DOI:
10.1039/C7RA06913H
(Paper)
RSC Adv., 2017,
7, 54100-54110
In vitro and in vivo studies of a gelatin/carboxymethyl chitosan/LAPONITE® composite scaffold for bone tissue engineering
Received
21st June 2017
, Accepted 20th November 2017
First published on 24th November 2017
Abstract
In the present study, we fabricated a biocomposite scaffold composed of carboxymethyl chitosan (CMC), gelatin and LAPONITE® (Lap) nanoparticles via freeze-drying and investigated its potential use in bone tissue engineering. The prepared gelatin/carboxymethyl chitosan (GC) scaffolds and laponite-incorporated scaffolds were characterized by scanning electron microscopy (SEM), and Fourier transform infrared spectroscopy (FTIR) analyses. The swelling and biodegradation were also investigated. In vitro assays such as cell attachment and proliferation, osteogenic differentiation of rat bone marrow-derived mesenchymal stem cells (rBMCSs) grown on those scaffolds and in vivo cranial bone defect assays were further carried out. We found that our prepared scaffolds had a porous architecture, and the increased Lap content resulted in improved mechanical strength, whereas the swelling ratio and degradation rate decreased. In vitro cell proliferation and live cell staining experiments demonstrated that the addition of Lap (5 and 10 wt% relative to gelatin, GC-Lap5% and GC-Lap10% respectively) would facilitate cell proliferation, but caused an inhibition effect at 15% of Lap content (GC-Lap15%). Furthermore, GC-Lap10% induced a higher degree of osteogenic differentiation of rBMSCs compared with the GC scaffold and GC-Lap5% scaffold. More importantly, in vivo cranial defect experiments revealed that the addition of Lap into the GC scaffold promoted bone regeneration. These findings indicate that a composite scaffold with Lap incorporation is a promising material for bone tissue engineering.
1. Introduction
Bone defects and non-unions in the clinic have traditionally been repaired with autografts or allografts.1 However, these approaches are restricted by donor shortages, donor-site morbidity, and the risk of disease transfer.2,3 A promising alternative to bone grafting is bone tissue engineering (BTE), in which bone tissue is constructed de novo by combining bone-forming cells, growth factors, and three-dimensional (3D) porous scaffolds.4
The scaffold used in BTE acts as an artificial extracellular matrix (ECM) to support cell adhesion and promote cell differentiation without hindering proliferation, thereby guiding bone regeneration.5 Recently, many types of synthetic and natural polymer materials have been used as scaffolds and have shown promising results for bone regeneration.6–9 Gelatin, a hydrolytic derivative of collagen, has been widely used in the biomedical field due to its stable physicochemical properties, lack of antigenicity, and low cost.10,11 In addition, the presence of an Arg-Gly-Asp-like sequence in gelatin can also promote cell attachment, differentiation, and proliferation.12,13 However, low mechanical strength, poor elasticity, and morphological instability limit its biological applications.14 To improve chemical and biological performance, crosslinking or combining gelatin with other biopolymers has been proposed.15 Carboxymethyl chitosan (CMC), a water-soluble derivative of chitosan, has high aqueous solubility, controllable biodegradability, and osteogenesis-inducing potential as compared to chitosan.16 Previous studies have shown that blending CMC with gelatin can improve the physicochemical and biological properties of the gel, including mechanical strength, biocompatibility, and capacity to induce osteogenesis.16,17 Thus, gelatin/carboxymethyl-chitosan (GC) composites have various applications in wound healing and cartilage and bone regeneration.15–17 Despite interesting physical, chemical, and biological properties, insufficient osteoinductive properties and poor mechanical loading make the GC composites scaffolds far from satisfactory.
Nanoclay, namely synthetic silicate, has a high aspect-ratio morphology that enhances the structural integrity of nanocomposites into which it is incorporated. The physical and chemical properties of nanocomposite matrices can be modified by adding nanoclay.18,19 For example, the incorporation of montmorillonite into poly(lactic acid) scaffolds increased its compressibility to a level comparable to that of cancellous bone.20 Cloisite, another clay, were used to adjust the mechanical parameters of poly[ethylene-co-(vinyl acetate)].21 Halloysite, when added as an inorganic filler via electrospinning was found to improve the mechanical properties of poly(ε-caprolactone) (PCL)/nanocellulose fibrous scaffolds.22 Despite advances in the physical and chemical properties of polymers by reinforcement with nanoclay, few studies have examined the interaction between clay-based scaffolds and cells.23–26 Ambre et al. reported that incorporating synthetic silicate into chitosan/polygalacturonic acid scaffolds promoted osteogenic differentiation of human mesenchymal stem cells (hMSCs).26 Hong Zhuang et al. found out that montmorillonite-intercalated gelatin/chitosan induced the adhesion and proliferation of rat stromal stem cells;27 Ganesh Nitya et al. demonstrated hMSCs seeded on scaffolds embedded with halloysite nanoclay showed higher proliferation rate and alkaline phosphatase (ALP) activity.22
LAPONITE® (Lap), a silicate that usually acted as a physical or covalent cross-linker in polymer fabrication; it has recently received much attention because of its excellent physical and chemical properties as well as ideal osteogenesis-inducing potential.23,24,28,29 Incorporating Lap into polymers has shown to alter their characteristics such as hydration, dissolution, and mechanical properties. On the other hand, the adhesion, proliferation, and osteogenic potential of cells cultured on these nanocomposites are correlated with Lap concentration.23–25 For example, Lap crosslinking not only improved the mechanical strength and network stability of a poly(ethylene oxide) (PEO) nanocomposite polymer, but also enhanced the adhesion, spreading, proliferation, and mineralization of preosteoblast cells.23 Recently, an electrospun PCL scaffold was fabricated in which surface roughness, degradation rate, and mechanical properties were markedly altered by adding Lap; moreover, the adhesion, proliferation, and osteogenic differentiation of hMSCs grown on the these scaffolds were dependent on nanoclay concentration.24
Despite previous studies have displayed the beneficial effect of Lap in preparing novel bioactive materials in tissue engineering,23,30 further in vivo analysis needs to be performed before Lap incorporated materials can be used in clinical applications. Meanwhile, for BTE application, especially in regeneration of large bone defect, silicate nanoparticles developed materials should be highly porous to induce cell migration and allow nutrient transportation as well as to improve bone tissue ingrowth.31 In this regard, a promising solution lies in the incorporation of Lap nanoplatelets with polymers to fabricate a 3D porous scaffold. To the best of our knowledge, there have been no studies to date describing the reinforcement of GC scaffolds with Lap for BTE applications. Furthermore, there is no information on the biocompatability and bioactivity of polymer/Lap composites in vivo.24 In the present study, we fabricated a porous Lap-incorporated GC scaffold by freeze drying. The scaffold was characterized with regards to its physical and mechanical properties, biodegradability, and biocompatibility as well as its ability to promote osteogenesis in vitro and in vivo.
2. Experimental
2.1 Materials
α-Minimal Essential Medium (α-MEM), fetal bovine serum, PBS, penicillin, and streptomycin were purchased from Gibco (Carlsbad, CA, USA). Lap (Na0.7+[Mg5.5:Li0.3Si8O20(OH)4]0.7−) with an average diameter of 25–30 nm and 1 nm in thickness was a kindly gift from Donghua University. CMC (degree of deacetylation 96.5%; MW: 70
000) was purchased from Qingdao Honghai Co. Ltd (Qingdao, China). Gelatin (∼225 g bloom, type B) was purchased from Sigma-Aldrich (St. Louis, MO, USA). 1-(3-Dimethylaminopropyl)-3-ethylcarbodiimide hydrochloride (EDC) and N-hydroxysuccinimide (NHS) were obtained from Energy Chemical (Shanghai, China). Other chemicals were purchased from Sinopharm Chemical Regent Co. Ltd (Shanghai, China). The rBMSCs (passage 3) were purchased from the Type Culture Collection of the Chinese Academy of Sciences, Shanghai, China. The water used in this study was from a Millipore Milli-Q system (Millipore, Bedford, MA) with resistivity of 18.2 MΩ cm at 25 °C.
2.2 Preparation of GC and GC-Lap scaffolds
A CMC solution was obtained by dissolving 80 mg of powder in ultrapure water at 40 °C. Gelatin powder (800 mg) was added while stirring for 1 h to obtain the GC solution. For Lap incorporation, appropriate amounts of Lap (5%, 10%, and 15% of the gelatin mass) were introduced into the above mixture. After stirring for 30 min, the mixture was poured into the mold and frozen overnight at −20 °C, then lyophilized for 48 h. Dried scaffolds were crosslinked using EDC and NHS in a mixed solvent of acetone and water (volume ratio: 4
:
1) for 24 h at 4 °C. The scaffolds were lyophilized and stored at −20 °C. The synthetic process of GC-Lap scaffolds was illustrated in the Scheme 1.
 |
| Scheme 1 The schematic diagram of composite scaffold processing. | |
2.3 Scaffold characterization
The surface morphology of prepared scaffolds was examined by SEM (TM-1000; Hitachi, Tokyo, Japan). FTIR spectroscopy was carried out on Nicolet 6700 instrument (Thermo Fisher Scientific, Waltham, MA, USA).
2.4 Measurement of swelling
To determine the amount of fluid absorbed, swelling assay was conducted by immersing the scaffolds into PBS (pH 7.4) at room temperature. At predetermined time intervals up to 2 h, the swollen samples were taken out from the solution and blotted onto filter paper to remove the excess solution, and the wet weight (Ww) was recorded. After drying, all the samples were weighted (Wd). Each sample was carried out in triplicates. The swelling percentage was calculated as follows:
S = [(Ww − Wd)/Wd] × 100% |
2.5 Evaluation of mechanical properties (compression test)
To assess the mechanical properties of the scaffolds, the compressive properties were measured with a mechanical tester (HY-940FS; Shanghai Hengyu Co., Ltd, Shanghai, China) fitted with a 200 N load cell and operated at a loading speed of 1 mm min−1. Cylindrical samples (n = 5) approximately 6 mm thick and 10 mm in diameter were compressed until the thickness was reduced to 60% of the original value. The Young's modulus was determined as the initial linear section of the stress–strain curve.
2.6 Evaluation of degradation in vitro
Three specimens of each type of scaffold were immersed in PBS (pH 7.4) containing 8 × 104 U ml−1 lysozyme at 37 °C, and degradation was assessed by monitoring weight loss. At predetermined time intervals (1, 3, 5, 7, 14, and 21 days), the scaffolds were removed from the solution, washed with deionized water, freeze-dried under vacuum, and weighed. The degradation ratio was calculated as follows:
where W0 and Wt represent the original weight and weight after incubation in lysozyme solution up to time t (days), respectively.
2.7 Cell proliferation assay
The MTT assay was used to analyze the biocompatibility of the scaffolds (pure GC, GC-Lap5%, GC-Lap10%, and GC-Lap15%). The scaffolds were sterilized by immersion in 75% ethanol for 12 h and then rinsed with PBS. Passage 3 rBMSCs (1 × 104) were cultured on the scaffolds for 1, 3, and 7 days. A 20 μl volume of MTT solution (5 mg ml−1) was added to each well followed by incubation at 37 °C for 2 h; 200 μl dimethylsulfoxide was then added in the dark on an oscillator to dissolve the formazan crystals. A 100 μl aliquot of each sample was transferred to a 96-well plate and the optical density (OD) at 492 nm was measured.
2.8 Evaluation of cell attachment
Sterilized scaffolds were placed in a 48-well plate and equilibrated in culture medium for 1 h. rBMSCs (1 × 104/well) were seeded on the scaffolds followed by incubation at 37 °C and 5% CO2 in a 100% humidified atmosphere. After 3 days, the culture medium was replaced with 1 ml calcein-AM solution (8 μg ml−1 in Hank's balanced salt solution) and the plate was incubated for 15 min at 37 °C and 5% CO2. The morphology of the attached cells was examined by confocal laser scanning microscopy (Leica, Tokyo, Japan).
For SEM analysis, scaffolds were removed from the wells after 12 h of culture and washed with PBS, then fixed with 2.5% glutaraldehyde for 2 h. After dehydration in a graded series of ethanol (50%, 70%, 80%, 90%, and 100%) and thermostatic drying, the scaffolds were gold sputtered and cell morphologies were analyzed by SEM.
2.9 ALP staining and quantitative analysis
Passage 3 rBMSCs were seeded on the surface of the scaffolds (GC, GC-Lap5%, and GC-Lap10%) in osteogenic medium in a 24-well plate at a density of 5 × 104 cells per well. On days 7, 14, and 21, ALP activity was quantified with an ALP detection kit (Jiancheng Technology, Nanjing, China) according to the manufacturer's instructions and normalized to total protein content, which was determined with the bicinchoninic acid assay. For ALP staining, scaffolds cultured for 7 or 14 days were rinsed three times with PBS and then fixed with 4% paraformaldehyde solution for 10 min. The cells were stained with 5-bromo-4-chloro-3-indolylphosphate and nitroblue tetrazolium ALP substrate (Beyotime Institute of Biotechnology, Shanghai, China) for 30 min at room temperature. The substrate solution was removed and distilled water was added to terminate the reaction.
2.10 Quantitative real time PCR (qRT-PCR) analysis
Runx-2, OCN, OPN and ALP expression in rBMSCs was evaluated by qRT-PCR after 7 and 14 days of culture on the scaffolds in osteogenic medium. Total RNA was extracted from cells using TRIzol reagent (Invitrogen), and the concentration and purity were confirmed by measuring OD at 260 and 280 nm. Samples with OD260/280 ratios between 1.9 and 2.1 were used for cDNA synthesis by reverse transcribing 1 mg total RNA in a final volume of 20 μl using the PrimeScript RT Reagent kit (Takara Bio, Otsu, Japan). PCR was performed as previously described32 using Power SYBR Green PCR Master Mix (Applied Biosystems, Foster City, CA, USA) on a 7500 Real-Time PCR detection system. The primer sequences are shown in Table 1. Relative mRNA levels were determined with the 2−ΔΔCt method,33 with glyceraldehydes-3-phosphate dehydrogenase (GAPDH) used as a reference gene. All reactions were carried out in triplicate.
Table 1 Primer sequences used for real-time PCR primer
Primer |
Sequence (5′–3′) |
Runx-2 |
F: 5′-GCGGACGAGGCAAGAGTT-3′ |
R: 5′-TTGGTGCTGAGTTCAGGGAG-3′ |
OCN |
F: 5′-CAGGTGCAAAGCCCAGCGACT-3′ |
R: 5′-AGGGGATCTGGGTAGGGGGCT-3′ |
OPN |
F: 5′-CAGTATCCCGATGCCACA-3′ |
R: 5′-TTTCCACGCTTGGTTCAT-3′ |
ALP |
F: 5′-TCACTTCCGCCCGGAACCCT-3′ |
R: 5′-TGTCCTGCCGGCCCAAGAGA-3′ |
GAPDH |
F: 5′-TGCACCACCAACTGCTTAGC-3′ |
R: 5′-GGCATGGACTGTGGTCATGAG-3′ |
2.11 Animal experiments
The use of animals and experimental protocol were approved by the Animal Research Committee of the Ninth People's Hospital, Shanghai Jiao Tong University School of Medicine. The procedure performed was in accordance with the Guide of the Care and Use of Laboratory Animals published by the National Academy of Sciences. The pie-shaped (φ5 mm × 1 mm3) porous scaffolds were sterilized and seeded with rBMSCs. All surgical procedures were performed on 12 week-old male Sprague-Dawley rats (n = 24) as previously described.34 A sagittal incision about 2 cm was made on the scalp. After blunt dissection, the calvarium was exposed. Bilateral critical-sized defects (CSDs) (5 mm-diameter) were created in every rat with an electric trephine (Saeshin, Taegu, South Korea). Then the cell-seeded scaffolds were implanted into the defects after 12 h of culture. The rats were randomly distributed to one of the following groups (n = 8 each): (1) blank (right CSD) and GC scaffold with rBMSCs (left CSD); (2) blank (right CSD) and GC-Lap5% scaffold with rBMSCs (left CSD); and (3) blank (right CSD) and GC-Lap10% with rBMSCs (left CSD). Rats showed normal activity after this procedure.
2.11.1 Micro-CT. At 4 and 8 weeks after the surgery, the rats were sacrificed by air embolization. Then the skulls were dissected and fixed in 4% paraformaldehyde immediately. The morphology of the reconstructed skulls was assessed by micro-CT (mCT-80; Scanco Medical, Brüttisellen, Switzerland) in high-resolution scanning mode (pixel matrix, 1024 × 1024; voxel size, 20 mm; slice thickness, 20 mm) to determine bone volume. After the scan, an image of the bone was reconstructed using GEHC Micro-View software (GE Healthcare BioSciences, Chalfont-St. Giles, UK). Bone volume/total volume (BV/TV) and bone mineral density were also calculated with the software.
2.11.2 Histological analysis. After micro-CT analysis, the skull of each mouse was dehydrated in increasing concentrations of alcohol (70–100%) and embedded in polymethyl methacrylate until solidification. Coronal sections (approximately 100 μm in thickness) of the central area from each defect were cut and ground. Specimens were polished to a final thickness of approximately 20–30 μm and stained with van Gieson's picrofuchsin to identify new bone formation. The area of newly formed bone was quantified with Image Pro Plus software (Media Cybernetics, Rockville, MD, USA) as a percentage of the whole bone defect area.
2.12 Statistical analysis
SPSS 17.0 software (SPSS Inc., Chicago, USA) was used for statistical analysis and the data were demonstrated as the means ± standard deviation (SD). Statistical differences were determined by an analysis of variance (ANOVA) with a level of statistically significance set at P < 0.05.
3. Results and discussion
3.1 Scaffold characterization
Open and interconnected pores promote gas and nutrient transport and waste diffusion, which are necessary for cell migration, proliferation, and differentiation.35,36 Additionally, an appropriate pore size in scaffolds is critical for promoting vascularization and bone growth.37 As observed in Fig. 1, the SEM images showed that our prepared scaffolds in this study were highly porous and interconnected. The pore diameter decreased with the increasing Lap content, ranging from 200–380, 160–300, 130–250, and 100–220 μm for pure GC scaffold and GC-Lap scaffolds containing 5% (GC-Lap5%), 10% (GC-Lap10%) and 15% (GC-Lap15%) Lap, respectively. This suggests that the incorporation of Lap nanoparticles reduced the average pore size in scaffolds, which was to some extent proportional to Lap concentration. It has been reported that a suitable pore size distribution for osteoinductivity or for promoting stem cell infiltration ranges from 100 to 400 μm;38 the pore sizes of the prepared GC-Lap scaffolds are within this range. Additionally, with the increase of Lap, the surface roughness in the pore wall of scaffolds increased in a concentration-dependent manner (Fig. 1). This was more evident in the GC scaffolds containing 10% and 15% nanoclay (GC-Lap10% and GC-Lap15%), showing greater roughness than that of GC-Lap5%. This is similar to what has been observed in chitosan-based polymer scaffolds containing nanohydroxyapatite,39 in which nanoparticles deposited along pore walls and therefore promoted mineralization, protein adsorption, and cell adhesion.40
 |
| Fig. 1 SEM images of GC (A and B), GC-Lap5% (C and D), GC-Lap10% (E and F) and GC-Lap15% scaffolds (G and H). A, C, E, G show the SEM for magnification ×100; B, D, F, H show the SEM for magnification ×3000. | |
3.2 Fourier transform infrared (FTIR) spectroscopy analysis
The FTIR spectra were obtained to confirm the blending of CMC and incorporation of Lap in the Lap-contained GC sample (Fig. 2). In the spectrum of pure gelatin scaffold, typical characteristic absorption bands at 3285 and 3073 cm−1 were attributed to N–H stretching of amide II, while those at 2940, 1631, 1544, 1231 cm−1 were ascribed to C–H stretching of alkyl groups, C
O stretching of amide I, N–H stretching of amide II and N–H stretching of amide III, respectively.41 For CMC, the characteristic absorption bands were observed at 3285, 1585, 1409, 1321 and 1054 cm−1 corresponding to the O–H stretching vibration, N–H stretching vibration of amide II, symmetric stretching vibration of COO− groups, C–H stretching vibration and C–O asymmetric stretching vibration, respectively.15,42 Additionally, the distinctive absorption band located at 992 cm−1 in the spectrum of Lap was assigned to the Si–O stretching vibration.43 Compared to the spectrum of gelatin samples, the peak at 1403 cm−1 was sharper and the peak intensity at 1077 cm−1 was enhanced in the spectrum of Lap-incorporated GC scaffold, which was due to the blending of CMC within the composite. Furthermore, a strong band at 1013 cm−1 was appeared, confirming the incorporation of Lap into the composite.
 |
| Fig. 2 FTIR spectra of: (a) LAPONITE®, (b) CMC, (c) gelatin, (d) GC-Lap scaffold. | |
3.3 Mechanical testing
The mechanical strength of scaffolds determines how effectively it can withstand the mechanical load from new tissue growing into the gradually degrading scaffold matrix.44 Mechanical properties are also important for cell adhesion, proliferation, and differentiation.23 We therefore evaluated the mechanical properties of GC and GC-Lap composite scaffolds in a dry state. As displayed in Fig. 3, both the compressive strength and Young's modulus enhanced with the increased Lap concentration: the compressive strength and Young's modulus of the GC scaffold were 0.72 ± 0.16 MPa and 12.15 ± 1.29 MPa, while the compressive strength and Young's modulus for GC-Lap5%, GC-Lap10% and GC-Lap15% were 0.80 ± 0.02 MPa and 14.50 ± 1.17 MPa, 0.77 ± 0.02 MPa and 16.25 ± 0.80 MPa, 1.01 ± 0.02 MPa and 18.5 ± 0.82 MPa, respectively. This increase in mechanical strength was likely due to the increased density of crosslinks (electrostatic interaction and hydrogen bonds interaction as LAPONITE® worked as noncovalent cross-linkers) between the polymer and Lap and consequent decrease in pore diameter, which yielded a structure that can better withstand stress.30,45 Similar results were reported in other studies where adding silicate nanoparticles to polymer significantly improved the mechanical strength of the nanocomposite films.46,47 It should be noted that despite the compressive strength of the prepared scaffolds didn't reach the standard of cancellous bone (2–20 MPa),48,49 the fabricated GC-Lap scaffolds can still be applied in non-load bearing bone defect repair. Moreover, once implanted in vivo, the compressive strength of the porous scaffolds increased with the ingrowth of new bone tissue.
 |
| Fig. 3 Mechanical properties of four different scaffolds: (A) strain–stress curves and (B) compressive modulus. *p < 0.05 compared with the GC. **p < 0.01 compared with the GC. | |
3.4 Swelling properties of the scaffold
Swelling capacity is an important property of scaffolds as it affects the exchange of cell nutrients and metabolites. It also increases pore diameter and total porosity, thus providing much larger internal surface area of scaffolds for cell in-growth and attachment. However, swelling under physiological conditions must be controlled, as it could cause weakening and rapid degradation of the bone scaffold.38 The degree of swelling of the prepared scaffolds was directly proportional to Lap concentration, as scaffolds containing larger amounts of silicate had a higher degree of hydration (Fig. 4). The point of saturated swelling differed among the prepared scaffolds. The sizes of pure GC, GC-Lap5%, GC-Lap10%, and GC-Lap15% scaffolds increased by 449%, 372%, 332%, and 224%, respectively, after immersion in phosphate-buffered saline (PBS) for 10 min. The swelling ratio increased with immersion time: after 60 min, the for pure GC, GC-Lap5%, GC-Lap10%, and GC-Lap15% scaffolds were 1120%, 892%, 771%, and 647%, respectively, of their original sizes. At this point, the scaffolds were saturated, as no further swelling was noted with longer incubation times. This is similar to the findings of other studies that the presence of Lap or other nanocomposites in a polymer scaffold decreased the swelling ratio.50 This may be due to ionic interactions between silicate and polymer as well as surrounding water molecules that promote the structural stability of the nanocomposite network upon immersion in PBS. The favorable hydrophilic properties of GC-Lap scaffolds facilitate the diffusion of nutrients and metabolites into newly grown tissue.45
 |
| Fig. 4 Swelling behaviour of four different scaffolds as a function of time. | |
3.5 In vitro biodegradation
Scaffold materials should be biodegradable and bioresorbable at an appropriate rate to match the speed of new bone tissue formation. The biodegradation rate that matches the new bone tissue regeneration rate is an important aspect of scaffold design.51 We therefore evaluated the degradation of pure GC and GC-Lap scaffolds immersed in PBS solution with lysozyme addition. The degradation rate of composite scaffold decreased as the added Lap increased (Fig. 5): the rates were 54%, 45%, and 26% for GC-Lap5%, GC-Lap10%, and GC-Lap15% scaffolds, respectively, after incubation for 21 days in lysozyme. However, the pure GC scaffold showed considerable degradation, with only 38% remaining on day 21. This is likely due to increased hydrogen bonding and ionic interaction between Lap and water molecules and decreased interaction between polymeric macromolecules and water.24 Given that Lap crosslinks enhance the integrity of the GC network; thus, the degradation rate of the scaffold can be regulated by modifying Lap concentration. The slow degradation rate of the scaffold can also provide adequate time for tissue regeneration.45
 |
| Fig. 5 In vitro biodegradation of porous scaffolds: GC, GC-Lap5%, GC-Lap10% and GC-Lap15%. | |
3.6 In vitro biocompatibility
Rat bone marrow-derived mesenchymal cells (rBMSCs) are one of the most frequently used cells for investigating tissue repair and reconstruction owing to its multipotency and capacity for self-renewal.52 The in vitro biocompatibility of GC-Lap scaffolds was investigated using rBMSCs (passage 3). The proliferation of cells co-cultured with various scaffolds was assessed with the 3-[4,5-dimethylthiazol-2-yl]-2,5-diphenyltetrazolium bromide (MTT) assay. Cell proliferation rates of cells grown on pure GC, GC-Lap5%, and GC-Lap10% scaffolds increased with time from 1 to 7 days (Fig. 6). The Lap-incorporated scaffolds stimulated cell growth to a greater extent than pure GC, with GC-Lap10% being more effective than GC-Lap5%, especially after 3 days incubation (P < 0.05). This may be due to the suitable concentration of dissolution products of Lap [Na+, orthosilicic acid (Si(OH)4), Mg2+, and Li+], which are similar to those of bioactive glass, and are known to promote cell proliferation.53,54 In contrast, in the presence of GC-Lap15%, cell proliferation was inhibited, possibly due to the adverse effects of high concentrations of Lap. In our study, the optimal Lap concentration for rBMSC proliferation was 10%. A previous study reported that the optimum concentration of cloisite clay in poly[ethylene-co-(vinyl acetate)]/cloisite scaffolds for human dermal fibroblast proliferation was 10%, with cell growth inhibited at higher concentrations.21 Other studies have found that the ideal Lap concentration for osteogenesis is 100 μg ml−1, above which cell proliferation is suppressed.28,29
 |
| Fig. 6 The proliferation of rBMSCs after 1, 3, and 7 days of culture on the different scaffolds determined by MTT assay. *p < 0.05 compared with the GC. **p < 0.01 compared with the GC. ***p < 0.001 compared with the GC. | |
3.7 Cell attachment to scaffolds
A major consideration in fabricating a scaffold for BTE is that it should support cell adhesion and spreading, since these play a critical role in promoting cell proliferation, differentiation, and formation of the ECM.19 To assess the potential of Lap incorporated nanocomposites for BTE applications, the attachment and morphology of rBMSCs on the scaffolds were evaluated by SEM (Fig. 7) and confocal microscopy (Fig. 8). As demonstrated by SEM (Fig. 7), cells on the GC, GC-Lap5%, and GC-Lap10% scaffolds had filopodial extensions that increased in number which consistent with Lap concentration after 12 hours of incubation. In contrast, cells on the GC-Lap15% scaffold were relatively round and extended few filopodia, suggesting that adhesion and spreading were inhibited. The confocal analysis (Fig. 8) revealed a large number of uniformly distributed cells on GC-Lap5%, and GC-Lap10% scaffold surfaces after 5 days of incubation. However, there were fewer cells on the GC and GC-Lap15% scaffolds. The greater adhesion and spreading in the GC-Lap5% and GC-Lap10% groups relative to the GC group may be explained by the existence of a magnetic field generated by adsorption of iron onto Lap.21 Additionally, divalent cations such as Mg2+ (a degradation product of Lap) play an important role in the adhesion of cells to biomaterial surfaces via interactions with integrin family proteins.55,56 Thus, GC-Lap scaffolds may stimulate rBMSC spreading and adhesion and consequently promote their proliferation. This is in agreement with earlier findings that nanoparticle-containing scaffolds23,56 enhanced cell adhesion, which in turn stimulated proliferation. Since GC-Lap15% inhibited rBMSC proliferation, adhesion, and spreading, it was excluded from subsequent analyses.
 |
| Fig. 7 SEM micrographs of rBMSCs growing on pure GC, GC-Lap5%, GC-Lap10% and GC-Lap15% scaffolds, after 12 h of culture. | |
 |
| Fig. 8 Confocal images of rBMSCs on pure GC, GC-Lap5%, GC-Lap10% and GC-Lap15% after 3 days of culture. | |
3.8 ALP activity
Bioactive materials influence cell behavior by releasing soluble components. At pH values between 7.3 and 8.4, Lap degrades into non-toxic products [Na+, Si(OH)4, Mg2+, Li+].57 These dissolution products have been reported to promote collagen type I synthesis and osteoblastic differentiation in vitro.58 Based on these observations, we speculated that freeze-dried GC scaffold containing Lap would promote osteogenic differentiation of rBMSCs by providing ionic dissolution products. The osteogenic differentiation potential of passage 3 rBMSCs was evaluated by monitoring ALP activity over a period of 21 days. ALP activity is an early osteogenic differentiation marker that initially increases before decreasing as mineralization of the ECM proceeds.24 A greater intensity of ALP staining was observed in rBMSCs grown on GC-Lap10% as compared to the GC-Lap5% and pure GC scaffolds after 7 days of culture (Fig. 9A). The intensity of ALP staining in GC-Lap5% and GC-Lap10% further increased on day 14 and was most apparent in GC-Lap10%. A quantitative analysis of ALP activity on days 7, 14, and 21 revealed an increase on days 7 and 14 in Lap-incorporated scaffolds as compared to pure GC scaffold, although a decrease was observed on day 21 (Fig. 9B). In contrast, the GC group showed delayed and moderate activity that peaked on day 14 before decreasing. These results indicate that Lap in polymeric scaffolds induces and promotes osteogenic differentiation of rBMSCs.
 |
| Fig. 9 The osteogenic differentiation of rBMSCs on four different scaffolds. (A) ALP staining at day 7 and day 14. (B) ALP quantification at day 7, day 14 and day 21. *p < 0.05 compared with the GC. **p < 0.01 compared with the GC. ***p < 0.001 compared with the GC. | |
3.9 Osteogenic marker expression in rBMSCs grown on scaffolds
To further investigate the osteogenic differentiation of rBMSCs induced by GC-Lap scaffolds, we examined the expression of Runt-related transcription factor 2 (Runx-2), osteocalcin (OCN), osteopontin (OPN), and ALP in rBMSCs grown on the scaffolds in osteogenic medium by quantitative real-time PCR (qRT-PCR). Runx-2 is an early marker of osteoblast differentiation that regulates the expression of osteoblast-specific genes such as ALP, collagen-I, and OCN.59 The presence of Lap in the scaffolds resulted in higher Runx-2 expression relative to the pure GC scaffold on day 7, with the highest levels observed in cells grown on GC-Lap10% (Fig. 10A). OCN, as a non-collagenous protein produced by osteoblasts that signals the termination of osteogenic differentiation, is used to assess bone cell lineage and new bone formation.60 There was no significant difference in OCN expression between pure GC and GC-Lap on day 7, but the level was higher in cells cultured on GC-Lap than on the GC scaffold on day 14, especially true for the GC-Lap10% group. OPN, another late marker of osteoblast differentiation, showed the same trend as OCN, with maximal expression observed in cells grown on GC-Lap10% on day 14. As an early differentiation marker, ALP plays a major role in the conversion of organic pyrophosphate to inorganic phosphate.61 As showed in Fig. 10D, ALP was significantly upregulated in cells cultured on the both Lap-containing scaffolds on day 7 relative to the GC group. A similar trend was observed on day 14, especially for cells grown on GC-Lap10%. Thus, Lap-containing nanocomposites can effectively promote the osteogenic differentiation of rBMSCs. These results are in agreement with previous studies demonstrating that adding bioactive nanoparticles (silica and hydroxyapatite) to polymeric matrix increased the expression levels of osteogenic differentiation genes.62,63
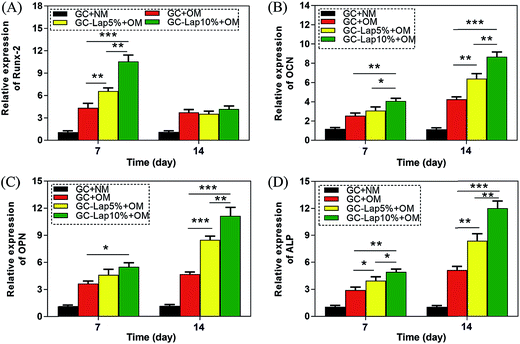 |
| Fig. 10 mRNA expression of osteoblast differentiation marker genes on four different scaffolds (A: Runx-2; B: OCN; C: OPN; D: ALP) at day 7 and day 14. *p < 0.05 compared with the GC. **p < 0.01 compared with the GC. ***p < 0.001 compared with the GC. | |
3.10 Induction of osteogenesis in vivo by GC-Lap scaffolds
The osteogenesis-inducing capacity of Lap-enriched polymers has not been investigated in vivo. We addressed this by implanting an rBMSC-loaded GC-Lap10% scaffold into a bilateral 5 mm calvarial bone defects in rats (Fig. 11), with GC and GC-Lap5% scaffolds serving as controls. Osteogenesis was evaluated by micro-computed tomography (micro-CT) and histology was analyzed by van Gieson's picrofuchsin staining. At 4 weeks after implantation, micro-CT analysis revealed no evidence of new bone formation in the GC group and only a small area of new bone in the GC-Lap5% group. In contrast, bone regeneration was clearly observed in the GC-Lap10% group (Fig. 12). The difference between groups was more pronounced at 8 weeks: the new bone volume to tissue volume ratio (BV/TV) was higher in the GC-Lap10% group (4 weeks: 10.87% ± 2.20%; 8 weeks: 34.80% ± 2.53%) than in the GC (4 weeks: 2.03% ± 0.76%; 8 weeks: 4.60% ± 1.50%) and GC-Lap5% (4 weeks: 5.70% ± 1.38% and 8 weeks: 18.64% ± 2.18%) groups. Interestingly, in Lap-incorporated scaffolds, the new bone generated not only along the defect margin, but also in the central part of the calvarial bone defect. A plausible explanation is chemoattraction by silicate, which is known to promote early angiogenesis, enhance BMSC migration, and promote bone regeneration in critical-sized calvarial defects in mice.64
 |
| Fig. 11 The scaffolds cut into sections 5 mm in diameter and 1 mm thick (A and B), and implanted in bilateral 5 mm critical-sized calvarial defects (C and D) in rats. | |
 |
| Fig. 12 Virtual micro-CT images from rats receiving the pure GC, GC-Lap5%, and GC-Lap10% scaffolds at 4 and 8 weeks after implantation. | |
A light microscopic examination of van Gieson's picrofuchsin staining of newly formed bone at 8 weeks further supported the results of the micro-CT analysis (Fig. 13). The percentages of newly formed bone area at 8 week was higher in the defect of GC-Lap10% (28.05 ± 2.01%) than that of GC-Lap5% (16.32 ± 1.26%) and GC (2.04 ± 0.68%). There was no evidence of new bone deposition in the GC scaffold group except at the border between the defect area and original bone, while in GC-Lap5% group, only a small quantity of red-stained bone tissue was distributed in the defect area.
 |
| Fig. 13 VG staining of cranial bone for the pure GC, GC-Lap5%, and GC-Lap10% scaffolds at 8 weeks after implantation. | |
4. Conclusions
A novel composite scaffold was fabricated by incorporating Lap nanoclay into a gelatin/CMC matrix by freeze drying. Lap incorporation increased the mechanical properties of GC scaffolds while decreasing pore size, swelling, and degradation. A scaffold with 10 wt% Lap was ideal for promoting rBMSC attachment, proliferation, and osteogenic differentiation. The in vivo experiments revealed a gradual growth of bone tissue into the scaffolds. These results indicate that GC-Lap10% scaffolds are a suitable material for BTE applications.
Conflicts of interest
There are no conflicts to declare.
Acknowledgements
The project was supported by the National Natural Science Foundation of China (Grant No. 81171707 and Grant No. 81572156), the National High Technology Research and Development Program of China (863 Program: 2015AA020308).
References
- C. T. Laurencin, A. M. Ambrosio, M. D. Borden and J. A. Cooper Jr, Annu. Rev. Biomed. Eng., 1999, 1, 19 CrossRef CAS PubMed.
- K. J. Burg, S. Porter and J. F. Kellam, Biomaterials, 2000, 21, 2347 CrossRef CAS PubMed.
- R. Langer and J. P. Vacanti, Science, 1993, 260, 920 CAS.
- T. Kokubo, H. M. Kim and M. Kawashita, Biomaterials, 2003, 24, 2161 CrossRef CAS PubMed.
- J. S. Mao, L. G. Zhao, Y. J. Yin and K. D. Yao, Biomaterials, 2003, 24, 1067 CrossRef CAS PubMed.
- A. Nandakumar, C. Cruz, A. Mentink, Z. Tahmasebi Birgani, L. Moroni, C. van Blitterswijk and P. Habibovic, Acta Biomater., 2013, 9, 5708 CrossRef CAS PubMed.
- J. Venkatesan, P. A. Vinodhini, P. N. Sudha and S. K. Kim, Adv. Food Nutr. Res., 2014, 73, 59 CAS.
- S. Hammouche, D. Hammouche and M. McNicholas, Curr. Stem Cell Res. Ther., 2012, 7, 134 CrossRef CAS PubMed.
- R. Yunus Basha, T. S. Sampath Kumar and M. Doble, Mater. Sci. Eng., C, 2015, 57, 452 CrossRef CAS PubMed.
- H. Ehrlich, E. Steck, M. Ilan, M. Maldonado, G. Muricy, G. Bavestrello, Z. Kljajic, J. L. Carballo, S. Schiaparelli, A. Ereskovsky, P. Schupp, R. Born, H. Worch, V. V. Bazhenov, D. Kurek, V. Varlamov, D. Vyalikh, K. Kummer, V. V. Sivkov, S. L. Molodtsov, H. Meissner, G. Richter, S. Hunoldt, M. Kammer, S. Paasch, V. Krasokhin, G. Patzke, E. Brunner and W. Richter, Int. J. Biol. Macromol., 2010, 47, 141 CrossRef CAS PubMed.
- E. Landi, F. Valentini and A. Tampieri, Acta Biomater., 2008, 4, 1620 CrossRef CAS PubMed.
- V. K. Nandagiri, P. Gentile, V. Chiono, C. Tonda-Turo, A. Matsiko, Z. Ramtoola, F. M. Montevecchi and G. Ciardelli, J. Mech. Behav. Biomed. Mater., 2011, 4, 1318 CrossRef CAS PubMed.
- J. Li, Y. Chen, Y. Yin, F. Yao and K. Yao, Biomaterials, 2007, 28, 781 CrossRef CAS PubMed.
- Q. Xing, K. Yates, C. Vogt, Z. Qian, M. C. Frost and F. Zhao, Sci. Rep., 2014, 16, 4706 Search PubMed.
- D. Mishra, B. Bhunia, I. Banerjee, P. Datta, S. Dhara and T. K. Maiti, Mater. Sci. Eng., C, 2011, 31, 1295 CrossRef CAS.
- L. Upadhyaya, J. Singh, V. Agarwal and R. P. Tewari, J. Controlled Release, 2014, 186, 54 CrossRef CAS PubMed.
- L. Upadhyaya, J. Singh, V. Agarwal and R. P. Tewari, Carbohydr. Polym., 2013, 91, 452 CrossRef CAS PubMed.
- P. J. Schexnailder, A. K. Gaharwar, R. L. Bartlett 2nd, B. L. Seal and G. Schmidt, Macromol. Biosci., 2010, 10, 1416 CrossRef CAS PubMed.
- A. K. Gaharwar, V. Kishore, C. Rivera, W. Bullock, C. J. Wu, O. Akkus and G. Schmidt, Macromol. Biosci., 2012, 12, 779 CrossRef CAS PubMed.
- G. Ozkoc, S. Kemaloglu and M. Quaedflieg, Polym. Compos., 2010, 31, 674 CAS.
- H. M. Lewkowitz-Shpuntoff, M. C. Wen, A. Singh, N. Brenner, R. Gambino, N. Pernodet, R. Isseroff, M. Rafailovich and J. Sokolov, Biomaterials, 2009, 30, 8 CrossRef CAS PubMed.
- G. Nitya, G. T. Nair, U. Mony, K. P. Chennazhi and S. V. Nair, J. Mater. Sci.: Mater. Med., 2012, 23, 1749 CrossRef CAS PubMed.
- A. K. Gaharwar, P. J. Schexnailder, B. P. Kline and G. Schmidt, Acta Biomater., 2011, 7, 568 CrossRef CAS PubMed.
- A. K. Gaharwar, S. Mukundan, E. Karaca, A. Dolatshahi-Pirouz, A. Patel, K. Rangarajan, S. M. Mihaila, G. Iviglia, H. Zhang and A. Khademhosseini, Tissue Eng., Part A, 2014, 20, 2088 CrossRef CAS PubMed.
- C. Wang, S. Wang, K. Li, Y. Ju, J. Li, Y. Zhang, J. Li, X. Liu, X. Shi and Q. Zhao, PLoS One, 2014, 23, e99585 Search PubMed.
- A. H. Ambre, D. R. Katti and K. S. Katti, J. Biomed. Mater. Res., Part A, 2013, 101, 26 Search PubMed.
- H. Zhuang, J. P. Zheng, H. Gao and K. De Yao, J. Mater. Sci.: Mater. Med., 2007, 18, 951 CrossRef CAS PubMed.
- A. K. Gaharwar, S. M. Mihaila, A. Swami, A. Patel, S. Sant, R. L. Reis, A. P. Marques, M. E. Gomes and A. Khademhosseini, Adv. Mater., 2013, 25, 3329 CrossRef CAS PubMed.
- S. M. Mihaila, A. K. Gaharwar, R. L. Reis, A. Khademhosseini, A. P. Marques and M. E. Gomes, Biomaterials, 2014, 35, 9087 CrossRef CAS PubMed.
- D. Su, L. Jiang, X. Chen, J. Dong and Z. Shao, ACS Appl. Mater. Interfaces, 2016, 8, 9619–9628 CAS.
- S. J. Hollister, Nat. Mater., 2005, 4, 518–524 CrossRef CAS PubMed.
- J. Xie, C. Peng, Q. Zhao, X. Wang, H. Yuan, L. Yang, K. Li, X. Lou and Y. Zhang, Acta Biomater., 2016, 29, 365 CrossRef CAS PubMed.
- K. J. Livak and T. D. Schmittgen, Methods, 2001, 25, 402 CrossRef CAS PubMed.
- D. Zou, Z. Zhang, D. Ye, A. Tang, L. Deng, W. Han, J. Zhao, S. Wang, W. Zhang, C. Zhu, J. Zhou, J. He, Y. Wang, F. Xu, Y. Huang and X. Jiang, Stem Cells, 2011, 29, 1380 CAS.
- Y. Takahashi and Y. Tabata, J. Biomater. Sci., Polym. Ed., 2004, 15, 41–57 CrossRef CAS PubMed.
- G. Akay, M. Birch and M. Bokhari, Biomaterials, 2004, 25, 3991 CrossRef CAS PubMed.
- X. Liu and P. X. Ma, Ann. Biomed. Eng., 2004, 32, 477 CrossRef PubMed.
- K. Maji, S. Dasgupta, K. Pramanik and A. Bissoyi, Int. J. Biomater., 2016, 2016, 9825659 Search PubMed.
- S. Dhivya, S. Saravanan, T. P. Sastry and N. Selvamurugan, J. Nanobiotechnol., 2015, 13, 40 CrossRef CAS PubMed.
- J. Huang, S. M. Best, W. Bonfield, R. A. Brooks, N. Rushton, S. N. Jayasinghe and M. J. Edirisinghe, J. Mater. Sci.: Mater. Med., 2004, 15, 441 CrossRef CAS PubMed.
- S. Kim, M. E. Nimni, Z. Yang and B. Han, J. Biomed. Mater. Res., Part B, 2005, 75, 442 CrossRef PubMed.
- C. Yang, L. Xu, Y. Zhou, X. M. Zhang, X. Huang, M. Wang, Y. Han, M. L. Zhai, S. C. Wei and J. Q. Li, Carbohydr. Polym., 2010, 82, 1297 CrossRef CAS.
- L. Ding, Y. Hu, Y. Luo, J. Zhu, Y. Wu, Z. Yu, X. Cao, C. Peng, X. Shi and R. Guo, Biomater. Sci., 2016, 4, 474 RSC.
- K. C. Kavya, R. Jayakumar, S. Nair and K. P. Chennazhi, Int. J. Biol. Macromol., 2013, 59, 255 CrossRef CAS PubMed.
- C. Sharma, A. K. Dinda, P. D. Potdar, C. F. Chou and N. C. Mishra, Mater. Sci. Eng., C, 2016, 64, 416 CrossRef CAS PubMed.
- G. F. Perotti, H. S. Barud, Y. Messaddeq, S. J. L. Ribeiro and V. R. L. Constantino, Polymer, 2011, 52, 157 CrossRef CAS.
- C.-H. Lee, M. Kato and A. Usuki, J. Mater. Chem., 2011, 21, 6844 RSC.
- S. Bose, M. Roy and A. Bandyopadhyay, Trends Biotechnol., 2012, 30, 546–554 CrossRef CAS PubMed.
- J. Ran, H. Zeng, J. L. Pathak, P. Jiang, Y. Bai, P. Yan, G. Sun, X. Shen, H. Tong and B. Shi, Biomacromolecules, 2017, 18, 3788–3801 CrossRef CAS PubMed.
- N. Pramanik, D. Mishra, I. Banerjee, T. Kumar Maiti, P. Bhargava and P. Pramanik, Int. J. Biomater., 2009, 512417 Search PubMed.
- G. Orive, R. M. Hernández, A. R. Gascón, M. Igartua and J. L. Pedraz, Int. J. Pharm., 2003, 259, 57 CrossRef CAS PubMed.
- S. Agacayak, B. Gulsun, M. C. Ucan, E. Karaoz and Y. Nergiz, Eur. Rev. Med. Pharmacol. Sci., 2012, 16, 679–686 CAS.
- T. Gao, H. T. Aro, H. Ylänen and E. Vuorio, Biomaterials, 2001, 22, 1475 CrossRef CAS PubMed.
- I. D. Xynos, A. J. Edgar, L. D. K. Buttery, L. L. Hench and J. M. Polak, J. Biomed. Mater. Res., 2001, 55, 151 CrossRef CAS PubMed.
- H. Zreiqat, C. R. Howlett, A. Zannettino, P. Evans, G. Schulze-Tanzil, C. Knabe and M. Shakibaei, J. Biomed. Mater. Res., 2002, 62, 175 CrossRef CAS PubMed.
- A. K. Gaharwar, C. Rivera, C.-J. Wu, B. K. Chan and G. Schmidt, Mater. Sci. Eng., C, 2013, 33, 1800 CrossRef CAS PubMed.
- D. W. Thompson and J. T. Butterworth, J. Colloid Interface Sci., 1992, 151, 236 CrossRef CAS.
- D. M. Reffitt, N. Ogston, R. Jugdaohsingh, H. F. J. Cheung, B. A. J. Evans, R. P. H. Thompson, J. J. Powell and G. N. Hampson, Bone, 2003, 32, 127 CrossRef CAS PubMed.
- H. Harada, S. Tagashira, M. Fujiwara, S. Ogawa, T. Katsumata, A. Yamaguchi, T. Komori and M. Nakatsuka, J. Biol. Chem., 1999, 274, 6972 CrossRef CAS PubMed.
- J. M. Oliveira, M. T. Rodrigues, S. S. Silva, P. B. Malafaya, M. E. Gomes, C. A. Viegas, I. R. Dias, J. T. Azevedo, J. F. Mano and R. L. Reis, Biomaterials, 2006, 27, 6123 CrossRef CAS PubMed.
- C. G. Bellows, J. E. Aubin and J. N. Heersche, Bone Miner., 1991, 14, 27 CrossRef CAS.
- A. J. Mieszawska, N. Fourligas, I. Georgakoudi, N. M. Ouhib, D. J. Belton, C. C. Perry and D. L. Kaplan, Biomaterials, 2010, 31, 8902 CrossRef CAS PubMed.
- J. H. Lee, N. G. Rim, H. S. Jung and H. Shin, Macromol. Biosci., 2010, 10, 173 CrossRef CAS PubMed.
- M. A. Saghiri, A. Asatourian, J. Orangi, C. M. Sorenson and N. Sheibani, Crit. Rev. Oncol. Hematol., 2015, 96, 143 CrossRef PubMed.
Footnote |
† These authors contributed equally to this work. |
|
This journal is © The Royal Society of Chemistry 2017 |
Click here to see how this site uses Cookies. View our privacy policy here.