DOI:
10.1039/C7RA08760H
(Paper)
RSC Adv., 2017,
7, 46713-46720
A broad-band orange-yellow-emitting Lu2Mg2Al2Si2O12: Ce3+ phosphor for application in warm white light-emitting diodes
Received
8th August 2017
, Accepted 29th September 2017
First published on 3rd October 2017
Abstract
A new garnet-type Lu2Mg2Al2Si2O12 compound was successfully synthesized via high-temperature solid–state reaction. The crystal structure was confirmed by the Rietveld refinement method and belongs to a cubic system with a space group of Ia
d (230) and cell parameters of a = b = c = 11.88310(1) Å. There are two different crystallographic positions for Mg2+ with coordination numbers of eight and six, respectively. A relatively large band gap (4.83 eV) was estimated by the diffuse reflectance spectrum, indicating that Lu2Mg2Al2Si2O12 may be a promising host matrix for luminescent materials. The synthetic Ce3+-doped Lu2Mg2Al2Si2O12 phosphor shows intense and broad-band orange-yellow emission peaking at about 575 nm under near-ultraviolet (n-UV) and blue light excitation. Additionally, the quantum efficiency, thermal stability, and packing performance were investigated to evaluate the practical use in white LEDs. The results indicate that Lu2Mg2Al2Si2O12: Ce3+ may be a promising orange-yellow phosphor for warm white LEDs.
1. Introduction
White light-emitting diodes (w-LEDs) have recently received intensive attention because of their superior advantages, such as high efficiency, low power consumption, great stability, and eco-friendliness.1–3 Combination of the yellow-emitting phosphor Y3Al5O12: Ce3+ (YAG: Ce3+) with a blue InGaN chip is considered to be one of the most common and efficient ways to generate w-LEDs.4 However, this combination usually generates cool white light with a modest color-rendering index (CRI, Ra) of ∼75 due to the lack of red emission.5–8 Thus, there is a drive to develop new orange-yellow-emitting phosphors that show a broad-band emission with an intensified red component to obtain warm w-LEDs.9,10
As an important part of w-LEDs, phosphors are required to exhibit a high quantum efficiency and small thermal quenching. According to the work of Seshadri and co-workers, a large Debye temperature (ΘD) could be a predictor of high quantum efficiency (QE), and a wide band gap (Eg) of host is necessary to limit thermal quenching.11 Typically, the well-known yttrium aluminium garnet (YAG) matrix owns a large Debye temperature (726 K) and a wide band gap (6.43 eV); and correspondingly, YAG: Ce3+ phosphor shows a high QE (∼90) and small thermal quenching.11,12 Furthermore, Ce3+-doped compositional derivatives of YAG with these outstanding characteristics have also been reported and lead to a wide variety of applications, such as the commercial green Lu3Al5O12: Ce3+ and Ca3Sc2Si3O12: Ce3+ phosphors.13,14 Therefore, it is preferable for many researchers to focus on the garnet structure when searching for new phosphor hosts.
Garnet structure belongs to the cubic system (space group of Ia
d) with a general formula {C}3[A]2(D)3O12. Wherein, {C}, [A], and (D) represent the cations that occupy 24c, 16a, and 24d Wychoff positions and are surrounded by 8, 6, and 4 oxygen atoms to form dodecahedron, octahedron, and tetrahedron, respectively.15–17 The solid solution design for the host compound through cation/anion substitution, cosubstitution, or chemical-unit substitution is well employed for the photoluminescence tuning.18–20 Especially, garnet phosphors exhibit unique tunability of luminescence properties by chemical substitutions on the {C}, [A], and (D) cation sublattices.21,22 On that basis, a large amount of Ce3+-doped garnet hosts with various emission colors have been developed and reported, such as cyan Ca2LuHf2(AlO4)3: Ce3+ and Ca3Zr2SiGa2O12: Ce3+ phosphors, green CaLu2Al4SiO12: Ce3+ and Mg1.5Lu1.5Al3.5Si1.5O12: Ce3+ phosphors, yellow Y3MgxSixAl5−2xO12: Ce3+ and Lu3−xYxMgAl3SiO12: Ce3+ solid solution phosphors, and orange Lu2CaMg2(Si,Ge)3O12: Ce3+ phosphor.23–29 It is worth noticing that Mg2+ can not only be incorporated into [A] sites, such as Y3MgxSixAl5−2xO12: Ce3+, but also be introduced in {C} sites, such as Mg1.5Lu1.5Al3.5Si1.5O12: Ce3+. However, Mg2+–Si4+ incorporation into the [A] and (D) sites, respectively, leads to the red shift of Ce3+ emission, but Mg2+–Si4+ substitution on the {C} and (D) sites, respectively, can shift the Ce3+ emission to the short wavelength.26,27 The above results indicate that Mg2+ incorporation into dodecahedral or octahedral sites has totally different effects on the coordination environment for Ce3+. However, there is few research on the incorporation of Mg2+ into both {C} and [A] sites and its effect on luminescence properties.
Motivated by the above knowledge, we designed a new Lu2Mg2Al2Si2O12 composition based on the typical Lu3Al5O12 matrix, where a Lu3+ ({C} site) as well as an Al3+ ([A] site) are substituted by two Mg2+, and for charge neutrality two other Al3+ ((D) site) are simultaneously replaced by two Si4+. The Rietveld refinement results confirmed that Mg2+ ions were incorporated into both dodecahedral and octahedral sites as designed. And the luminescence properties of Ce3+-doped Lu2Mg2Al2Si2O12 were investigated systematically. The obtained Lu2Mg2Al2Si2O12: Ce3+ phosphor shows intense orange-yellow emission containing more red component than that of YAG: Ce3+ under blue light excitation. Furthermore, the temperature-dependent photoluminescence behaviours as well as the fabrication properties of the phosphor were studied to evaluate the applicability as a color converter for w-LEDs.
2. Experimental
2.1. Materials and synthesis
Polycrystalline samples of Lu2−xMg2Al2Si2O12: xCe3+ (x = 0, 0.02, 0.04, 0.06, 0.08, 0.10, 0.12) were synthesized by high-temperature solid–state reaction. The starting materials (Lu2O3, 99.99%; MgO, 99.95%; Al2O3, 99.99%; SiO2, 99.99%; CeO2, 99.995%) were all weighed out in the desired stoichiometry and thoroughly mixed. The mixtures were put in an alumina crucible and continually fired at 1400 °C in a reducing atmosphere of H2/N2 (3/1) for 6 h. Then the samples were furnace-cooled continuously to room temperature, and ground well for the subsequent measurements. The commercial YAG: Ce3+ phosphor used for comparison was manufactured by Grirem Advanced Materials Co., Ltd. The blue InGaN chips (λex = 455 nm) were purchased from Hang Zhou Silan Azure Co., Ltd.
2.2. Characterization methods
The phase structure determination of the as-prepared samples were carried out by a powder X-ray diffractometer (Rigaku, Japan) with Co-Kα radiation (λ = 0.178752 nm), and the date were recorded in the 2θ range of 10–80° with scan step of 6° min−1. The XRD data, for structural refinement, of Lu1.94Mg2Al2Si2O12: 0.06Ce3+ were collected on a powder X-ray diffractometer (PANalytical, Holland) with Cu-Kα radiation (λ = 0.154056 nm) in the 10–120° range (2θ) with scan speed of 0.0170° s−1. Diffuse reflection (DR) spectra were detected on an UV-Vis-NIR spectrophotometer (SHIMADZU, Japan) with an integrated sphere attachment, and BaSO4 powder sample served as a standard. The room-temperature photoluminescence (PL) and photoluminescence excitation (PLE) spectra, as well as the temperature-dependent photoluminescence properties, were measured by a Fluoromax-4 spectrofluorometer (Edison, USA) equipped with a 200 W xenon lamp, a photomultiplier tube operating, and a heating attachment. The fluorescent decay curves were measured on a TemPro-01 time-resolved fluorescence spectrofluorometer (Horbia Jobin Yvon, France). The quantum efficiency (QE) value was measured by an intensified multichannel QE-2100 spectrometer (Otsuka Electronics, Japan) equipped with a Xe lamp. The Commission International de I'Eclairage (CIE) chromaticity coordinates of samples were determined by a DT-101 colour analyser with a CCD detector (Laiko, Japan).
3. Results and discussion
3.1. Phase and crystal analysis
Fig. 1 presents the XRD patterns for the series of Lu2−xMg2Al2Si2O12: xCe3+ samples with different doped concentrations of Ce3+ (x = 0, 0.02, 0.04, 0.06, 0.08, 0.10, 0.12). The diffraction peaks of all these samples could be indexed to the Ia
d space group of the cubic system, and no diffraction peak of impurities was observed. It indicates that the single-phased Lu2Mg2Al2Si2O12 compound can be attained by the given synthesis conditions and the dopant concentration of Ce3+ in the designated range didn't incur any impurities or cause significant structure change in the host. Moreover, the diffraction peaks slightly shift to the low-angle side with the increasing Ce3+ concentration (as shown in the right panel of Fig. 1), meaning the expansion of the lattice caused by the substitution of Lu3+ (rVIII = 97.7 pm) by Ce3+ (rVIII = 114.3 pm).30
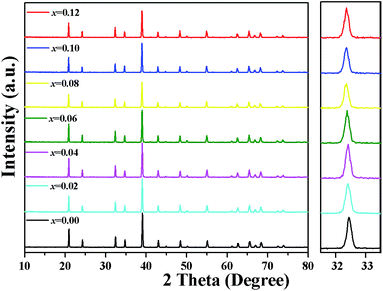 |
| Fig. 1 XRD patterns of Lu2−xMg2Al2Si2O12: xCe3+ samples with different Ce3+ (0 ≤ x ≤ 0.12). Right panel: the magnified patterns in the 2-theta range of 31.5–33.5°. | |
To confirm the phase structure and obtain detailed crystallographic information of the as-prepared samples, Rietveld structural refinement of XRD patterns for the selected Lu1.94Mg2Al2Si2O12: 0.06Ce3+ sample was performed (as shown in Fig. 2) by GSAS software package.31,32 In the process, the single-crystal structure data of Lu3Al5O12 (ICSD no. 23846) were used as an initial model. The refinement process was stably convergent to χ2 = 6.30, Rwp = 4.04%, Rp = 5.55%, which makes sure the formation of a single garnet phase. The final refined structure data and residual factors are listed in Table 1. Wherein, the unit cell parameters were a = b = c = 11.88310(1) Å and V = 1677.991(3) Å3.
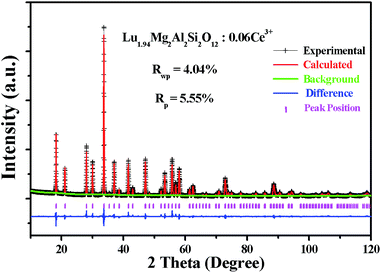 |
| Fig. 2 Rietveld refinement XRD patterns of the selected Lu1.94Mg2Al2Si2O12: 0.06Ce3+ sample. | |
Table 1 Main results of Rietveld refinement of the Lu1.94Mg2Al2Si2O12: 0.06Ce3+ sample
Crystal system |
cubic |
Space group |
Ia d |
a = b = c (Å) |
11.88310(1) |
α = β = γ (deg) |
90 |
V (Å3) |
1677.991(3) |
Z |
8 |
Rwp (%) |
4.04 |
Rp (%) |
5.55 |
χ2 |
6.30 |
Atom |
Site |
Occu. |
x |
y |
z |
Lu |
24c |
0.6467 |
0.12500 |
0.000000 |
0.25000 |
Ce |
24c |
0.02000 |
0.12500 |
0.000000 |
0.25000 |
Mg1 |
24c |
0.3333 |
0.12500 |
0.000000 |
0.25000 |
Mg2 |
16a |
0.5000 |
0.00000 |
0.000000 |
0.00000 |
Al1 |
16a |
0.5000 |
0.00000 |
0.000000 |
0.00000 |
Al2 |
24d |
0.3333 |
0.37500 |
0.000000 |
0.25000 |
Si |
24d |
0.6667 |
0.37500 |
0.000000 |
0.25000 |
O |
96h |
1.0000 |
−0.03863 |
0.052202 |
0.15270 |
From the refinement results, Fig. 3(a) displays a schematic diagram of the Lu1.94Mg2Al2Si2O12: 0.06Ce3+ garnet structure. The dodecahedral, octahedral, and tetrahedral sites are randomly occupied by Lu/Mg/Ce (1.94/1/0.06), Mg/Al (1/1) and Al/Si (1/2), coordinated by eight, six and four oxygen ions, respectively. In addition, the [(Lu/Mg/Ce)O8] dodecahedron connects with neighbour [(Mg/Al)O6] octahedrons or other dodecahedrons by sharing edge, but connects with part of neighbour [(Al/Si)O4] tetrahedrons by sharing edge and the other by sharing one O point, as shown in Fig. 3(b). Actually, each [(Lu/Mg/Ce)O8] dodecahedron is surrounded by four [(Lu/Mg/Ce)O8] dodecahedrons, four [(Mg/Al)O6] octahedrons and six [(Al/Si)O4] tetrahedrons, indicating that the photoluminescence of Ce3+ would be affected by the floating ratio of Lu/Mg/Ce, Mg/Al and Al/Si in surrounding dodecahedrons, octahedrons and tetrahedrons, respectively.
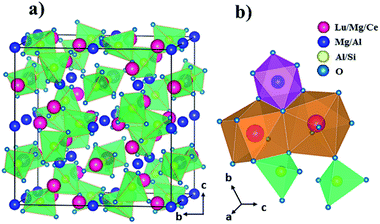 |
| Fig. 3 (a) Structure of Lu1.94Mg2Al2Si2O12: 0.06Ce3+ along a axis and (b) the connection between polyhedrons. | |
3.2. Photoluminescence properties
Fig. 4 depicts the diffuse reflectance (DR) spectra of the as-prepared Lu2Mg2Al2Si2O12 host and Lu1.92Mg2Al2Si2O12: 0.08Ce3+ phosphor. For the Lu2Mg2Al2Si2O12 host, the DR spectrum shows an intense reflection in the visible range (380–780 nm), and a drastic decrease in the ultraviolet range (200–350 nm) originating from the valence-to-conduction transitions. The band gap value (Eg) of the Lu2Mg2Al2Si2O12 host can be estimated by the following equation:33,34 |
[F(R∞)hν]n = A(hν − Eg)
| (1) |
where hν and A refer to the photon energy and a proportional constant, respectively; R∞ = Rsample/Rstandard; n = 1/2 for an indirect transition or 2 for a direct transition; and F(R∞) is the remission (Kubelka–Munk) function, defined as35 |
F(R∞) = K/S = (1 − R∞)2/2R∞
| (2) |
where K, and S represent the absorption and scattering coefficient, respectively. Through the linear extrapolation of [F(R∞)hν]n = 0 (as shown in the inset of Fig. 4), the Eg value was determined to be about 4.83 eV. When 8 mol% of Ce3+ was doped into the Lu2Mg2Al2Si2O12 host lattice, the DR spectrum exhibits enhanced absorption and two broad valleys (300–350 nm and 400–500 nm), which is attributable to the 4f–5d transitions of Ce3+ and consistent with the excitation spectrum.
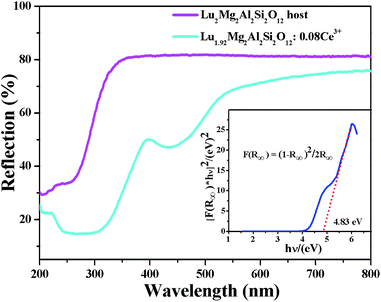 |
| Fig. 4 Diffuse reflectance spectra of Lu2Mg2Al2Si2O12 host and Lu1.92Mg2Al2Si2O12: 0.08Ce3+ phosphor. Inset: the band gap calculation of Lu2Mg2Al2Si2O12 host. | |
Fig. 5 shows the room-temperature photoluminescence excitation (PLE) and emission (PL) spectra of Lu1.92Mg2Al2Si2O12: 0.08Ce3+. The PLE spectrum (monitored at 575 nm), ranging from 300–540 nm, possesses a strong excitation band (about 370–540 nm) peaking at about 436 nm and a weak excitation band (about 300–360 nm) peaking at about 325 nm, corresponding to transitions of Ce3+ from the ground state (4f) to the two lowest excited levels (5d1 and 5d2, respectively). As also shown in Fig. 5, the PL spectrum of Lu1.92Mg2Al2Si2O12: 0.08Ce3+ under 436 nm excitation exhibits an asymmetric broad band (about 480–750 nm) peaking at 575 nm assigned to the 5d–4f transitions of Ce3+.36,37 The full width at half maximum (FWHM) of Lu1.92Mg2Al2Si2O12: 0.08Ce3+ is calculated to be about 144 nm. Moreover, the asymmetric emission band can be resolved into two Gaussian profiles with peaks centered at 554 nm and 614 nm, respectively, which are attributed to the transitions from the lowest 5d excited state to the 2F5/2 and 2F7/2 ground states.38,39 And the energy difference Δk between these two bands is about 1764 cm−1, which is close to the theoretical energy difference between the 2F5/2 and 2F7/2 levels (∼2000 cm−1).40,41
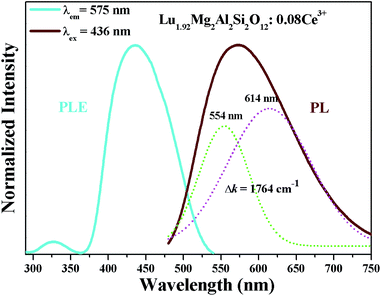 |
| Fig. 5 PLE (λem = 575 nm) spectrum and PL (λex = 436 nm) spectrum of Lu1.92Mg2Al2Si2O12: 0.08Ce3+. | |
In order to optimize the doping concentration of Ce3+ ion for this new garnet phosphor, the PL (λex = 436 nm) spectra of Lu2−xMg2Al2Si2O12: xCe3+ samples with different concentrations of Ce3+ (x = 0.02, 0.04, 0.06, 0.08, 0.1, 0.12) were measured as shown in Fig. 6. The emission intensity increases with Ce3+ concentration increasing at first, reaches a maximum when x = 0.08, and then declines when Ce3+ concentration exceeds this critical concentration (also see the inset of Fig. 6) on account of concentration quenching effect.42 The concentration quenching mainly results from non-radiative energy transfer among Ce3+ ions, whose occurring possibility increases as the content of Ce3+ increases. Three main mechanisms responsible for non-radiative energy transfer are exchange interaction, radiation reabsorption and multipolar interaction, respectively.43,44 To make clear which mechanism dominates, it is requisite to obtain the critical distance (Rc) of energy transfer. The critical distance (Rc) among Ce3+ ions can be estimated based on the following formula:45,46
|
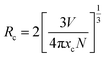 | (3) |
where
V,
xc, and
N refer to the unit cell volume, the critical concentration of activator, and the number of crystallographic sites in the unit cell that can be occupied by activator ions, respectively. In this case, the values
V = 1677.991(28) Å
3,
xc = 0.08, and
N = 24, and the obtained
Rc value is 11.86. It's less likely that the exchange interaction mechanism plays the dominant role for the non-radiative energy transfer, because this mechanism generally requires a forbidden transition (the
Rc value is ∼5 Å).
47 Besides, the radiation reabsorption mechanism has an obvious effect only when there is a considerable overlap between the excitation and emission spectra. Thus, it is deduced that the electric multipolar interaction should take the main responsibility for non-radiative energy transfer between activator ions for Lu
2Mg
2Al
2Si
2O
12: Ce
3+ phosphor.
 |
| Fig. 6 PL spectra of Lu2−xMg2Al2Si2O12: xCe3+ (0.02 ≤ x ≤ 0.12). Insert: the dependence of emission intensity and peak wavelength on Ce3+ concentration. | |
Furthermore, the inset of Fig. 6 also depicts the dependence of peak wavelength on Ce3+ dopant concentration. It can be found that the peak wavelength of emission spectra gradually red-shifts from 561 nm for x = 0.02 to 580 nm for x = 0.12. The redshift could be ascribed to the following two factors. First, the possibility of energy transfer from the Ce3+ ions at higher-energy sublevels of 5d to those at lower-energy sublevels increases with the increasing Ce3+ ion content, reducing the probability of the higher-energy emission. Second, as the substitution for smaller Lu3+ ions by larger Ce3+ ions increases, the crystal field strength surrounding Ce3+ ions enlarges, which causes a larger splitting of 5d levels and thus decreases the emission energy from the lowest excited level (5d1) to the ground state (4f) of Ce3+.23 For these two factors, it is observed that the emission band of Lu2Mg2Al2Si2O12: Ce3+ gradually red-shifts as the Ce3+ ion content increases.
Fig. 7 depicts the normalized PL spectra of the orange-yellow-emitting Lu1.92Mg2Al2Si2O12: 0.08Ce3+ and the commercial yellow-emitting YAG: Ce3+ under 450 nm excitation. It is observed that the orange-yellow-emitting Lu1.92Mg2Al2Si2O12: 0.08Ce3+ phosphor prepared by us contains more red-component emission than the commercial YAG: Ce3+ phosphor. In addition, the bandwidth of Lu1.92Mg2Al2Si2O12: 0.08Ce3+ (FWHM = 144 nm) is wider than that of YAG: Ce3+ (FWHM = 124 nm). The results indicate that higher-quality white light obtained by coupling 455 nm blue InGaN chips with the orange-yellow-emitting Lu1.92Mg2Al2Si2O12: 0.08Ce3+ phosphor is expected. In addition, the particularly broad emission band of Lu1.92Mg2Al2Si2O12: 0.08Ce3+ phosphor may be due to the diverse local distortions of Ce3+. Since the dodecahedral, octahedral and tetrahedral sites near Ce3+ ions are occupied by Lu3+/Mg2+, Mg2+/Al3+ and Al3+/Si4+, respectively, the different distribution ratio of cation ions around Ce3+ results in the site-to-site variation of local crystalline environment and accordingly inhomogeneous broadening of the Ce3+ emission spectra.48
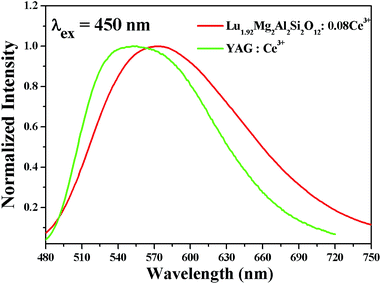 |
| Fig. 7 Normalized PL spectra of Lu1.92Mg2Al2Si2O12: 0.08Ce3+ and YAG: Ce3+. | |
Fig. 8 shows the fluorescence decay curves of Lu2−xMg2Al2Si2O12: xCe3+ phosphors with varying Ce3+ contents (x = 0.02, 0.04, 0.06, 0.08, 0.1, 0.12) excited at 436 nm and monitored at 570 nm. It was found that these decay curves had parallel decay behaviours and all the curves can be fitted well by the mono-exponential function:49
|
I(t) = A1 exp(−t/τ)
| (4) |
where
I(
t) and
A1 represent the photoluminescence intensity at time
t and the initial intensity immediately after the exciting pulse, respectively, and
t refers to fluorescence lifetime. And the decay times were determined by the fitting to be 66.8, 65.6, 64.4, 63.9, 62.9 and 62.2 ns for Lu
2−xMg
2Al
2Si
2O
12:
xCe
3+ (
x = 0.02, 0.04, 0.06, 0.08, 0.1, 0.12) phosphors, respectively. As Ce
3+ concentration increases, both the possibility of energy transfer from Ce
3+ ions to quenching sites and the energy transfer rate between Ce
3+ ions increase, resulting in the decrease of the lifetime.
50 In addition, the well-fitting results by mono-exponential decay illustrate that Ce
3+ ions only occupy one crystallographic site (24c) in the Lu
2Mg
2Al
2Si
2O
12 host in accordance with the Rietveld refinement result of Lu
1.94Mg
2Al
2Si
2O
12: 0.06Ce
3+.
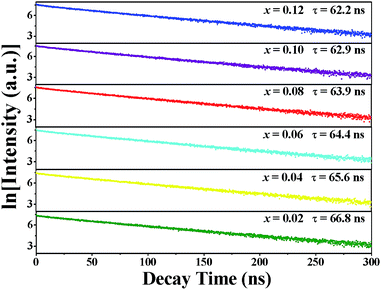 |
| Fig. 8 Decay curves and calculated lifetime values of Lu2−xMg2Al2Si2O12: xCe3+ phosphors with different Ce3+ concentrations (0.02 ≤ x ≤ 0.12). | |
The temperature-dependent performance of phosphor is one of the significant technological parameters, which has a great effect on the luminous efficacy and color rendering performance of the w-LEDs, especially for phosphor-converted w-LEDs based on blue chips. The temperature-dependent emission spectra of Lu1.92Mg2Al2Si2O12: 0.08Ce3+ phosphor under 436 nm excitation are presented in Fig. 8. As temperature rises from 25 to 200 °C, the emission intensity gradually declines due to the thermal quenching. All the spectral shape keeps similar with a weak blue shift (575 nm at 25 °C vs. 571 nm at 200 °C). The blue shift can be ascribed to the thermally activated phonon-assisted excitation from a lower-energy sublevel to a higher-energy sublevel of Ce3+ in the excited state.51 The variation of emission intensity with temperature is also shown in the inset of Fig. 9. When the temperature reaches 150 °C, the emission intensity of Lu1.92Mg2Al2Si2O12: 0.08Ce3+ phosphor drops to 74.9% of its initial intensity, indicating that this phosphor could exhibit excellent performance in the operation temperature range of w-LEDs. In case of the thermal quenching process only generated by the nonradiative relaxation process, the activation energy (barrier energy for thermal quenching) can be calculated by the Arrhenius equation:52,53
|
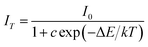 | (5) |
where
I0 is the initial emission intensity;
IT represents the emission intensity at different testing temperatures;
c is a constant; Δ
E is the activation energy of thermal quenching; and
k is the Boltzmann constant (8.629 × 10
−5 eV K
−1). By linear fitting the relationship of ln[(
I0/
IT) − 1]
vs. 1000/
T with a slope of −4.075 got, the activation energy was calculated to be 0.351 eV (as shown in
Fig. 10). The relatively high activation energy of this phosphor also illustrates its excellent thermal properties.
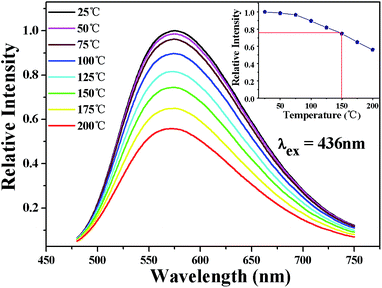 |
| Fig. 9 Temperature-dependent PL (λex = 436 nm) spectra of Lu1.92Mg2Al2Si2O12: 0.08Ce3+ phosphor. Insert: the relative emission intensity of Lu1.92Mg2Al2Si2O12: 0.08Ce3+ phosphor varying with temperature. | |
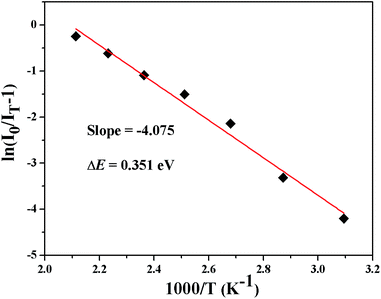 |
| Fig. 10 Arrhenius fitting of the PL intensity of Lu1.92Mg2Al2Si2O12: 0.08Ce3+ phosphor as a function of temperature. | |
3.3. CIE chromaticity coordinates, quantum efficiency, and w-LED device performance
The CIE chromaticity coordinates of Lu2−xMg2Al2Si2O12: xCe3+ with different Ce3+ doped concentrations (0.02 ≤ x ≤ 0.12) under 436 nm excitation are depicted in Fig. 11. The color coordinates are shown as ranging from (0.458, 0.516) for x = 0.02 to (0.493, 0.493) for x = 0.12, which means that these phosphors may serve as orange-yellow-emitting phosphors for w-LEDs applications. Besides, the image of Lu1.92Mg2Al2Si2O12: 0.08Ce3+ phosphor under blue light radiating is also presented in the inset of Fig. 11, and intense orange-yellow light can be clearly caught by the naked eye. Furthermore, the internal and external QE of the optimal Lu1.92Mg2Al2Si2O12: 0.08Ce3+ phosphor under 436 nm excitation are measured to be about 72.6% and 53.3%, respectively. Generally, the QE value could be further improved by optimizing the preparation conditions.
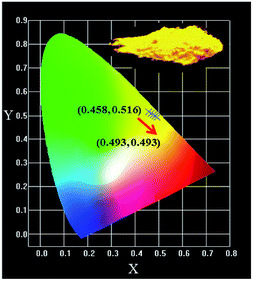 |
| Fig. 11 The CIE coordinates of Lu2−xMg2Al2Si2O12: xCe3+ (0.02 ≤ x ≤ 0.12) and the image of Lu1.92Mg2Al2Si2O12: 0.08Ce3+ phosphor under blue light lamp. | |
The Lu2Mg2Al2Si2O12: Ce3+ phosphor is great appropriate as a color converter for blue chip w-LEDs due to its strong blue light absorption and orange-yellow light emission. To further demonstrate the potential of Lu2Mg2Al2Si2O12: Ce3+ phosphor for pc-wLEDs application, the Lu1.92Mg2Al2Si2O12: 0.08Ce3+ phosphor and a blue InGaN chip (λex = 455 nm) were used to fabricate a w-LED device. Fig. 12 presents the electroluminescence (EL) spectrum of the as-fabricated w-LED device under 50 mA current operating. The device emits warm bright light with the CIE chromaticity coordinates of (0.3485, 0.3216) and relatively low correlated colour temperature (CCT) of 4718 K. And the colour rendering index (Ra) of this w-LED lamp was checked out to be 84.5, which is higher than that of the w-LEDs using blue chips pumped by YAG: Ce3+ phosphor (Ra = 75).54 Consequently, Lu2Mg2Al2Si2O12: Ce3+ displays significant potential as a w-LED phosphor, achieving high Ra and low CCT without blending additional red phosphor.
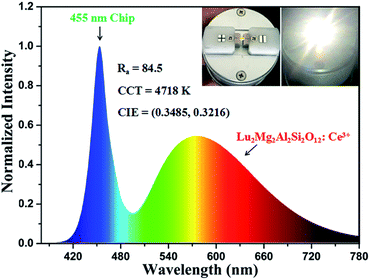 |
| Fig. 12 Electroluminescence spectrum of a w-LED consisting of a blue InGaN chip and the Lu1.92Mg2Al2Si2O12: 0.08Ce3+ phosphor. Insert: as-prepared w-LED and w-LED in operation. | |
4. Conclusions
In summary, a new phosphor Lu2Mg2Al2Si2O12: Ce3+ has been successfully synthesized via the high-temperature solid–state reaction method. Rietveld structural refinement results make sure the formation of a single garnet phase. Under excitation with n-UV to blue light, the Lu2Mg2Al2Si2O12: Ce3+ phosphor exhibited intense broad-band orange-yellow emission (peaking at about 575 nm, FWHM = 144 nm) containing more red component than that of YAG: Ce3+. The optimal dopant concentration of Ce3+ was x = 0.08, of which the internal quantum efficiency was about 72.6%. The corresponding concentration quenching mechanism is considered to be the electric multipolar interaction. When heated up to 150 °C, the Lu1.92Mg2Al2Si2O12: 0.08Ce3+ phosphor still remained at about 75% of the emission intensity at room temperature. The w-LED device fabricated with a blue chip and the Lu2Mg2Al2Si2O12: Ce3+ phosphor displayed the CIE color coordinate of (0.3485, 0.3216), CCT of 4718 K, and Ra value of 84.5. Those results indicate that Lu2Mg2Al2Si2O12: Ce3+ may be a promising candidate for applications in n-UV and blue light excited warm white LEDs.
Conflicts of interest
There are no conflicts to declare.
Acknowledgements
This work was financially supported by the National Basic Research Program of China (2014CB643801).
References
- S. Nakamura, T. Mukai and M. Senoh, Appl. Phys. Lett., 1994, 64, 1687–1689 CrossRef CAS.
- S. Pimputkar, J. S. Speck, S. P. DenBaars and S. Nakamura, Nat. Photonics, 2009, 3, 180–182 CrossRef CAS.
- P. Pust, V. Weiler, C. Hecht, A. Tücks, A. S. Wochnik, A. K. Henß, D. Wiechert, C. Scheu, P. J. Schmidt and W. Schnick, Nat. Mater., 2014, 13, 891–896 CrossRef CAS PubMed.
- Y. Jia, R. Pang, H. Li, W. Sun, J. Fu, L. Jiang, S. Zhang, Q. Su, C. Li and R. S. Liu, Dalton Trans., 2015, 44, 11399–11407 RSC.
- C. H. Huang and T. M. Chen, Inorg. Chem., 2011, 50, 5725–5730 CrossRef CAS PubMed.
- L. Y. Feng, Z. D. Hao, X. Zhang, L. L. Zhang, G. H. Pan, Y. S. Luo, L. G. Zhang, H. F. Zhao and J. H. Zhang, Dalton Trans., 2016, 45, 1539–1545 RSC.
- F. Du, W. D. Zhuang, R. H. Liu, Y. H. Liu, J. Y. Zhong, W. Gao, K. Chen, L. Chen, K. Kato and K. Lin, RSC Adv., 2016, 6, 77059–77065 RSC.
- N. Guo, H. P. You, Y. H. Song, M. Yang, K. Liu, Y. H. Zheng, Y. J. Huang and H. J. Zhang, J. Mater. Chem., 2010, 20, 9061–9067 RSC.
- W. Z. Sun, Y. L. Jia, R. Pang, H. F. Li, T. F. Ma, D. Li, J. P. Fu, S. Zhang, L. H. Jiang and C. Y. Li, ACS Appl. Mater. Interfaces, 2015, 7, 25219–25226 CAS.
- L. L. Zhang, J. H. Zhang, X. Zhang, Z. D. Hao, H. F. Zhao and Y. S. Luo, ACS Appl. Mater. Interfaces, 2013, 5, 12839–12846 CAS.
- J. Brgoch, S. P. DenBaars and R. Seshadri, J. Phys. Chem. C, 2013, 117, 17955–17959 CAS.
- V. Bachmann, C. Ronda and A. Meijerink, Chem. Mater., 2009, 21, 2077–2084 CrossRef CAS.
- A. A. Setlur and A. Srivastava, Opt. Mater., 2007, 29, 1647–1652 CrossRef CAS.
- Y. Shimomura, T. Honma, M. Shigeiwa, T. Akai, K. Okamoto and N. Kijima, J. Electrochem. Soc., 2007, 154, J35–J38 CrossRef CAS.
- Y. R. Shi, G. Zhu, M. Mikami, Y. Shimomura and Y. H. Wang, Dalton Trans., 2015, 44, 1775–1781 RSC.
- X. H. Gong, J. H. Huang, Y. J. Chen, Y. F. Lin, Z. D. Luo and Y. D. Huang, Inorg. Chem., 2014, 53, 6607–6614 CrossRef CAS PubMed.
- S. K. Hussain and J. S. Yu, RSC Adv., 2017, 7, 13281–13288 RSC.
- M. M. Shang, S. S. Liang, N. R. Qu, H. Z. Lian and J. Lin, Chem. Mater., 2017, 29, 1813–1829 CrossRef CAS.
- Z. G. Xia and K. R. Poeppelmeier, Acc. Chem. Res., 2017, 50, 1222–1230 CrossRef CAS PubMed.
- Z. G. Xia, C. G. Ma, M. S. Molokeev, Q. L. Liu, K. Rickert and K. R. Poeppelmeier, J. Am. Chem. Soc., 2015, 137, 12494–12497 CrossRef CAS PubMed.
- Z. G. Xia and A. Meijerink, Chem. Soc. Rev., 2017, 46, 275–299 RSC.
- Z. G. Xia and Q. L. Liu, Prog. Mater. Sci., 2016, 84, 59–117 CrossRef CAS.
- X. C. Wang, Z. Y. Zhao, Q. S. Wu, Y. Y. Li and Y. H. Wang, J. Mater. Chem. C, 2016, 4, 11396–11403 RSC.
- J. Y. Zhong, W. D. Zhuang, X. R. Xing, R. H. Liu, Y. F. Li, Y. L. Zheng, Y. S. Hu and H. B. Xu, RSC Adv., 2015, 6, 2155–2161 RSC.
- A. Katelnikovas, J. Plewa, D. Dutczak, S. Möller, D. Enseling, H. Winkler, A. Kareiva and T. Jüstel, Opt. Mater., 2012, 34, 1195–1201 CrossRef CAS.
- Y. C. Jia, Y. J. Huang, N. Guo, H. Qiao, Y. H. Zheng, W. Z. Lv, Q. Zhao and H. P. You, RSC Adv., 2012, 2, 2678–2681 RSC.
- M. M. Shang, J. Fan, H. Z. Lian, Y. Zhang, D. L. Geng and J. Lin, Inorg. Chem., 2014, 53, 7748–7755 CrossRef CAS PubMed.
- H. P. Ji, L. Wang, M. S. Molokeev, N. Hirosaki, Z. H. Huang, Z. G. Xia, O. M. ten Kate, L. H. Liu and R. J. Xie, J. Mater. Chem. C, 2016, 4, 2359–2366 RSC.
- A. A. Setlur, W. J. Heward, Y. Gao, A. M. Srivastava, R. G. Chandran and M. V. Shankar, Chem. Mater., 2006, 18, 3314–3322 CrossRef CAS.
- R. D. Shannon and C. T. Prewitt, Acta Crystallogr., 1969, 25, 925–946 CrossRef CAS.
- H. M. Rietveld, J. Appl. Crystallogr., 1969, 2, 65–71 CrossRef CAS.
- B. H. Toby, J. Appl. Crystallogr., 2001, 34, 210–213 CrossRef CAS.
- A. E. Morales, E. S. Mora and U. Pal, Rev. Mex. Fis. S, 2007, 53, 18–22 CAS.
- Y. J. Hua, X. J. Li, D. W. Zhang, H. P. Ma, D. G. Deng and S. Q. Xu, New J. Chem., 2016, 40, 5458–5466 RSC.
- Z. J. Zhang, O. M. ten Kate, A. C. A. Delsing, Z. Y. Man, R. J. Xie, Y. F. Shen, M. J. H. Stevens, P. H. L. Notten, P. Dorenbos, J. T. Zhao and H. T. Hintzen, J. Mater. Chem. C, 2013, 1, 7856–7865 RSC.
- X. Ding, G. Zhu, W. Y. Geng, Q. Wang and Y. H. Wang, CrystEngComm, 2015, 17, 3235–3242 RSC.
- Y. L. Zheng, W. D. Zhuang, X. R. Xing, J. Y. Zhong, R. H. Liu, Y. F. Li, Y. H. Liu and Y. S. Hu, RSC Adv., 2016, 6, 68852–68859 RSC.
- W.-R. Liu, C.-H. Huang, C.-P. Wu, Y.-C. Chiu, Y.-T. Yeh and T.-M. Chen, J. Mater. Chem., 2011, 21, 6869–6874 RSC.
- R. J. Yu, S. L. Zhong, N. Xue, H. J. Li and H. L. Ma, Dalton Trans., 2014, 43, 10969–10976 RSC.
- G. Blasse and B. C. Grabmaier, Luminescent Materials, Springer, Berlin, German, 1994, p. 45 Search PubMed.
- Y. Q. Li, N. Hirosaki, R. J. Xie, T. Takeda and M. Mitomo, Chem. Mater., 2008, 20, 6704–6714 CrossRef CAS.
- W. B. Im, S. Brinkley, J. Hu, A. Mikhailovsky, S. P. DenBaars and R. Seshadri, Chem. Mater., 2010, 22, 2842–2849 CrossRef CAS.
- S.-P. Lee, T.-S. Chan and T.-M. Chen, ACS Appl. Mater. Interfaces, 2015, 7, 40–44 CAS.
- J. Y. Zhong, W. D. Zhuang, X. R. Xing, R. H. Liu, Y. F. Li, Y. H. Liu and Y. S. Hu, J. Phys. Chem. C, 2015, 119, 5562–5569 CAS.
- G. Blasse, Phys. Lett. A, 1968, 28, 444–445 CrossRef CAS.
- G. Blasse, Philips Res. Rep., 1969, 24, 131 CAS.
- Z. W. Zhang, L. J. Wang, S. S. Yang, W. G. Chen and X. J. Chu, Dyes Pigm., 2017, 142, 272–276 CrossRef CAS.
- N. M. Khaidukov, V. N. Makhov, Q. H. Zhang, R. Shi and H. B. Liang, Dyes Pigm., 2017, 142, 524–529 CrossRef CAS.
- X. Chen, Z. G. Xia and Q. L. Liu, Dalton Trans., 2014, 43, 13370–13376 RSC.
- S.-P. Lee, C.-H. Huang, T.-S. Chan and T.-M. Chen, ACS Appl. Mater. Interfaces, 2014, 6, 7260–7267 CAS.
- Z. G. Xia, X. M. Wang, Y. X. Wang, L. B. Liao and X. P. Jing, Inorg. Chem., 2011, 50, 10134–10142 CrossRef CAS PubMed.
- L. Chen, R. H. Liu, W. D. Zhuang, Y. H. Liu, Y. S. Hu, X. F. Zhou, W. Gao and X. L. Ma, CrystEngComm, 2015, 17, 3687–3694 RSC.
- G. Li, Y. Fan, H. J. Guo and Y. H. Wang, New J. Chem., 2017, 41, 5565–5571 RSC.
- N. Komuro, M. Mikami, Y. Shimomura, E. G. Bithell and A. K. Cheetham, J. Mater. Chem. C, 2014, 2, 6084–6089 RSC.
|
This journal is © The Royal Society of Chemistry 2017 |