction and a SAED pattern recorded (left) from the same area, which confirmed that the nanorod is single crystalline and indicated that the growth direction is (200) of YVO4:Eu3+.9 Therefore, a lattice spacing of the crystalline can be identified 0.29 nm (222) crystal growth direction of Y2O3:Eu3+ in Fig. 5b2.29 Obviously, the partially coated structure had been successfully synthesized, while the YVO4:Eu3+ shell partially covered the Y2O3:Eu3+ core with a size less than 5 nm, similar emission property expected to that of a typical quantum dot.
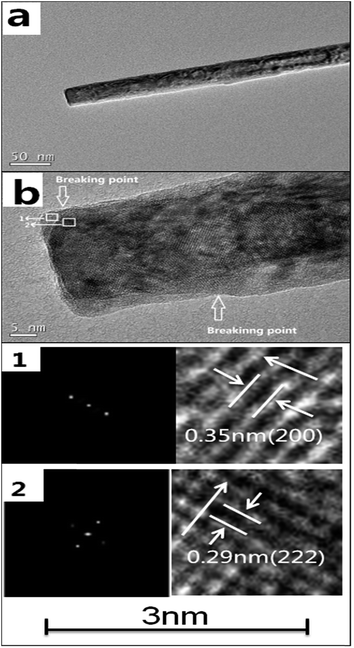 |
| Fig. 5 TEM (a) and HRTEM (b) images of rod-Y2O3:Eu3+@0.01YVO4:Eu3+ (b1, b2: representative SAED patterns and the measured lattice spacing of the crystalline). | |
PL. The excitation spectra of pure Y2O3:Eu3+ (a), pure YVO4:Eu3+ (b), and rod-Y2O3:Eu3+@0.01YVO4:Eu3+ (c) are shown in Fig. 6 (left). Only one peak appeared for pure Y2O3:Eu3+, at 254 nm, which corresponded to the charge-transfer band (CTB) related to an electronic transition from the 2p orbital of O2− to the 4f orbital of Eu3+.30 Pure YVO4:Eu3+ also only possessed one excitation band, at 308 nm, which can be regarded as a charge transfer from the O atoms of the ligands to the central V atom inside the VO43− group.31 However, the spectrum of rod-Y2O3:Eu3+@0.01YVO4:Eu3+ contained a broader excitation band in the range of 200–350 nm, which can be seen as two peaks centered at ∼254 nm and ∼280 nm, similar to that of a ∼5 nm YVO4:Eu3+ quantum dot layer, albeit slightly blue-shifted.32 The former peak was likely related to the CTB in Y2O3:Eu3+, while the latter corresponded to a vanadate band in the open quantum shell of YVO4:Eu3+. This special optical property is only observed in partially coated core/shell structures, and cannot be replicated in fully coated core/shell materials. In order to show this, a sample was prepared with more NH4VO3, corresponding to a V/Y molar ratio of 0.4. SEM and HR-TEM images of the nanocomposite prepared with 0.436 g of NH4VO3 (molar ratio nV
:
nY = 0.4) are shown in Fig. S1 (see ESI†). The HR-TEM image shows that a continuous interface can be clearly observed, meaning that the shell of YVO4:Eu3+ had totally covered the surface of the core Y2O3:Eu3+. The excitation spectrum of this material is shown in Fig. S2.† Surprisingly, the full coated sample showed only one excitation band at 308 nm, corresponding to that of YVO4:Eu3+. The continuous shell fully encompassed the core, enhancing the transition competition between the shell and the core. It can be concluded that efficient energy transfer from Y2O3:Eu3+ to YVO4:Eu3+ occurred following the excitation into the CTB of Y2O3:Eu3+.33 Therefore, the novel nanostructure rod-Y2O3:Eu3+@0.01YVO4:Eu3+, in which YVO4:Eu3+ was only partially coated on the Y2O3:Eu3+ core, acted as a smart phosphor which exhibited PL under wide-band excitation covering the range of 200–350 nm, as displayed in Fig. 6. This wide-band response phosphor emitted strong red light, and may be a candidate as a fluorescent probe for use in chemical and biological detection.
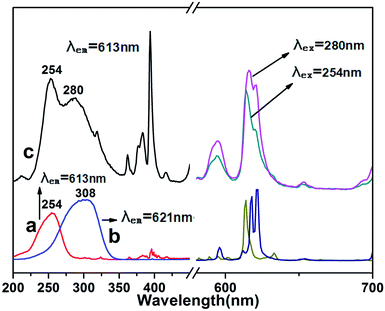 |
| Fig. 6 Excitation (left) and emission (right) spectra of pure Y2O3:Eu3+ (a), pure YVO4:Eu3+ (b), and rod-Y2O3:Eu3+@0.01YVO4:Eu3+ (c). | |
Cu2+ (off) and amino acid (on) sensing characteristics of rod-Y2O3:Eu3+@0.01YVO4:Eu3+ nanoparticles. The fluorescence quenching that occurs upon the addition of Cu2+ can be observed in Fig. 7, which shows the fluorescence intensity of the partially coated rod-Y2O3:Eu3+@0.01YVO4:Eu3+ decreasing gradually as the Cu2+ concentration was increased. Meanwhile, pure Y2O3:Eu3+ (Fig. S3†), pure YVO4:Eu3+ (Fig. S4†), Y2O3:Eu3+ coated with 0.01YVO4:Eu3+ by physical blending (Fig. S5†), and fully coated Y2O3:Eu3+@0.4YVO4:Eu3+ (Fig. S6†) were exposed to the same conditions to illustrate the excellent properties of the partially coated structure. Fig. 8 shows that the partially coated rod-Y2O3:Eu3+@0.01YVO4:Eu3+ had a linear relationship with the Cu2+ concentration (linear regression equation: y = 0.0396x + 0.5655, R2 = 0.9962), with the fluorescence intensity decreasing with increasing concentration of Cu2+. However, there were no obvious linear relationships between the concentration of Cu2+ and the other four materials. The partially coated nanostructures were also tested in the presence of 2, 4, 6, and 8 × 10−8 mol L−1 (Fig. S7†) and 2, 4, 6, and 8 × 10−10 mol L−1 (Fig. S8†) of Cu2+. Good linear relationships were also observed for these Cu(II) concentrations. In Fig. 9, I and I0 are the fluorescence intensities of Y2O3:Eu3+@0.01YVO4:Eu3+ in the presence and absence of Cu2+, respectively. Linear regression equations can be obtained for I/I0 under these conditions, which are y = −1.4175x + 85.365, R2 = 0.9947 ((a): 2, 4, 6, 8 × 10−8 mol L−1 grads) and y = −1.076x + 93.51, R2 = 0.9954 ((b): 2, 4, 6, 8 × 10−10 mol L−1 grads).
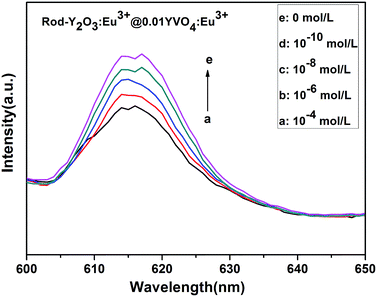 |
| Fig. 7 Fluorescence response of rodY2O3:Eu3+@0.01YVO4:Eu3+ in the presence of increasing concentration of Cu2+. | |
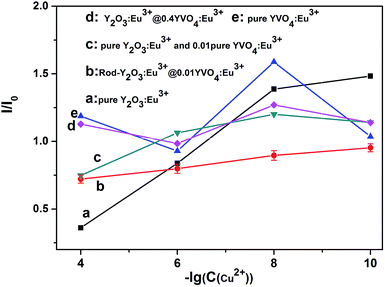 |
| Fig. 8 I/I0 relationships of five different samples with increasing Cu2+ concentration. | |
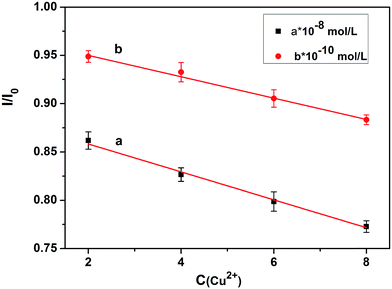 |
| Fig. 9 Linear I/I0 relationships versus concentration of Cu2+ over the ranges of 2–8 × 10−8 mol L−1 and 2–8 × 10−10 mol L−1, respectively. The error bars represent the standard deviations of three measurements. | |
This shows that the partially coated rod-Y2O3:Eu3+@0.01YVO4:Eu3+ nanostructures possess excellent properties that make them suitable for use as a fluorescent probe for the detection of Cu(II) ions. Quantum dots are already frequently used for the detection of minute quantities of elements. The partial YVO4:Eu3+ shells are as thin as quantum dots are small, providing outstanding properties that enable the detection of minute quantities of elements via use as a fluorescent probe. The emission intensity of the rod-Y2O3:Eu3+@0.01YVO4:Eu3+ decreased as the concentration of Cu(II) ions increased. Thus, a fluorescently quenched probe (off state) was obtained.
The quenching (off) of the rod-Y2O3:Eu3+@0.01YVO4:Eu3+–Cu2+ (10−8 mol L−1) system can be explored as a new fluorescent probe for the detection of amino acids based on the competition mechanism between amino acids, Cu2+, and rod-Y2O3:Eu3+@0.01YVO4:Eu3+. Glutamic acid and leucine were employed to illustrate this principle. The fluorescence spectra of rod-Y2O3:Eu3+@0.01YVO4:Eu3+–Cu2+ (10−8 mol L−1) with glutamic acid (Fig. S9†) and leucine (Fig. S10†) were measured, and the relationships between the fluorescence intensities of the rod-Y2O3:Eu3+@0.01YVO4:Eu3+–Cu2+ and the concentrations of the amino acids are shown in Fig. 10 (glutamic acid) and Fig. 11 (leucine). The linear regression equations (y = 111.85 + 24.98x, R2 = 0.9982 (glutamic acid) and y = 248.40 + 19.96x, R2 = 0.9986 (leucine)) indicate that the rod-Y2O3:Eu3+@0.01YVO4:Eu3+–Cu2+ (10−8 mol L−1) system can be used as a fluorescent probe for amino acids, as the fluorescence intensities of these samples increased with the concentration of amino acids, as the fluorescence intensity of the sample was restored (on state).
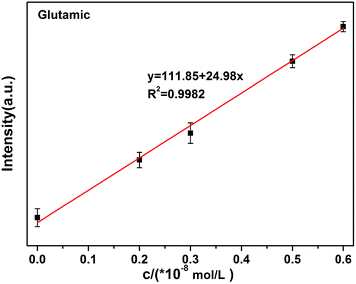 |
| Fig. 10 Linear relationship of the fluorescence intensity versus the concentration of glutamic acid over the range of 0–1.0 × 10−8 mol L−1. The error bars represent the standard deviations of three measurements. | |
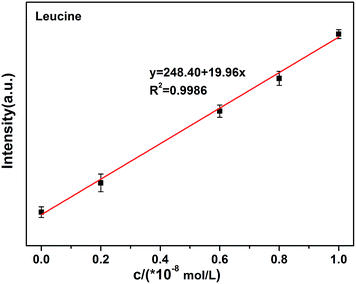 |
| Fig. 11 Linear relationship of the fluorescence intensity versus the concentration of leucine over the range of 0–1.0 × 10−8 mol L−1. The error bars represent the standard deviations of three measurements. | |
The mechanism of the “off-and-on” detection of Cu2+ and amino acids using anisotropic rods of Y2O3:Eu3+ that were partially coated with 0.01YVO4:Eu3+ as fluorescent probes is shown in Scheme 1. There were many –OH groups on the surface of the YVO4:Eu3+ quantum shell in the rod-Y2O3:Eu3+@0.01YVO4:Eu3+ composite nanostructure while dispersed in aqueous solution, which can easily combine with Cu2+ to form [Cu(OH)4]−. As is well known, [Cu(OH)4]− ions appear blue in aqueous solutions due to their absorption of red light, while the rod-Y2O3:Eu3+@0.01YVO4:Eu3+ nanocomposite is a red phosphor when excited with UV light. Therefore, the fluorescence intensity of the rod-Y2O3:Eu3+@0.01YVO4:Eu3+ nanocomposite was reduced or even quenched in the presence of trace Cu2+, allowing this OCSNS to be used as a probe for the detection of Cu2+. On other hand, the fluorescence intensity of the rod-Y2O3:Eu3+@0.01YVO4:Eu3+–Cu2+ (10−8 mol L−1) system was increased in the presence of trace amino acids. The amino acids possess a strong binding preference toward Cu2+, which removed Cu2+ from the surface of the partially coated nanocomposite, thus decreasing the concentration of [Cu(OH)4]− and allowing the fluorescence of the system to recover. The opposite fluorescent responses in the presence of Cu2+ and amino acids give this rod-Y2O3:Eu3+@0.01YVO4:Eu3+ OCSNS the potential to be used to detect trace Cu2+ and amino acids, exhibiting “off-and-on” fluorescence properties.
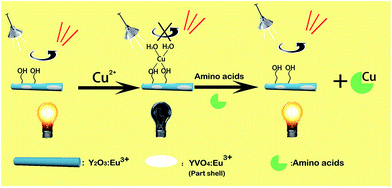 |
| Scheme 1 Schematic illustration of Cu2+ and amino acid detection using rod-Y2O3:Eu3+@0.01YVO4:Eu3+. | |
4. Conclusions
In conclusion, partially coated core–shell nanostructures, rod-Y2O3:Eu3+@0.01YVO4:Eu3+, were first fabricated by a facile hydrothermal approach. The crystal structure, morphology, and optical properties were characterized in detail by XRD, FTIR, SEM, TEM, and PL spectroscopy. This revealed that a quantum shell of YVO4:Eu3+ had been partially coated onto the surfaces of the Y2O3:Eu3+ core. The excitation spectra of this special nanostructure can be deconstructed into two components centered at ∼254 and ∼280 nm, covering a much wider excitation period of UV wavelengths than is obtained for either material alone. Other OCSNSs composed of an anisotropic core or substrate and an open shell with a thickness of less than 5 nm can also be fabricated in the same way, and the photo-luminescent performances of these materials in the presence of trace ions or molecules can be examined. As an example, super sensitive probes consisting of rod-Y2O3:Eu3+@0.01YVO4:Eu3+ with “off-and-on” properties can be employed to detect minute amounts of Cu(II) ions (off), followed by reuse as a fluorescent probe for various amino acids (on). Far more examples of this kind of OCSNS, their probing properties, and their selectivity performances will be examined in the future for the purpose of inventing novel fluorescent probes.
Conflicts of interest
There are no conflicts to declare.
Acknowledgements
The authors acknowledge the financial support from the National Natural Science Foundation of China (Grant No. 11374109) and the Natural Science Foundation of Guangdong Province, China (Grant. No. 2016A030308010). S. Lan and S. Tie would like to thank the financial support from the Science and Technology Planning Project of Guangdong Province, China(Grant No. 2015B090927006).
References
- D. Y. Fan, S. Yang, J. Q. Wang, A. Q. Zheng, X. P. Song and D. M. Yu, J. Lumin., 2012, 132, 1122–1125 CrossRef CAS.
- S. J. Wang, J. B. Hu, Y. Y. Wang and F. Luo, J. Mater. Sci., 2013, 48, 805–811 CrossRef CAS.
- S. Zhang, H. B. Liang, C. M. Liu, Z. M. Qi, T. Shao and Y. Y. Wang, Opt. Lett., 2013, 38, 612–614 CrossRef CAS PubMed.
- S. L. Gai, C. X. Li, P. P. Yang and J. Lin, Chem. Rev., 2014, 114, 2343–2389 CrossRef CAS PubMed.
- G. C. Li, M. Lv, J. Dai and X. Li, Opt. Mater., 2015, 46, 40–44 CrossRef CAS.
- A. Khanna and P. S. Dutta, J. Solid State Chem., 2013, 198, 93–100 CrossRef CAS.
- P. Gupta, A. K. Bedyal, V. Kumar, Y. Khajuria, V. Kumar, E. Coetsee-Hugo, O. M. Ntwaeaborwa and H. C. Swart, Opt. Mater., 2014, 36, 996–1001 CrossRef CAS.
- D. den Engelsen, G. R. Fern, T. G. Ireland, P. G. Harris, P. R. Hobson, A. Lipman, R. Dhillon, P. J. Marsha and J. Silver, J. Mater. Chem. C, 2016, 4, 7026–7034 RSC.
- L. Zhu, J. Y. Li, Q. Li, X. D. Liu, J. Meng and X. Q. Cao, Nanotechnology, 2007, 18, 055604 CrossRef.
- W. X. Wang, Z. Y. Cheng, P. P. Yang, Z. Y. Hou, C. X. Li, G. G. Li, Y. L. Dai and J. Lin, Adv. Funct. Mater., 2011, 21, 456–463 CrossRef CAS.
- T. Liu, W. Xu, X. Bai and H. W. Song, J. Appl. Physiol., 2012, 111, 064312 CrossRef.
- K. Kömpe, H. Borchert, J. Storz, A. Lobo, S. Adam, T. Möller and M. Haase, Angew. Chem., Int. Ed. Engl., 2003, 42, 5513–5516 CrossRef PubMed.
- H. Wang, M. Yu, C. K. Lin and J. Lin, J. Colloid Interface Sci., 2006, 300, 176–182 CrossRef CAS PubMed.
- W. T. Gan, S. L. Xiao, L. K. Gao, R. A. Gao, J. Li and X. X. Zhan, ACS Sustainable Chem. Eng., 2017, 5, 3855–3862 CrossRef CAS.
- M. L. Chang and S. L. Tie, Nanotechnology, 2008, 19, 075711 CrossRef PubMed.
- Z. Wu, L. Lin, W. Yang, D. Zhang, C. Shen, W. Lou, H. Yin and K. Chang, RSC Adv., 2017, 7, 30963–30969 RSC.
- M. Jing, J. Wang, H. Hou, Y. Yang, Y. Zhang, C. Pan, J. Chen, Y. Zhu and X. Ji, J. Mater. Chem. A, 2015, 3, 16824–16830 CAS.
- L. C. Spangler, L. Lu, C. Kiely, B. W. Berger and S. McIntosh, J. Mater. Chem. A, 2016, 4, 6107–6115 CAS.
- M. Zeng, X. Peng, J. Liao, G. Wang, Y. Li, J. Li, Y. Qin, J. Wilson, A. song and S. Lin, Phys. Chem. Chem. Phys., 2016, 18(26), 17404 RSC.
- P. O. Anikeeva, J. E. Halpert, M. G. Bawendi and V. Bulović, Nano Lett., 2009, 9, 2532–2536 CrossRef CAS PubMed.
- B. A. Kairdolf, A. M. Smith, T. H. Stokes, M. D. Wang, A. N. Young and S. M. Nie, Annu. Rev. Anal. Chem., 2013, 6, 143–162 CrossRef CAS PubMed.
- J. Z. Wang, X. P. Zhou, H. B. Ma and G. H. Tao, Spectrochim. Acta, Part A, 2011, 81, 178–183 CrossRef CAS PubMed.
- S. Rong, P. Zhang, Y. Yang and F. Liu, RSC Adv., 2016, 6, 114632–114638 RSC.
- J. Zong, X. L. Yang, A. Trinchi, S. Hardin, I. Cole, Y. H. Zhu, C. Z. Li, T. Muster and G. Wei, Biosens. Bioelectron., 2014, 51, 330–335 CrossRef CAS PubMed.
- P. Jeevanandam, Y. Koltypin, O. Palchik and A. Gedanken, J. Mater. Chem., 2001, 11, 869–873 RSC.
- N. Venkatachalam, Y. Saito and K. Soga, J. Mater. Chem., 2001, 11, 869–873 RSC.
- X. Y. Shen and Y. C. Zhai, Rare Met., 2011, 30, 33–38 CrossRef CAS.
- Y. J. Liang, J. Ouyang, H. Y. Wang, W. L. Wang, P. F. Chui and K. N. Sun, Appl. Surf. Sci., 2012, 258, 3689–3694 CrossRef CAS.
- R. J. Gaboriaud, F. Pailloux, P. Guerin and F. Paumier, J. Phys. D: Appl. Phys., 2000, 33, 2884–2889 CrossRef CAS.
- H. Razavi-Khosroshahi, K. Edalati, H. Emami, E. Akiba, Z. Horita and M. Fuji, Inorg. Chem., 2017, 56, 2576–2580 CrossRef CAS PubMed.
- A. M. Kaczmarek, D. Ndagsi and R. Van Deun, Dalton Trans., 2016, 45, 16231–16239 RSC.
- A. F. Khan, D. Haranath, R. Yadav, S. Singh, S. Chawla and V. Dutta, Appl. Phys. Lett., 2008, 93, 073103 CrossRef.
- G. H. Pan, H. W. Song, X. Bai, Z. G. Liu, H. Q. Yu, W. H. Di, S. W. Li, L. B. Fan, X. G. Ren and S. Z. Lu, Chem. Mater., 2006, 18, 4526–4532 CrossRef CAS.
Footnote |
† Electronic supplementary information (ESI) available: SEM image, HR-TEM image and PL spectra of Y2O3:Eu3+@0.4YVO4:Eu3+; fluorescence response of pure Y2O3:Eu3+, pure YVO4:Eu3+, Y2O3:Eu3+ and 0.01YVO4:Eu3+, Y2O3:Eu3+@0.4YVO4:Eu3+ in the presence of increasing concentration of Cu2+; fluorescence response of rod-Y2O3:Eu3+@0.01YVO4:Eu3+ in the presence of increasing concentration of Cu2+; fluorescence response of rod-Y2O3:Eu3+@0.01YVO4:Eu3+–Cu2+ (10−8 mol L−1) in the presence of increasing concentration of glutamic acid and leucine. See DOI: 10.1039/c7ra08905h |
|
This journal is © The Royal Society of Chemistry 2017 |
Click here to see how this site uses Cookies. View our privacy policy here.