DOI:
10.1039/C7RA07775K
(Paper)
RSC Adv., 2017,
7, 42919-42928
Ag modified g-C3N4 composite entrapped PES UF membrane with visible-light-driven photocatalytic antifouling performance
Received
15th July 2017
, Accepted 30th August 2017
First published on 5th September 2017
Abstract
Membrane fouling is still the main obstacle for the wider application of membrane processes. In this study, different amounts of silver modified graphitic carbon nitride (Ag/g-C3N4) were firstly introduced into polyethersulfone (PES) membranes by blending via a phase-inversion method. The impacts of Ag/g-C3N4 addition on the membrane morphology, filtration and photocatalysis antifouling properties of Ag/g-C3N4/PES nanocomposite membranes were systematically studied. The results illustrated that the addition of Ag/g-C3N4 could make the finger-like voids in Ag/g-C3N4 nanocomposite membrane longer and wider, increasing the hydrophilicity and filtration property of the nanocomposite membrane. Also the addition of Ag/g-C3N4 nanosheets could endow the nanocomposite membranes with excellent antibacterial, photocatalytic dye degradation and antifouling properties under visible light irradiation.
1. Introduction
Membrane filtration technology has been widely used in many fields due to its reliability and ease of operation. However, membrane fouling remains the main obstacle which strongly restricts its application.1 According to different pollutants, membrane fouling can be classified into organic fouling, inorganic fouling and biofouling. No matter what kind of fouling occurs, it will result in a significant decline in filtration performance, increased maintenance costs and shortened membrane life.2 Therefore, many efforts have been made to address this problem.
With the development of nanotechnology, various of nanomaterials have been introduced into polymeric matrices to endow the resulting nanocomposite membranes with desired properties.2 Silver nanoparticles (AgNPs) have been widely used to modify various of membrane materials by blending or in situ reduction, because of their excellent antibacterial property.3–5 The results showed that the silver based nanocomposite membranes exhibited significant antibacterial and antibiofouling performances, mainly due to the release of silver ions (Ag+).
Nano titanium dioxide (TiO2) as a traditional photocatalyst also has been widely incorporated into various polymeric materials.6–8 Many reports indicated that the introduction of TiO2 could significantly increase the surface hydrophilicity, and improve the ability to degrade organic pollutants by photocatalytic processes.9,10 Therefore the anti-organic fouling property can be enhanced. In addition to these merits, the TiO2 based nanocomposite membrane still faces the challenge of poor photocatalytic performance under visible light irradiation. Besides, most of the current nanomaterial modification strategies are focused on single type of membrane fouling. However, the membrane fouling in the actual process is often very complex and made up of different kinds of membrane fouling. Thus, using a new nanomaterial to address the comprehensive membrane fouling problem has become an urgent need.
Graphitic carbon nitride (g-C3N4), which has similar stacked 2D layered structure to graphene, exhibits excellent visible light photocatalysis, chemical and thermal stability.11,12 It has become the research hot spot in the fields of photocatalysis and environmental purification because of the outstanding photocatalytic property, low cost and simple preparation process. However, the photocatalytic ability of g-C3N4 still faces challenge due to the recombination of photo-generated charge carriers.13,14 In order to remedy this issue, many methods have been taken to modify the pure g-C3N4. Among various strategies, noble metal doping as a simple method attracts more attention. Silver nanoparticles (AgNPs) as a popular noble metal were widely used to fabricate Ag/g-C3N4 nanocomposite by photodeposition.12,15,16 Many researchers found the similar phenomenon that the modification of silver nanoparticles could effectively enhanced the photocatalytic activity of g-C3N4 due to the improved charge separation efficiency.12,14
At present, the modification and application of g-C3N4 based materials have been well studied in the field of photocatalysis, but there are few reports in the preparation of organic antifouling membrane materials. Recently, Zhao et al. successfully introduced g-C3N4/reduced graphene oxide (RGO) on the surface of cellulose acetate (CA) membrane by vacuum filtration.17 They found that the addition of g-C3N4/RGO could enhance the degradation ability of organic pollutants and bacterial removal rate of CA composite microfiltration membrane. However, membrane surface modification may araise problems during long time filtration such as the release of particles because of the poor adhesion between photocatalyst and membrane substrate.18 Alternatively, blending modification is an attractive method due to its facile preparation and stable performance.
As mentioned above, the advantages of Ag/g-C3N4 nanocomposite materials can be summarized as below. First of all, it combines the excellent antibacterial activity of AgNPs and the photocatalytic property of g-C3N4 together. Secondly, the doping of AgNPs by photo-reduction not only can increase the photocatalytic activity of g-C3N4 but also may facilitate the well disperse of AgNPs on the support and decrease the aggregation. Therefore, we considered that the Ag/g-C3N4 composite may be a good alternative that can be used to improve the comprehensive antifouling performance of polymeric membranes.
Thus, in this work Ag/g-C3N4 composite was firstly introduced into polyethersulfone (PES) membranes by blending. The effect of Ag/g-C3N4 composite on membrane morphology, filtration and antibacterial property was studied. Also the photocatalytic performance of nanocomposite membranes was tested by the dye degradation experiment. Furthermore, the influence of inorganic additive on the fouling mitigation capacity of polyethersulfone (PES) membranes under visible light illumination was examined based on the bovine serum albumin (BSA) filtration and by the calculation of flux recovery ratio (FRR) and fouling resistances.
2. Experimental
2.1 Materials
PES (Ultrason E6020P) was purchased from BASF. Melamine (C3H6N6, Analytical grade), silver nitrate (AgNO3), sulfuric acid (H2SO4, analytical grade) and nitric acid (HNO3, analytical grade), N,N-dimethylformamide (DMF) was obtained from Sinopharm Chemical Reagent Co., Ltd. Bovine serum albumin (BSA, 69 kDa), egg albumin (45 kDa), pepsin (35 kDa) were purchased from Sigma Aldrich.
2.2 Preparation and characterization of photocatalyst
In a typical synthesis, 5 g of melamine was heated at 500 °C in a muffle furnace for 2 h and following elevated to the 520 °C with a step of 10 °C min−1 for another 2 h for the further reaction. The resultant yellow powder were first dispersed in the mixture of nitric acid (65%) and sulfuric acid (98%) with the volume ratio of 1
:
3, then the turbid liquid was magnetic stirred overnight, the resultant powder was washed by deionized water completely until the neutral and following baked in the oven overnight to get the g-C3N4 nanosheets.
The Ag/g-C3N4 composite photocatalysts were fabricated by photodeposition as described elsewhere.11,12 Generally, 0.1 g g-C3N4 nanosheets and certain amount of AgNO3 (0.1 mol L−1, 5 mL) was dispersed in 100 mL deionized water with stirring. Then 10 mL methanol was introduced as sacrificial agent. At last the suspension was irradiated under UV light (11 W, 605 μmW cm−2) for 10 h. The Ag/g-C3N4 was obtained after filtration, washing and drying. The final silver concentration was about 140 mg g−1 and determined by ICP-OES.
The morphology of obtained samples was characterized by transmission electron microscopy (TEM, Tecnai F30). The crystal structure was examined by X-ray diffraction (XRD, X′ Pert PRO) at a scanning rate of 10° min−1 in the range of 10–80°. The chemical structure of the nanocomposite was studied by Fourier transform infrared spectrometer (FTIR) (Thermo Fisher Scientific). Photoluminescence (PL) spectra were studied by a HITACHI spectrometer (F-4600, Ex = 327 nm).
2.3 Preparation of Ag/g-C3N4/PES membranes
All membranes were prepared by the wet phase inversion process. Different amounts of dry Ag/g-C3N4 powders were added into DMF and well dispersed by ultrasonic. Then PES was dissolved and heated at 60 °C until completely dissolved. The dope solution was degassed at 60 °C and then scattered on the nonwoven fabric support with a casting knife gap setting of 200 μm. The nascent membrane was dipped in pure water (25 ± 1 °C) to induce phase inversion. The dope solution was prepared as shown in Table 1.
Table 1 The basic parameters of various membranes
Membrane abbreviation |
Silver content (%) |
PES (%) |
DMF (%) |
MWCO (kDa) |
CA (°) |
Porosity (%) |
M0 |
0 |
18 |
82 |
45 |
68.5 ± 3.6 |
70.2 |
M1 |
0.1 |
18 |
81.9 |
45 |
64.5 ± 2.1 |
71.6 |
M2 |
0.3 |
18 |
81.7 |
45 |
62.0 ± 1.7 |
74.5 |
M3 |
0.5 |
18 |
81.5 |
45 |
58.9 ± 2.1 |
79.3 |
M4 |
1.00 |
18 |
81.00 |
45 |
56.4 ± 1.7 |
81.8 |
2.4 Characterization of Ag/g-C3N4/PES membranes
The morphologies of the membranes were observed by SEM (HITACHI S-4800) after coating with gold. The hydrophilicity was evaluated by the contact angle test (CAM200, KSV). The measurements at six different locations were conducted to minimize the error.
The membrane porosity ε(%)of membranes can be estimated as below:
|
 | (1) |
The wet weight (ww) can be obtained after membranes were stored in deionized water for 24 h and dabbing it with filter paper. After 24 h drying at 60 °C, the dry weight (wd) can be got. A and l are the membrane area (m2) and thickness (m), respectively. dw is the water density (0.998 g cm−3).
2.5 Permeate flux measurements
UF experiment was conducted in a dead-end stirred cell at 0.1 MPa. The water flux (J) was obtained with eqn (2) |
 | (2) |
where V (L) is the volume of permeated water, A (m2) is the membrane area and Δt (h) is the filtration time.
Molecular weight cut-off (MWCO) is a parameter that reflects the surface pore size of membrane. It can be obtained by the protein rejection (R) calculation of different molecular weight such as BSA (69 kDa), egg albumin (45 kDa), pepsin (35 kDa) and trypsin (24 kDa) with eqn (3)
|
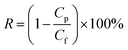 | (3) |
where
Cp and
Cf (g L
−1) is the permeate and feed protein concentration, respectively.
2.6 Antibacterial test
The antimicrobial activities of various membranes were firstly tested by agar diffusion method.19 Escherichia coli (E. Coli) and Pseudomonas aeruginosa (PA) were grown overnight. Then the sterilized membranes were put on the LB agar containing bacteria. After 24 h cultivation, the inhibition ring was recorded by the camera.
The colony forming units counting method was also conducted to characterize the antibacterial effect of various membranes.20 Briefly, the samples with the same diameter were immersed in the dilute bacterial suspension. After 12 h incubation the number of living bacteria was determined by Colony forming units (CFU) counting.
2.7 Photocatalytic property of membranes
The photocatalytic property of various membranes was tested by the MO degradation experiment. The visible-light was provided by a 300 W xenon lamp with an optical cut-off filter (λ ≥ 400 nm) and the intensity was 100 mW cm−2. First, the membrane (20 cm2) was anchored to the bottom of the Petri dish using double-sided tape to form a flat membrane surface. Then 50 mL of MO solution (10 mg L−1) was filled in to ensure the fully contact between membrane and dye molecules. The membrane samples were kept 10 cm vertical distance off the visible-light source. During the visible-light illumination, 3 mL solution was collected every 20 min to test the absorption value at 505 nm.
2.8 The antifouling characteristics in photocatalysis
In order to compare the antifouling performance of various membranes, the filtration experiments included four steps were conducted as follows. Firstly, the pure water flux (Jw1) was recorded for 0.5 h. Then the BSA solution (1 g L−1) was fed in the cell and the flux (Jp) was recorded for 0.5 h. The fouled membranes were cleaned by deionized water and the third water flux (Jrw) was measured for another 0.5 h. Finally, in order to prove the photocatalysis antifouling property of nanocomposite membrane, the BSA fouled membrane was irradiated by the xenon lamp for 1 h and the flux (Jw2) were recorded again for 0.5 h in order to get the flux recovery ratio. The FRR before and after visible light irradiation can be got using eqn (4). |
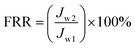 | (4) |
Moreover, the fouling resistance parameters were analyzed to study the fouling process. The irreversible (Rir), reversible (Rr) and total fouling ratio (Rt) were calculated as:
|
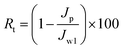 | (5) |
|
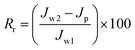 | (6) |
|
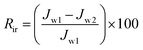 | (7) |
3. Results and discussion
3.1 The morphology of photocatalyst
TEM was used to observe the morphology of nanocomposite. Fig. 1a shows a typical rippled 2D paper-like flat layered structure of pure g-C3N4, which is consistent with reported results.12,16 From Fig. 1b it was clearly observed that a number of silver nanoparticles were well dispersed on the g-C3N4 sheet without obvious aggregation for composite material.
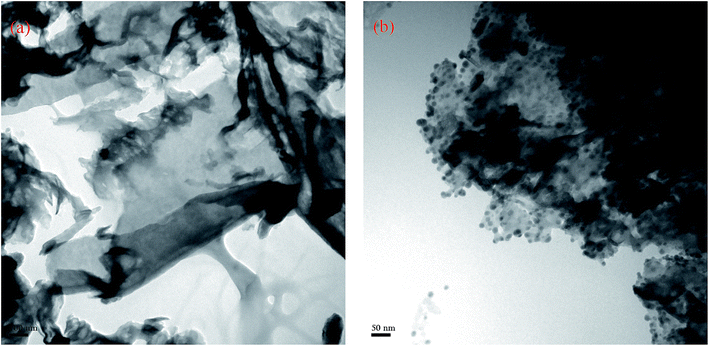 |
| Fig. 1 TEM morphology of (a) pure g-C3N4 nanosheet and (b) Ag/g-C3N4 composites. | |
The XRD pattern of nanocomposite is shown in Fig. 2. The peaks at 12.8° and 27.1° of pure g-C3N4 are attributed to the in-plane structure packing of tristriazine units and interlayer-stacking reflection respectively.12,21,22 As for the Ag/g-C3N4 sample, the diffraction peaks at 38.1° and 44.2° were observed which are indexed to the (111) and (200) planes of silver.12
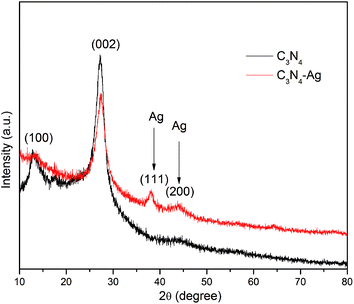 |
| Fig. 2 XRD patterns of the g-C3N4 and Ag/g-C3N4 composite. | |
The chemical structure of samples was further characterized by FTIR. Fig. 3 shows the typical molecular structure of g-C3N4. The broad peak at 3000–3500 cm−1 is attributed to the N–H stretching vibration.12,23 The peak at 808 cm−1 may be attributed to the triazine units breathing.24,25 The multiple peaks in the 1200–1700 cm−1 region, with the characteristic peaks at and 1240 and 1652 cm−1, may be assigned to the stretching vibration of CN heterocycles.22,25 The Ag/g-C3N4 sample shows the similar spectra, illustrating the doping of silver did not change the original chemical structure of g-C3N4 which was in accordance with the early reports.12
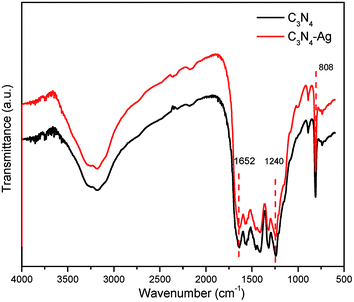 |
| Fig. 3 The FTIR characterization of the g-C3N4 and Ag/g-C3N4 composite. | |
PL spectra is a widely used method to study the photochemical behavior of photocatalyst. Fig. 4 showed that the emission peaks of samples centered at about 470 nm. The PL intensity of composite material reduced significantly compared to the g-C3N4. It is reported that the PL emission derives from the recombination of photo induced electron/hole pairs.12 The weaker intensity represents the lower recombination rate of free charge carriers.26 Therefore, the dispersion of Ag in the matrix of g-C3N4 could promote the charge separation, subsequently increasing the photocatalytic activity.12 The results above indicating that the use of Ag/g-C3N4 composite for the modification of the organic membrane is a meaningful attempt.
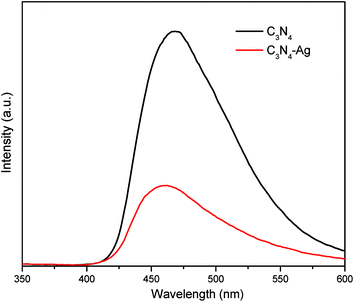 |
| Fig. 4 PL spectra of pure g-C3N4 and Ag/g-C3N4 composite. | |
3.2 Characterization of membranes
3.2.1 Membrane morphology. As shown in Fig. 5a, pure PES membrane (M0) has a smooth and clean surface. With the increase of Ag/g-C3N4 content, some white particles were observed on the nanocomposite membrane surfaces, which may be the aggregates of Ag/g-C3N4 nanosheets. The overall surface morphologies of nanocomposite membranes were still flat and smooth, indicating that the addition of Ag/g-C3N4 has little effects on surface structure.
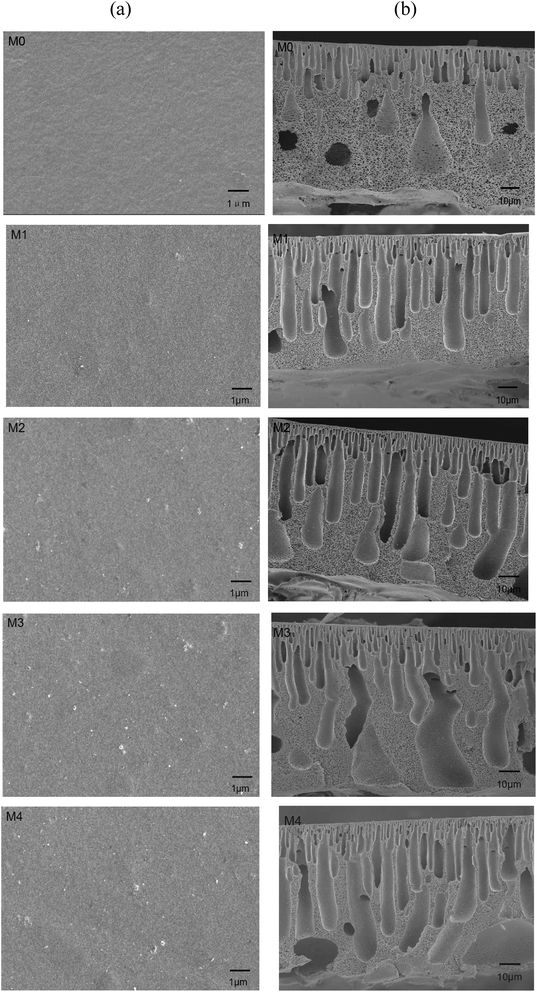 |
| Fig. 5 The SEM morphologies of membranes. (a) Surface, (b) cross section. | |
Fig. 5b exhibits all the membranes own the dense selective layer, thicker finger-like layer and sponge like pores at the bottom which is the typical asymmetric structure. Compared to M0, the finger-like voids in Ag/g-C3N4 modified membrane become longer and wider. This effect becomes more pronounced with the further increased Ag/g-C3N4 loading. As for M2, M3 and M4 the finger-like voids almost elongate across the whole cross section, also there are macropores formed at the bottom of M4, which are beneficial for the increased water flux. It is considered that the relative diffusion rate and driving force have great influences on membrane structure.27 The presence of Ag/g-C3N4 nanosheets may enhance the instability of casting solution and induce the accelerated demixing process. The accelerated demixing process may facilitate the fully development of the finger-like micro voids in nanocomposite membranes.28 It is also believed that if the nanoparticles had higher affinity to non-solvent, elongated macrovoids could appear due to the higher solvent/non-solvent exchange rate.29 In this study, the hydrophilic group such as –NH as shown in Fig. 3 and the highly dispersed hydrophilic silver nanoparticles on g-C3N4 nanosheets may facilitate the exchange rate of solvent and non-solvent. Hence, the macrovoids formed at the bottom of the composite membranes (M4) due to the presence of large amount of Ag/g-C3N4 nanosheets.
3.2.2 Hydrophilicity, porosity and pore size of various membranes. The contact angles were used to evaluate the surface hydrophilicity (Table 1). The contact angle of M0 was 68.5°, which was consistent with previous results.20 The overall contact angle reduced with the incorporation of Ag/g-C3N4, which revealed that the hydrophilicity of membrane was increased by Ag/g-C3N4. The existence of hydrophilic groups such as –NH2 or –NH after treated by sulfuric acid and nitric acid and the high affinity of Ag to water may be responsible for the hydrophilicity increase.12,18 This is also confirmed by FTIR results as shown in Fig. 3.The overall porosity of various membranes is shown in Table 1. A slightly increased porosity can be observed for Ag/g-C3N4 modified membranes. This is consistent with the cross-section pore structure change. As presented in Fig. 5b, wider and prolonged finger-like voids were formed due to the introduction of Ag/g-C3N4 nanosheets may lead to the increased porosity.
Molecular weight cut-off (MWCO) is an important parameter to reflect the UF membrane pore size. As shown in Table 1, all the membranes have the same MWCO of 45 kDa indicating that the additions of Ag/g-C3N4 nanosheets do not significantly change the surface membrane pore size.
3.3 The permeation and rejection properties
Dead-end filtration was performed to study the filtration performance of various samples as shown in Fig. 6. Compared to M0, the flux of nanocomposite membranes kept increasing and M4 had the highest flux of 268 L m−2 h−1. Considering all the membranes have the same MWCO, the changes in flux with the incorporation of Ag/g-C3N4 may be clarified as below. Firstly, the incorporation of g-C3N4 improved the hydrophilicity and the porosity of nanocomposite membranes which may further improve the water uptake property. Secondly, the introduction of Ag/g-C3N4 enlarged the finger-like pore and improved the inter-connectivity of cross section pore structure, which reduced the hydraulic resistance and enhanced the flux permeate. The flux change was consistent with the variation of pore structure, porosity and hydrophilicity of membrane surfaces.
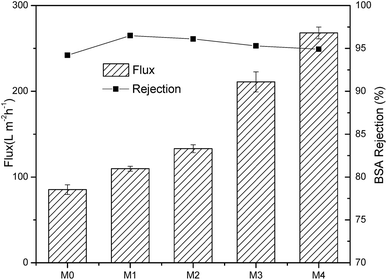 |
| Fig. 6 The pure water flux and BSA rejection of various membranes. | |
The BSA rejection of various membranes was 94.2%, 96.5%, 96.1%, 95.3%, 94.9%, respectively. The small differences in BSA rejection were attributed to the same MWCO of various membranes. The results indicated that the blending of Ag/g-C3N4 may be an effective way to promote the water flux without sacrifice of BSA rejection.
3.4 Antibacterial properties of membranes
The inhibition zone is a simple and widely used method to characterize the inactivation activity of membranes.30 As shown in Fig. 7, no bactericidal effect was observed for virgin PES (M0). All the modified membranes presented clear and wide inhibition zone and the width of inhibition zone was proportional to the Ag/g-C3N4 content, indicating the significant inactivation efficiency towards P. aeruginosa and E. coli.
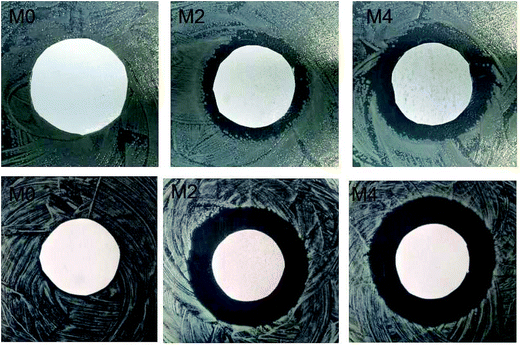 |
| Fig. 7 The antibacterial effect of nanocomposite membranes on (a) Escherichia coli (b) Pseudomonas aeruginosa observed in the disk diffusion test. | |
Moreover, the results of colony forming units counting also verified the inactivation efficiency. As shown in Table 2, the control (without membrane) and M0 had the maximal values of log
CFU. The exists of Ag/g-C3N4 modified membranes significantly decrease the number of live bacteria. Specifically for M3 and M4, no living bacteria were found in the test indicating the excellent bactericidal effect of nanocomposite membranes.
Table 2 Bacteriostatic effects of various membranes on Escherichia coli and Pseudomonas aeruginosaa
Membrane no. |
PA (log CFU) |
EC (log CFU) |
—: no found. |
Control |
10.78 ± 0.04 |
9.64 ± 0.07 |
M0 |
10.54 ± 0.08 |
9.50 ± 0.1 |
M1 |
4.53 ± 0.11 |
3.63 ± 0.06 |
M2 |
— |
2.91 ± 0.09 |
M3 |
— |
— |
M4 |
— |
— |
The results above clearly revealed that the modification of Ag/g-C3N4 could endow the PES membrane with outstanding antibacterial performance. It was reported that the silver based nanocomposite membranes could release silver ions (Ag+) to the surrounding.19 Ag+ can induce the serious destruction of cell membrane and proteins, which may be one of the main sources of excellent antimicrobial properties of nanocomposite membranes.3,4
3.5 Photocatalytic property of membranes
Methyl orange (MO) is a widely used organic dye. As reference, the visible-light photodegradation property of pure PES membrane (M0) was also investigated under the same condition. As shown in Fig. 8, almost no photo degradation effect of MO was observed for M0. This indicated that PES and nonwoven fabric didn't show any photocatalytic performance. In contrast, the introduction of Ag/g-C3N4 could enhance the photocatalytic activity of nanocomposite membranes under visible-light. The M2 owned 66% degradation efficiency toward MO. While the degradation activity kept improving with the increase of Ag/g-C3N4 content. As for M4, the degradation efficiency was up to 77%. The results above confirmed that the incorporation of Ag/g-C3N4 could significantly increase the photocatalytic property of nanocomposite membrane under visible-light illumination.
 |
| Fig. 8 Degradation rate of MO under visible light irradiation for various membranes. | |
The possible degradation mechanism under visible-light was mainly attributed to the generation of photo-electrons (e−) and holes (h+) by g-C3N4. The photo-electrons (e−) could react with the oxygen molecule (O2) to produce superoxide radical anion O2˙−. Meanwhile the Ag nanoparticles could entrap the e− to prevent the recombination of e− and h+, thus improving the transfer of charge carriers. The enriched holes and O2˙− are responsible for the degradation of Methyl orange (MO).6,17,31
3.6 The antifouling performance in photocatalysis
To study the alleviative effect of photocatalysis on membrane fouling, the BSA filtration tests were conducted. As shown in Fig. 9, the water flux declined sharply during BSA filtration step, which is attributed to the deposition of BSA and the formation of cake layers on membrane surfaces. Nevertheless, the Ag/g-C3N4 modified membranes owned higher fluxes compared to M0. After simple water rinsing, the fluxes of all samples enhanced in different degrees, indicating that the loosely bound BSA and the cake layer can be removed by hydraulic shear force.
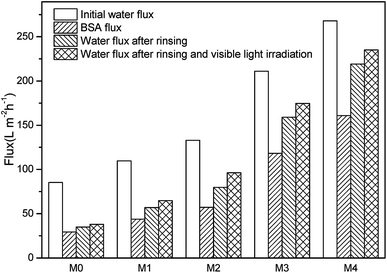 |
| Fig. 9 The flux values of various membranes at different processes. | |
Flux recovery ratio (FRR) is an important parameter to characterize the antifouling property of membranes and it is generally believed that the higher FRR means better antifouling performance.32,33 As shown in Fig. 10, the FRR of M0 was as low as 41% and all composite membranes had higher FRR than pristine PES membrane (without light). The modification of Ag/g-C3N4 significantly increased the FRR of nanocomposite membranes and M4 owned the maximum FRR (81.7%) without visible-light irradiation. The improved hydrophilicity of membrane surface (Table 1) compared to M0 may contribute to the promoted antifouling behavior. After the supplementation of visible light illumination following water rinsing, further flux increase was observed for nanocomposite membranes (Fig. 9) and the FRR reached to 59.1%, 72.2%, 82.7% and 87.7% respectively (Fig. 10). But as for M0, the further flux increment was less than that of composite membranes. The further recovery of flux and FRR indicated the photocatalytic degradation of strongly bound organic pollutants, which was similar with the results before.32,33
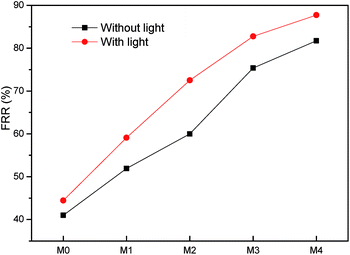 |
| Fig. 10 The flux recovery ratio (FRR) of membranes before and after visible light irradiation. | |
To further investigate the membrane fouling details, different fouling ratio were calculated. The Rr and Rir were mainly related to loosely bound protein on membrane surface and the plugging of protein in membrane pores, respectively.33
As shown in Fig. 11, all the Ag/g-C3N4 modified membranes had obvious lower Rt than M0, which may be explained by the increase of surface hydrophilicity. The initial Rr values after water rinsing of various samples were 6.5%, 11.8%, 16.9%, 19.4% and 21.7% respectively. After the visible light illumination, the Rr value of Ag/g-C3N4 modified membranes increased to 19.1%, 29.1%, 26.7% and 27.7%, respectively.
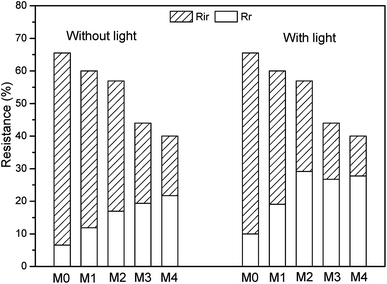 |
| Fig. 11 The distribution of fouling resistance of all the membranes. | |
Moreover, the ratio of Rir and Rr to the Rt was also calculated. As shown in Fig. 12, the Rr/Rt was 9.9%, 19.8%, 29.7%, 44.0% and 54.3% for various samples without visible light illumination. While the Rr/Rt values increased to 15.3%, 31.8%, 51.2%, 60.8% and 69.3% under visible light irradiation, respectively. This trend showed that reversible fouling (Rr) gradually became the dominant fouling factor with the increase of nanocomposite loading. Fig. 10–12 were consistent with each other, indicating the improved antifouling performance of membranes with the addition of Ag/g-C3N4.
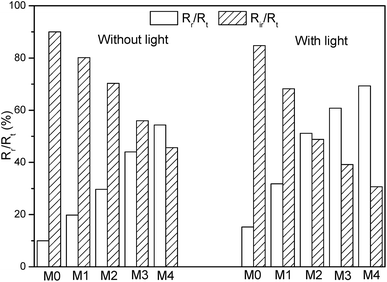 |
| Fig. 12 The ratio of irreversible fouling (Rir) and reversible fouling (Rr) to the total fouling (Rt). | |
As mentioned above, the enriched holes and O2˙− that generated by g-C3N4 under visible light irradiation can degrade many kinds of complex organic materials.34,35 All the results above confirmed that the holes and O2˙− can induce the degradation of strongly bound pollutants and make the pollutants more easier to be removed from membrane surface, thus decreasing the Rir and improving the photocatalytic antifouling property of nanocomposite membranes.
4. Conclusions
In this study, a novel Ag/g-C3N4/PES ultrafiltration membrane was successfully fabricated by phase-inversion method. The incorporation of Ag/g-C3N4 into PES membranes as additives was systematically studied. The SEM pictures illustrated that the addition of Ag/g-C3N4 could make the finger-like voids in Ag/g-C3N4 nanocomposite membranes longer and wider. The membrane hydrophilicity and porosity were slightly enhanced due to the introduction of Ag/g-C3N4 nanosheets. The bacterial tests showed the excellent antibacterial property of Ag/g-C3N4 based nanocomposite membranes. The dye degradation experiments confirmed the significantly improved photocatalytic effect of nanocomposite membrane by the incorporation of Ag/g-C3N4 nanosheets with visible light illumination. The BSA filtration experiments indicated the introduction of Ag/g-C3N4 can endow PES membrane with photocatalytic self-cleaning property and improve the antifouling performance. The work may contribute to a potential research of the Ag/g-C3N4 in advanced membrane materials. All advantages shown by the Ag/g-C3N4/PES nanocomposite membrane make it promising for wider industrial application.
Conflicts of interest
There are no conflicts to declare.
Acknowledgements
This work was supported by the National Natural Science Foundation of China (51508239) and Jiangsu province (BK20150245, BY2016030-01), Postgraduate Research & Practice Innovation Program of Jiangsu Province (SJCX17-0772) and Qing Lan Project.
References
- C. S. Ong, P. S. Goh, W. J. Lau, N. Misdan and A. F. Ismail, Nanomaterials for biofouling and scaling mitigation of thin film composite membrane: A review, Desalination, 2016, 393, 2–15 CrossRef CAS.
- Q. Yang and B. Mi, Nanomaterials for Membrane Fouling Control: Accomplishments and Challenges, Adv. Chron. Kidney. Dis., 2013, 20, 536–555 CrossRef PubMed.
- L. F. Dumée, L. He, P. C. King, M. L. Moing, I. Güller, M. Duke, P. D. Hodgson, S. Gray, A. J. Poole and L. Kong, Towards integrated anti-microbial capabilities: Novel bio-fouling resistant membranes by high velocity embedment of silver particles, J. Membr. Sci., 2015, 475, 552–561 CrossRef.
- M. Sile-Yuksel, B. Tas, D. Y. Koseoglu-Imer and I. Koyuncu, Effect of silver nanoparticle (AgNP) location in nanocomposite membrane matrix fabricated with different polymer type on antibacterial mechanism, Desalination, 2014, 347, 120–130 CrossRef CAS.
- M. Ben-Sasson, X. Lu, E. Bar-Zeev, K. R. Zodrow, S. Nejati, G. Qi, E. P. Giannelis and M. Elimelech, In situ formation of silver nanoparticles on thin-film composite reverse osmosis membranes for biofouling mitigation, Water Res., 2014, 62, 260–270 CrossRef CAS PubMed.
- Y. Zhang and M. Cui, Porous YxFeyZr1−x−yO2 coated TiO2 solid superacid particles/PVDF hybrid membranes with anti-fouling property, Chem. Eng. J., 2016, 301, 342–352 CrossRef CAS.
- M. Safarpour, V. Vatanpour and A. Khataee, Preparation and characterization of graphene oxide/TiO2 blended PES nanofiltration membrane with improved antifouling and separation performance, Desalination, 2016, 393, 65–78 CrossRef CAS.
- N. A. M. Nor, J. Jaafar, A. F. Ismail, M. A. Mohamed, M. A. Rahman, M. H. D. Othman, W. J. Lau and N. Yusof, Preparation and performance of PVDF-based nanocomposite membrane consisting of TiO2 nanofibers for organic pollutant decomposition in wastewater under UV irradiation, Desalination, 2016, 391, 89–97 CrossRef CAS.
- Y. Zhang and J. Zhu, Composite photocatalytic membrane prepared by embedding porous SiO2 shell/void/TiO2 core particles into polycarbonate for photodegrading and removing pollutant from water, Chem. Eng. Sci., 2015, 126, 390–398 CrossRef CAS.
- J. P. Méricq, J. Mendret, S. Brosillon and C. Faur, High performance PVDF-TiO2 membranes for water treatment, Chem. Eng. Sci., 2015, 123, 283–291 CrossRef.
- Z. Zhu, X. Tang, C. Ma, M. Song, N. Gao, Y. Wang, P. Huo, Z. Lu and Y. Yan, Fabrication of conductive and high-dispersed Ppy@Ag/g-C3N4 composite photocatalysts for removing various pollutants in water, Appl. Surf. Sci., 2016, 387, 366–374 CrossRef CAS.
- W. Zhang, L. Zhou and H. Deng, Ag modified g-C3N4 composites with enhanced visible-light photocatalytic activity for diclofenac degradation, J. Mol. Catal. A: Chem., 2016, 423, 270–276 CrossRef CAS.
- M. Zhang, W. Luo, Z. Wei, W. Jiang, D. Liu and Y. Zhu, Separation free C3N4/SiO2 hybrid hydrogels as high active photocatalysts for TOC removal, Appl. Catal., B, 2016, 194, 105–110 CrossRef CAS.
- G. Mamba and A. K. Mishra, Graphitic carbon nitride (g-C3N4) nanocomposites: A new and exciting generation of visible light driven photocatalysts for environmental pollution remediation, Appl. Catal., B, 2016, 198, 347–377 CrossRef CAS.
- M. J. Muñoz-Batista, O. Fontelles-Carceller, M. Ferrer, M. Fernández-García and A. Kubacka, Disinfection capability of Ag/g-C3N4 composite photocatalysts under UV and visible light illumination, Appl. Catal., B, 2016, 183, 86–95 CrossRef.
- S. Ma, S. Zhan, Y. Jia, Q. Shi and Q. Zhou, Enhanced disinfection application of Ag-modified g-C3N4 composite under visible light, Appl. Catal., B, 2016, 186, 77–87 CrossRef CAS.
- H. Zhao, S. Chen, X. Quan, H. Yu and H. Zhao, Integration of microfiltration and visible-light-driven photocatalysis on g-C3N4 nanosheet/reduced graphene oxide membrane for enhanced water treatment, Appl. Catal., B, 2016, 194, 134–140 CrossRef CAS.
- M. Zhang, R. W. Field and K. Zhang, Biogenic silver nanocomposite polyethersulfone UF membranes with antifouling properties, J. Membr. Sci., 2014, 471, 274–284 CrossRef CAS.
- S. Liu, M. Zhang, F. Fang, L. Cui, J. Wu, R. Field and K. Zhang, Biogenic silver nanocomposite TFC nanofiltration membrane with antifouling properties, Desalin. Water Treat., 2015, 1–12 CrossRef.
- M. Zhang, K. Zhang, B. De Gusseme and W. Verstraete, Biogenic silver nanoparticles (bio-Ag0) decrease biofouling of bio-Ag0/PES nanocomposite membranes, Water Res., 2012, 46, 2077–2087 CrossRef CAS PubMed.
- L. C. Chen, X. T. Zeng, P. Si, Y. M. Chen, Y. W. Chi, D. H. Kim and G. N. Chen, Gold Nanoparticle-Graphite-Like C3N4 Nanosheet Nanohybrids Used for Electrochemiluminescent Immunosensor, Anal. Chem., 2014, 86, 4188–4195 CrossRef CAS PubMed.
- L. Liu, Y. Qi, J. Lu, S. Lin, W. An, Y. Liang and W. Cui, A stable Ag3PO4@g-C3N4 hybrid core@shell composite with enhanced visible light photocatalytic degradation, Appl. Catal., B, 2016, 183, 133–141 CrossRef CAS.
- M. Faisal, A. A. Ismail, F. A. Harraz, S. A. Al-Sayari, A. M. El-Toni and M. S. Al-Assiri, Synthesis of highly dispersed silver doped g-C3N4 nanocomposites with enhanced visible-light photocatalytic activity, Mater. Des., 2016, 98, 223–230 CrossRef CAS.
- Y. Shi, S. Jiang, K. Zhou, C. Bao, B. Yu, X. Qian, B. Wang, N. Hong, P. Wen, Z. Gui, Y. Hu and R. K. K. Yuen, Influence of g-C3N4 Nanosheets on Thermal Stability and Mechanical Properties of Biopolymer Electrolyte Nanocomposite Films: A Novel Investigation, ACS Appl. Mater. Interfaces, 2013, 6, 429–437 Search PubMed.
- S. C. Yan, Z. S. Li and Z. G. Zou, Photodegradation Performance of g-C3N4 Fabricated by Directly Heating Melamine, Langmuir, 2009, 25, 10397–10401 CrossRef CAS PubMed.
- X. Chen, J. Wei, R. Hou, Y. Liang, Z. Xie, Y. Zhu, X. Zhang and H. Wang, Growth of g-C3N4 on mesoporous TiO2 spheres with high photocatalytic activity under visible light irradiation, Appl. Catal., B, 2016, 188, 342–350 CrossRef CAS.
- W. Zhao, Y. Su, C. Li, Q. Shi, X. Ning and Z. Jiang, Fabrication of antifouling polyethersulfone ultrafiltration membranes using Pluronic F127 as both surface modifier and pore-forming agent, J. Membr. Sci., 2008, 318, 405–412 CrossRef CAS.
- X. Li, X. Fang, R. Pang, J. Li, X. Sun, J. Shen, W. Han and L. Wang, Self-assembly of TiO2 nanoparticles around the pores of PES ultrafiltration membrane for mitigating organic fouling, J. Membr. Sci., 2014, 467, 226–235 CrossRef CAS.
- V. Vatanpour, S. S. Madaeni, A. R. Khataee, E. Salehi, S. Zinadini and H. A. Monfared, TiO2 embedded mixed matrix PES nanocomposite membranes: Influence of different sizes and types of nanoparticles on antifouling and performance, Desalination, 2012, 292, 19–29 CrossRef CAS.
- J.-H. Li, B.-F. Yan, X.-S. Shao, S.-S. Wang, H.-Y. Tian and Q.-Q. Zhang, Influence of Ag/TiO2 nanoparticle on the surface hydrophilicity and visible-light response activity of polyvinylidene fluoride membrane, Appl. Surf. Sci., 2015, 324, 82–89 CrossRef CAS.
- Y. Zhang, L. Wang and Y. Xu, ZrO2 solid superacid porous shell/void/TiO2 core particles (ZVT)/polyvinylidene fluoride (PVDF) composite membranes with anti-fouling performance for sewage treatment, Chem. Eng. J., 2015, 260, 258–268 CrossRef CAS.
- Z. Xu, T. Wu, J. Shi, K. Teng, W. Wang, M. Ma, J. Li, X. Qian, C. Li and J. Fan, Photocatalytic antifouling PVDF ultrafiltration membranes based on synergy of graphene oxide and TiO2 for water treatment, J. Membr. Sci., 2016, 520, 281–293 CrossRef CAS.
- R. A. Damodar, S.-J. You and H.-H. Chou, Study the self cleaning, antibacterial and photocatalytic properties of TiO2 entrapped PVDF membranes, J. Hazard. Mater., 2009, 172, 1321–1328 CrossRef CAS PubMed.
- B. Lin, C. Xue, X. Yan, G. Yang, G. Yang and B. Yang, Facile fabrication of novel SiO2/g-C3N4 core–shell nanosphere photocatalysts with enhanced visible light activity, Appl. Surf. Sci., 2015, 357, 346–355 CrossRef CAS.
- H. Xu, H. Zhao, Y. Song, W. Yan, Y. Xu, H. Li, L. Huang, S. Yin, Y. Li, Q. Zhang and H. Li, g-C3N4/Ag3PO4 composites with synergistic effect for increased photocatalytic activity under the visible light irradiation, Mater. Sci. Semicond. Process., 2015, 39, 726–734 CrossRef CAS.
|
This journal is © The Royal Society of Chemistry 2017 |