DOI:
10.1039/C7RA04885H
(Paper)
RSC Adv., 2017,
7, 32461-32467
Introducing Ti3+ defects based on lattice distortion for enhanced visible light photoreactivity in TiO2 microspheres†
Received
1st May 2017
, Accepted 8th June 2017
First published on 26th June 2017
Abstract
Defective titanium dioxide (TiO2) is of much significance due to its improved visible light photoreactivity. Generally, the existence of defects leads to imperfections in the crystal lattice, which in turn affect the dynamics of the evolution of defects and the corresponding physical properties of TiO2. Until now, how lattice distortion affects the formation of Ti3+ defects as well as the corresponding visible light photoreactivity in TiO2 has remained elusive. Herein, we have successfully introduced Ti3+ defects based on lattice distortion in TiO2 microspheres and found the photocurrent of anatase TiO2 has been significantly enhanced from 1.78 to 80 μA cm−2 with an increase in photocatalytic activity of almost three times under visible light irradiation. Furthermore, we show that lattice distortions have minimal contribution to enhancing the visible light photocatalytic activity because the band gap cannot be narrowed due to the absence of Ti3+ defects, yet the existence of lattice distortions could suppress the recombination of electron–hole pairs. Moreover, the formation of Ti3+ defects is energetically favored in lattice-distorted TiO2 compared to that of pristine TiO2. This work highlights the design and development of highly efficient TiO2 photocatalysts that operate under visible light irradiation.
Introduction
Titanium dioxide (TiO2) has attracted much attention in organic contaminant degradation since Carey et al.1 successfully degraded polychlorinated biphenyl using TiO2-based photocatalysts. Since then, enormous effort has been devoted to photocatalyst-based research, photovoltaics, and photo-electrochemical cells. However, the applications of TiO2 are greatly hindered by its low quantum efficiency in photocatalytic reactions, ineffective utilization of visible light due to the high recombination of photo-induced electron–hole pairs, and relatively large band gap (∼3.2 eV).2–4 To address these issues, researchers have used various strategies, such as fabricating composites,5–9 metal anion/cation or non-metal doping,10–15 surface sensitization by dyes,16–18 introducing defects19–28 and so on.
Among the above strategies, the introduction of defects, such as Ti3+, oxygen vacancies (OVs), and lattice disorder defects, has been widely studied due to their close relationship with the electronic structure, charge transport, and surface activity of TiO2.19–28 Chen et al.25 reported black TiO2 nanoparticles with significant lattice disorder and Ti3+ defects, exhibiting excellent solar-driven photocatalytic activities towards hydrogen generation. Grabstanowicz et al.27 have demonstrated that Ti3+-doped TiO2, accompanied with the formation of OVs, displays a broadened absorbance in the visible light region. These imperfections in the crystal lattice lead to lattice distortion, which further affect the corresponding photochemical properties.29–31 Still, our understanding with regards to (1) how lattice distortions affect the resulting visible light photoreactivity and (2) the formation of defects remains unclear.
Herein, we have successfully introduced defects based on lattice distortion in TiO2 microspheres. It is demonstrated that the enhancement of visible-light-driven photocatalysis is mainly attributed to the existence of Ti3+ defects, which leads to an elevated valence band edge and a narrower band gap, resulting in higher photoreactivity. The band gap of TiO2 with lattice distortions could not be narrowed, failing to improve visible light photocatalytic activity without Ti3+ defects, but the higher photocurrent generation indicates the migration of recombination of electron–holes pairs. Interestingly, lattice-distorted TiO2 favours the formation of Ti3+ defects. A combined effect of lattice distortion and defects was found to increase the photocatalytic activity nearly three times, and the photocurrent generation increased from 1.78 to 80 μA cm−2. Such progress is significant for the development of highly efficient photocatalysts and devices for solar fuel generation.
Experimental
Synthesis of TiO2-based materials
All chemicals were of analytical grade and used without further purification. In order to introduce lattice distortions to TiO2 microspheres, 0.05 mol Ti(SO4)2 and 0.05 mol NaCl were added to 60 mL of deionized water. After 30 min of stirring, the transparent solution was transferred into a high-pressure reactor and heated at 120 °C for 12 h with a heating rate of 5 °C min−1. Then, the resulting mass was cleaned, dried at 100 °C for 12 h, and calcined at 300 °C for 5 h, yielding a white powder. To prepare pure TiO2, no NaCl was added.
In a typical procedure of introducing defects, 10 mL ethylene glycol (EG) and 0.3 g of as-prepared TiO2 were added into a high-pressure reactor and were heated at 180 °C for 5 h with a heating rate of 5 °C min−1. The upper transparent solution was discarded and the solid mass was washed and dried at 100 °C for 12 h. The TG test (Fig. S7†) shows that the EG reduction does not leave organic remains in as-prepared samples.
TiO2 with both lattice distortion and defects was prepared by a combination of experimental procedures described above.
Characterization
X-ray diffraction (XRD) patterns were collected with a PANalytical Empyrean X-ray diffractometer with monochromatic Cu Kα radiation (λ = 1.5418 Å). X-ray photoelectron spectroscopy (XPS) was carried out on a PHI5300 (PerkinElmer) with a monochromatic Mg Kα source to analyze the surface chemistry. Scanning electron microscopy (SEM) images were carried out on a field emission scanning electron microscope (JEOL JSM-7001F; acceleration voltage = 10 kV). Transmission electron microscopy (TEM) images were obtained on a Zeiss LIBRA 200 FEG transmission electron microscope operating at 200 kV. UV-vis diffuse reflectance spectroscopy (DRS) was performed on a Shimadzu UV-2100 spectrophotometer using BaSO4 as the reference.
Photoelectrochemical characterization
The photoelectrochemical response was measured using a CHI 660B electrochemical workstation with conventional three-electrode setup under visible-light illumination. To fabricate the working electrode, 1 mg of as-prepared pure TiO2 and chlorine introduced TiO2 microspheres were coated onto a slice of ITO glass with an area of 1.5 × 1.5 cm2. A 0.5 × 0.5 cm2 Pt plate and a saturated calomel electrode were used as the counter reference electrodes, respectively. A 0.1 M Na2SO4 solution was used as the electrolyte and a 500 W Xe lamp was utilized as the visible light source.
Photocatalytic assessment
The photocatalytic activity of the as-prepared TiO2 microspheres was evaluated by photocatalytic degradation of a solution containing 20 mg L−1 rhodamine B (RhB) or 15 mg L−1 phenol irradiated with visible light (500 W Xe lamp and a UV cutoff filter (λ > 420 nm)). In a typical process, 70 mg of the as-prepared sample was added to 100 mL of RhB or phenol. Afterwards, the photocatalyst was dispersed in the solution and stirred for 0.5 h in the dark to reach adsorption equilibrium before being exposed to visible-light irradiation. The suspension was sampled every 20 min, and the photocatalyst was removed by centrifugation. The change in RhB or phenol concentration was determined relative to the extinction spectra for RhB by UV-vis spectroscopy.
Results and discussion
XRD patterns (Fig. 1a) show that all samples are composed of the pure anatase phase, which suggests that there was no significant change in the phase structure by the addition of chloride ions during the hydrothermal processing or reduction with ethylene glycol (EG). Nevertheless, it is noted that the (101) and (004) diffraction peaks slightly shift to smaller angles after adding chloride ions, while no obvious shift is observed after EG reduction, implying that the lattice parameters of TiO2 become larger and lattice distortion is introduced by adding chloride ions. It is further confirmed that the introduction of chlorine ions during the synthetic procedures brings about surface stress in the titania crystals, which results in lattice distortion. All samples are mainly composed of microspheres with average diameters of about 350 nm (Fig. 1b). Our results suggest that reduction with EG has no effect on the morphology, however, the existence of Cl ions during hydrothermal processing has a strong effect on the morphology of TiO2 (Fig. S1†). The presence of chloride ions leads to more hollow structures in the samples, indicating that the existence of chloride ions may play the role as the surface surfactant4,32,37 (Fig. S2†). TEM analysis further demonstrates that the hollow microspheres are constructed of numerous nanostructures (Fig. 1c). The lattice in area I matches the (101) crystallographic plane of anatase with d spacing of 0.36 nm, indicating that the exposed facets on the surface hold no significant changes (Fig. 1c). Nonetheless, evident lattice distortion exists in the TiO2 with the addition of chloride ions, as shown in Fig. 1c and S3,† which is consistent with the XRD results.
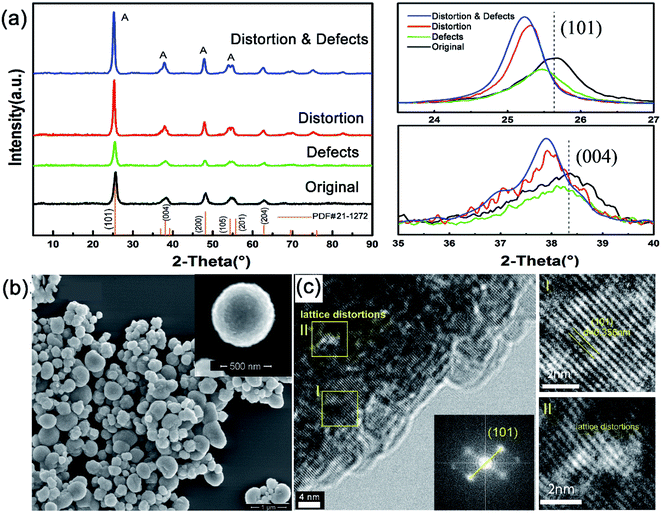 |
| Fig. 1 (a) XRD patterns of the as-prepared TiO2 samples (pristine TiO2 (Original), pure TiO2 with lattice with Ti3+ defects (Defects), pure TiO2 with lattice distortion (Distortion), TiO2 with both lattice distortion and defects (Distortion & Defects)). Patterns specially displaying the (101) and (004) peaks are shown to the right. (b) SEM images of the as-prepared TiO2 microspheres with both lattice distortion and defects. (c) HRTEM image of TiO2 with lattice distortion (inset: Fast Fourier Transformation (FFT) for area I) with enlarged HRTEM images of selected areas (I) and (II). | |
XPS analysis was performed to identify the existence of chloride ions and defects on the surface of the different TiO2 samples. In all samples, there was no signal for chlorine (Fig. S4†), suggesting that the existence of chloride ions in the hydrothermal processing only leads to lattice distortion in-stead of doping and can be removed with subsequent sample washing. Fig. 2 shows the Ti 2p peaks, where the 2p spin–orbital doublets two peaks center at ∼458.8 eV and ∼464.6 eV correspond to the characteristic Ti 2p3/2 and Ti 2p1/2 peaks of Ti4+, respectively.33,34 A detectable shoulder was observed at lower binding energies for the samples that contain defects, which can be ascribed to Ti3+ ions.35,36 More importantly, the peak area ratio of Ti3+/Ti4+ increases from 0.256
:
1 to 0.488
:
1 when lattice distortion is also present in TiO2. This increase in the Ti3+ concentration can be ascribed to the presence of lattice distortion, which allows for more accessible sites for Ti4+ to be reduced to Ti3+.
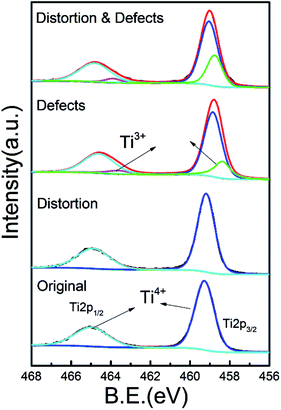 |
| Fig. 2 Ti 2p XPS spectrum of as-prepared TiO2. | |
The photocatalytic activity of the different TiO2 samples was evaluated by the degradation of rhodamine B (RhB) dye as well as phenol (Fig. S8†) under visible light irradiation (λ > 420 nm) (Fig. 3a). Only 37.8% RhB is degraded after 120 min by the original TiO2. When lattice distortion is present in the TiO2 microspheres, the degradation rate of RhB slightly decreases, indicating that lattice distortion due to the introduction of chloride ions during the preparation of TiO2 microspheres fails to enhance the photocatalytic activity of TiO2. After introducing Ti3+ defects, 47.9% RhB is degraded after 120 min using the same reaction conditions, whereas TiO2 with both distortion and defects achieved a degradation of more than 75%. The degradation rate increases dramatically from 38 × 10−4 to 95 × 10−4 s−1 from TiO2 with just defects to TiO2 with both distortion and defects, displaying that the activity is nearly three times higher when both lattice distortion and defects exist in TiO2 microspheres (Fig. S5†). The transient photocurrent response spectra (Fig. 3b) reveal that lattice distortion elevates the photocurrent from 1.78 to 15.56 μA cm−2 in the TiO2 microspheres, while Ti3+ defects elevate the photocurrent density to 53.78 μA cm−2. Even though the lattice distortion has no contribution on the improvement of visible light photocatalytic activity, the increase of photocurrent suggests that lattice distortion in TiO2 could efficiently supress the recombination of electron–hole pairs.38 The existence of both lattice distortion and Ti3+ defects elevates the photocurrent density to 80 μA cm−2. The larger the photocurrent value, the higher the charge separation efficiency. Thus, it can be concluded that the introduction of Ti3+ defects facilitates the separation of photoelectrons and holes, which is in accordance with the study of Cai et al.24 Considering the effect of surface area, as listed in Table S1,† the TiO2 with both defects and lattice distortion possess lower surface areas than the original TiO2, implying that the surface area is not the major effect on the improvement of visible light photoreactivity. It is thereby deduced that the enhancement in the photocurrent generation can be mainly attributed to the existence of Ti3+ defects. The introduction of lattice distortion could not only mitigate the recombination of photo-induced electron–hole pairs,39,45 but was also found to be advantageous for the generation of Ti3+ defects. These results demonstrate that the Ti3+ defects play a dominant role in improving the photoreactivity of TiO2 rather than lattice distortion, and a combined effect of lattice distortion and Ti3+ defects is beneficial for the enhancement of visible-light-driven photocatalytic activity and photocurrent generation.
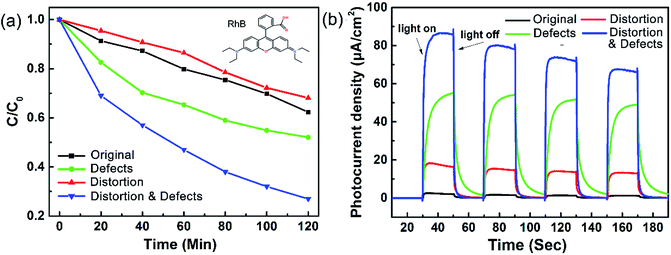 |
| Fig. 3 (a) The degradation of RhB and (b) photocurrent response of the different TiO2 samples under visible light irradiation. | |
A schematic is presented in Fig. 4 to illustrate the contribution of surficial lattice distortion to the formation of Ti3+ defects. Previous work reported that the effect of adding halogen ions during the sample preparation could tune the surface morphology due to the higher electronegativity of the halogen atoms,37–39 resulting in lattice expansion on the surface of TiO2. This increased bond length would energetically favour the generation of oxygen vacancies from TiO2 due to a lower bonding energy.40 Then the oxygen deficiencies transfers their extra two electrons to the adjacent two Ti4+ atoms to form Ti3+.41 Hence, Ti3+ defects appear and the proportion of these defects is increased for TiO2 with increased amounts of lattice distortion. Finally, the formation of Ti3+ defects for TiO2 with lattice distortion leads to the enhancement of photocurrent generation under visible light while also boosting the photocatalytic activity of TiO2.
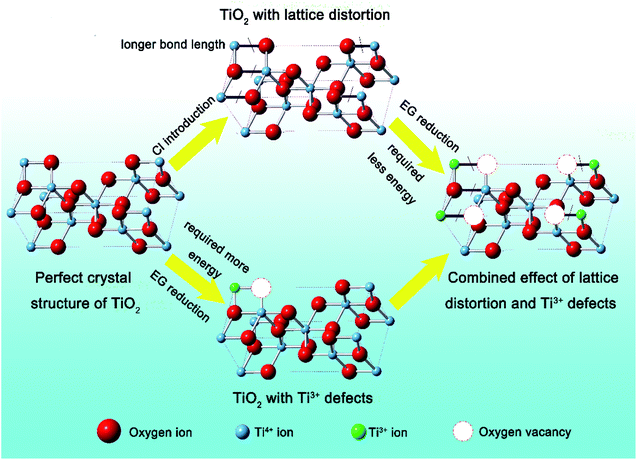 |
| Fig. 4 Schematic illustrating how lattice distortion contributes to the introduction of oxygen vacancies. | |
Fig. 5a displays the UV-vis diffuse reflectance spectra and the color of the different TiO2 samples. The color changes from white to yellow, and a noticeable difference in the absorption of visible light is observed when Ti3+ defects exist. Valence band (VB) XPS spectra (Fig. 5b) aided in determining the band position for the different TiO2 samples. The pure TiO2 shows a VB maximum energy of 3.10 eV below the Fermi level. After Ti3+ defects were introduced,43–45 an obvious upward shift by 0.36 eV occurs in the VB while lattice distortion only brings a slight upward shift by 0.14 eV. When both lattice distortion and defects exist, the VB top shifts to 2.64 eV with a 0.46 eV shift from the original TiO2. After introducing defects, a VB tail with a difference of 0.38 eV and 0.46 eV appears in TiO2 with and without lattice distortion, respectively. The corresponding band gaps were calculated42 to be 3.06 eV for the TiO2 with lattice distortion, similar to the band gap for the original TiO2 The band gap was found to be 2.98 eV after Ti3+ defects were introduced (Fig. S6†). It is thus deduced that the Ti3+ defects (rather than lattice distortion) lead to the extension of the visible light absorption. The VB edge of TiO2 with Ti3+ defects results in a narrower band gap and higher visible light activity when compared to pristine TiO2, which is in line with the results of Pan et al.28 A schematic illustration of the energy band structure for the different TiO2 samples is presented in (Fig. 5c). Due to the contribution of VB tails, the band gap is narrowed to 2.60 eV and further to 2.52 eV as the proportion of Ti3+ defects increases. Therefore, lattice distortion has little influence on the visible light photoreactivity of TiO2, while it benefits the migration and recombination of electron–hole pairs, and both Ti3+ defects and lattice distortion, in turn, greatly improve the visible light photoreactivity of TiO2.
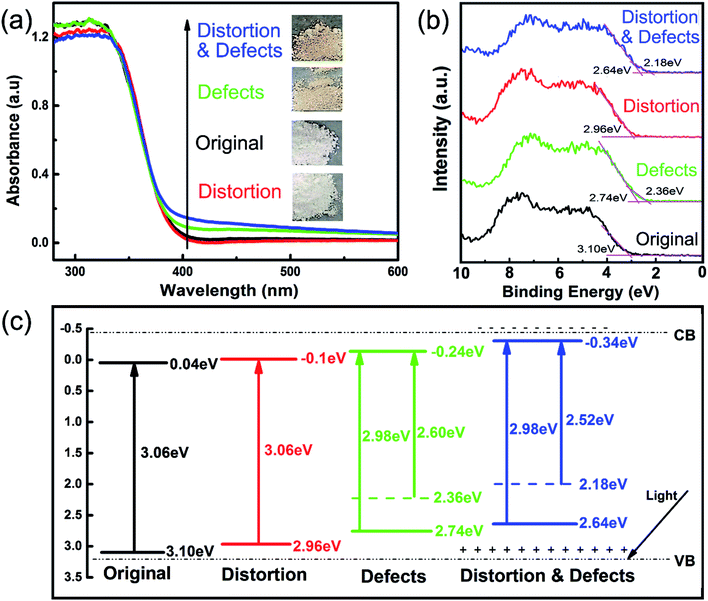 |
| Fig. 5 (a) UV-vis diffuse reflectance spectra. (b) Valence band XPS spectra of the different TiO2 samples. (c) Schematic illustration of the energy band structure for the different TiO2 samples. | |
Conclusions
In conclusion, lattice distortion was introduced to TiO2 microspheres by introducing chlorine during the hydrothermal processing while defects (Ti3+) were introduced by reduction with ethylene glycol. Our results demonstrate the relationship between lattice distortion and Ti3+ defects for anatase, which were strongly correlated with their photocatalytic properties. The existence of Ti3+ defects was found to strengthen the absorption of light in the UV and visible light regions and further elevates the VB position, leading to overall improved photocatalytic activity towards the degradation of RhB and phenol under visible light irradiation. Our results also demonstrate that defects and lattice distortion enhance the photocurrent generation of anatase TiO2 under visible light. Ti3+ defects are energetically favored over lattice distortion in TiO2 compared to that in pristine anatase TiO2. This study sheds light on the mechanism for engineering materials with lattice disorder and opens new opportunities for the design and synthesis of high-performance photocatalysts and solar fuel generation devices that operate under visible light irradiation.
Acknowledgements
This research is financially supported by the Fundamental Research Funds for the Central Universities (No. 106112015CDJXY130010) and the National Natural Science Foundation of China (No. 51302329). We gratefully acknowledge the theoretical calculations processed by Dr Wen Zeng. The preparation of this manuscript was also supported by the Center for Nanophase Materials Sciences, which is a DOE Office of Science User Facility. ZDH gratefully acknowledges a graduate fellowship from the National Science Foundation under Grant No. DGE-1148903 and the Georgia Tech-ORNL Fellowship.
Notes and references
- J. H. Carey, J. Lawrence and H. M. Tosine, Photodechlorination of PCB's in the Presence of Titanium Dioxide in Aqueous Suspensions, Bull. Environ. Contam. Toxicol., 1976, 16, 697–701 CrossRef CAS PubMed.
- X. Pan, M. Q. Yang and X. Fu, Defective TiO2 with Oxygen Vacancies: Synthesis, Properties and Photocatalytic Applications, Nanoscale, 2013, 5, 3601–3614 RSC.
- A. L. Linsebigler, G. Lu and J. T. Yates Jr, Photocatalysis on TiO2 Surfaces: Principles, Mechanisms, and Selected Results, Chem. Rev., 1995, 95, 735–758 CrossRef CAS.
- M. Kapilashrami, Y. Zhang and Y. S. Liu, et al., Probing the Optical Property and Electronic Structure of TiO2 Nanomaterials for Renewable Energy Applications, Chem. Rev., 2014, 114, 9662–9707 CrossRef CAS PubMed.
- W. J. Ong, L. L. Tan and S. P. Chai, Self-assembly of Nitrogen-doped TiO2 with Exposed {001} Facets on a Graphene Scaffold as Photo-active Hybrid Nanostructures for Reduction of Carbon Dioxide to Methane, Nano Res., 2014, 7, 1528–1547 CrossRef CAS.
- J. C. Wu, D. S. Liu and A. N. Ko, Dehydrogenation of Ethylbenzene over TiO2–Fe2O3 and ZrO2–Fe2O3 Mixed Oxide Catalysts, Catal. Lett., 1993, 20, 191–201 CrossRef CAS.
- K. Vinodgopal, I. Bedja and P. V. Kamat, Nanostructured Semiconductor Films for Photocatalysis. Photoelectrochemical Behavior of SnO2/TiO2 Composite Systems and its Role in Photocatalytic Degradation of a Textile Azo Dye, Chem. Mater., 1996, 8, 2180–2187 CrossRef CAS.
- R. Sasikala, A. Shirole and V. Sudarsan, et al., Highly Dispersed Phase of SnO2 on TiO2 Nanoparticles Synthesized by Polyol-mediated Route: Photocatalytic Activity for Hydrogen Generation, Int. J. Hydrogen Energy, 2009, 34, 3621–3630 CrossRef CAS.
- C. Yu, G. Li and S. Kumar, Stable Au25 (SR) 18/TiO2 Composite Nanostructure with Enhanced Visible Light Photocatalytic Activity, J. Phys. Chem. Lett., 2013, 4, 2847–2852 CrossRef CAS.
- H. Sun, S. Wang and H. M. Ang, et al., Halogen Element Modified Titanium Dioxide for Visible Light Photocatalysis., Chem. Eng. J., 2010, 162, 437–447 CrossRef CAS.
- X. Liu, P. Lv and G. Yao, Effection of Chlorine-doped TiO2 Photocatalysts for Photocatalytic Activity, Asian J. Chem., 2013, 25, 3275 CAS.
- R. Yuan, T. Chen and E. Fei, Surface Chlorination of TiO2-based Photocatalysts: A Way to Remarkably Improve Photocatalytic Activity in Both UV and Visible Region, ACS Catal., 2011, 1, 200–206 CrossRef CAS.
- S. Horikoshi, T. Miura and M. A. Kajitani, FT-IR (DRIFT) Study of the Influence of Halogen Substituents on the TiO2-assisted Photooxidation of Phenol and p-halophenols under Weak Room Light Irradiance, J. Photochem. Photobiol., A, 2008, 194, 189–199 CrossRef CAS.
- W. Choi, A. Termin and M. R. Hoffmann, The Role of Metal Ion Dopants in Quantum-sized TiO2: Correlation between Photoreactivity and Charge Carrier Recombination Dynamics, J. Phys. Chem., 1994, 98, 13669–13679 CrossRef.
- I. Y. Jeon, H. J. Choi and M. Choi, Facile, Scalable Synthesis of Edge-halogenated Graphene Nanoplatelets as Efficient Metal-free Electrocatalysts for Oxygen Reduction Reaction, Sci. Rep., 2013, 3, 1810 CrossRef PubMed.
- J. Wang and Z. Lin, Dye-sensitized TiO2 Nanotube Solar Cells with Markedly Enhanced Performance via Rational Surface Engineering, Chem. Mater., 2009, 22, 579–584 CrossRef.
- N. Guijarro, T. Lana-Villarreal and T. Lutz, et al., Sensitization of TiO2 with PbSe Quantum Dots by SILAR: How Mercaptophenol Improves Charge separation, J. Phys. Chem. Lett., 2012, 3, 3367–3372 CrossRef CAS.
- W. T. Sun, Y. Yu and H. Y. Pan, et al., CdS Quantum Dots Sensitized TiO2 Nanotube-array Photoelectrodes, J. Am. Chem. Soc., 2008, 130, 1124–1125 CrossRef CAS PubMed.
- F. Zuo, L. Wang and T. Wu, Self-doped Ti3+ Enhanced Photocatalyst for Hydrogen Production under Visible Light, J. Am. Chem. Soc., 2010, 132, 11856–11857 CrossRef CAS PubMed.
- J. Huo, Y. Hu and H. Jiang, In Situ Surface Hydrogenation Synthesis of Ti3+ Self-doped TiO2 with
Enhanced Visible Light Photoactivity, Nanoscale, 2014, 6, 9078–9084 RSC.
- S. Wang, L. Pan and J. J. Song, et al., Titanium-defected undoped anatase TiO2 with p-type conductivity, room-temperature ferromagnetism, and remarkable photocatalytic performance, J. Am. Chem. Soc., 2015, 137, 2975–2983 CrossRef CAS PubMed.
- Y. H. Hu, A Highly Efficient Photocatalyst—Hydrogenated Black TiO2 for the Photocatalytic Splitting of Water, Angew. Chem., Int. Ed., 2012, 51, 12410–12412 CrossRef CAS PubMed.
- L. Pan, S. Wang and J. Xie, et al., Constructing TiO2 pn homojunction for photoelectrochemical and photocatalytic hydrogen generation, Nano Energy, 2016, 28, 296–303 CrossRef CAS.
- J. Cai, K. Lv and J. Sun, et al., Ti powder-assisted synthesis of Ti3+ self-doped TiO2 nanosheets with enhanced visible-light photoactivity, RSC Adv., 2014, 4, 19588–19593 RSC.
- X. Chen, L. Liu and F. Huang, Black Titanium Dioxide (TiO2) Nanomaterials, Chem. Soc. Rev., 2015, 44, 1861–1885 RSC.
- A. L. Linsebigler, G. Lu and J. T. Yates Jr, Photocatalysis on TiO2 Surfaces: Principles, Mechanisms, and Selected Results, Chem. Rev., 1995, 95, 735–758 CrossRef CAS.
- L. R. Grabstanowicz, S. Gao and T. Li, et al., Facile oxidative conversion of TiH2 to high-concentration Ti3+-self-doped rutile TiO2 with visible-light photoactivity, Inorg. Chem., 2013, 52, 3884–3890 CrossRef CAS PubMed.
- L. Pan, S. Wang and J. J. Zou, et al., Ti3+-defected and V-doped TiO2 quantum dots loaded on MCM-41, Chem. Commun., 2014, 50, 988–990 RSC.
- D. Kim, J.-h. Yang and J. Hong, Ferromagnetism induced by Zn vacancy defect and lattice distortion in ZnO, J. Appl. Phys., 2009, 106, 013908 CrossRef.
- I. M. Lifshitz and A. M. Kosevich, The dynamics of a crystal lattice with defects, Rep. Prog. Phys., 1966, 29(1), 217–254 Search PubMed.
- M. Trau, N. Yao and E. Kim, et al., Microscopic patterning of orientated mesoscopic silica through guided growth, Nature, 1997, 390, 674–676 CAS.
- E. Andrews and S. M. Larson, Effect of surfactant layers on the size changes of aerosol particles as a function of relative humidity, Environ. Sci. Technol., 1993, 27, 857–865 CrossRef CAS.
- X. Chen, L. Liu and Y. Y. Peter, Increasing Solar Absorption for Photocatalysis with Black Hydrogenated Titanium Dioxide Nanocrystals, Science, 2011, 331, 746–750 CrossRef CAS PubMed.
- M. S. Lazarus and T. K. Sham, X-ray Photoelectron Spectroscopy (XPS) Studies of Hydrogen Reduced Rutile (TiO2−x) Surfaces, Chem. Phys. Lett., 1982, 92, 670–674 CrossRef CAS.
- G. Liu, H. G. Yang and X. Wang, Enhanced Photoactivity of Oxygen-deficient Anatase TiO2 Sheets with Dominant {001} Facets, J. Phys. Chem. C, 2009, 113, 21784–21788 CAS.
- C. Di Valentin, G. Pacchioni and A. Selloni, Reduced and n-type doped TiO2: nature of Ti3+ species, J. Phys. Chem. C, 2009, 113, 20543–20552 CAS.
- T. R. Gordon, M. Cargnello and T. Paik, Nonaqueous Synthesis of TiO2 Nanocrystals Using TiF4 to Engineer Morphology, Oxygen Vacancy Concentration, and Photocatalytic Activity, J. Am. Chem. Soc., 2012, 134, 6751–6761 CrossRef CAS PubMed.
- H. Tan, A. Jain and O. Voznyy, et al., Efficient and stable solution-processed planar perovskite solar cells via contact passivation, Science, 2017, 355, 722–726 CrossRef CAS PubMed.
- M. Lapertot, P. Pichat and S. Parra, Photocatalytic Degradation of p-halophenols in TiO2 Aqueous Suspensions: Halogen Effect on Removal Rate, Aromatic Intermediates and Toxicity Variations, J. Environ. Sci. Health, Part A: Environ. Sci. Eng., 2006, 41, 1009–1025 CrossRef CAS PubMed.
- L. Ye, L. Zan and L. Tian, et al., The {001} facets-dependent high photoactivity of BiOCl nanosheets, Chem. Commun., 2011, 47, 6951–6953 RSC.
- M. Batzill, E. H. Morales and U. Diebold, Surface studies of nitrogen
implanted TiO2, Chem. Phys., 2007, 339, 36–43 CrossRef CAS.
- M. A. Butler, Photoelectrolysis and physical properties of the semiconducting electrode WO2, J. Appl. Phys., 1977, 48, 1914 CrossRef CAS.
- S. Wang, L. Zhao and L. Bai, Enhancing photocatalytic activity of disorder-engineered C/TiO2 and TiO2 nanoparticles, J. Mater. Chem. A, 2014, 2, 7439–7445 CAS.
- A. Sinhamahapatra, J. P. Jeon and J. S. Yu, A New Approach to Prepare Highly Active and Stable Black Titania for Visible Light-assisted Hydrogen Production, Energy Environ. Sci., 2015, 8, 3539–3544 CAS.
- A. Naldoni, M. Allieta and S. Santangelo, Effect of Nature and Location of Defects on Bandgap Narrowing in Black TiO2 Nanoparticles, J. Am. Chem. Soc., 2012, 134, 7600–7603 CrossRef CAS PubMed.
Footnote |
† Electronic supplementary information (ESI) available. See DOI: 10.1039/c7ra04885h |
|
This journal is © The Royal Society of Chemistry 2017 |